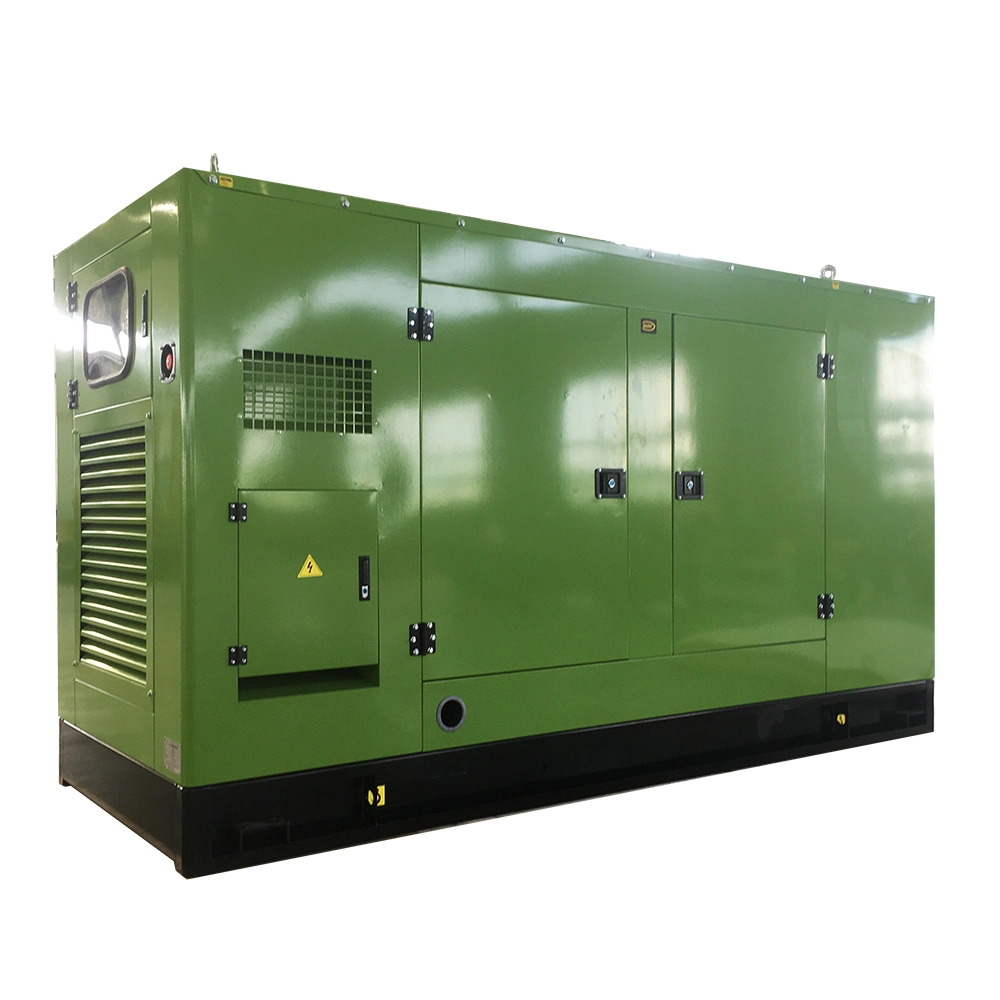
Gasifier: Gasification is a thermochemical conversion process that transforms carbon-containing feedstocks, such as biomass, coal, or waste materials, into a gaseous mixture known as syngas or synthesis gas. This process occurs under controlled conditions of temperature, pressure, and oxygen supply, typically in the absence of complete combustion. Gasification represents a versatile and efficient approach to convert diverse feedstocks into a valuable energy carrier with numerous applications.
The gasification process involves several key steps:
- Feedstock Preparation: The feedstock, which can range from wood chips and agricultural residues to coal and municipal solid waste, undergoes pretreatment to achieve the desired size, moisture content, and composition. This may involve drying, shredding, or pelletizing the feedstock to enhance its suitability for gasification.
- Gasification Reaction: The prepared feedstock is introduced into the gasification reactor, where it undergoes chemical reactions in the presence of a controlled amount of oxygen, steam, or a combination of both. These reactions typically include pyrolysis (thermal decomposition), combustion (partial oxidation), and gasification (conversion of carbon to syngas constituents).
- Syngas Production: The primary product of the gasification process is syngas, a mixture of hydrogen (H2), carbon monoxide (CO), carbon dioxide (CO2), methane (CH4), and other trace gases. The composition of syngas depends on factors such as the feedstock type, gasification temperature, residence time, and gasification agent.
- Tar and Particulate Removal: During gasification, tar compounds and particulate matter may be formed as byproducts of incomplete combustion and thermal decomposition. These impurities can foul equipment, reduce gas quality, and pose environmental and health risks. Therefore, syngas undergoes purification steps, such as tar cracking, filtration, or scrubbing, to remove these contaminants and improve syngas quality.
- Syngas Utilization: The produced syngas can be utilized in various applications, including power generation, biofuels production, chemical synthesis, and industrial processes. Depending on the specific requirements and desired end products, syngas may undergo further processing, such as catalytic conversion, reforming, or upgrading, to tailor its composition and properties for different applications.
Gasifier
Gasification offers several advantages compared to conventional combustion-based technologies:
- Fuel Flexibility: Gasification can utilize a wide range of feedstocks, including biomass, coal, municipal solid waste, and industrial residues, providing flexibility and resilience in energy supply.
- Energy Efficiency: Gasification processes can achieve high energy conversion efficiencies, especially when integrated with combined heat and power (CHP) systems, where waste heat is utilized for heating or industrial processes.
- Emissions Reduction: Gasification can produce syngas with lower emissions of greenhouse gases, particulates, and sulfur compounds compared to conventional combustion processes, contributing to environmental sustainability and air quality improvement.
- Resource Recovery: Gasification facilitates the recovery of energy and valuable byproducts from waste materials, promoting resource conservation and waste valorization.
Gasification technology continues to evolve with ongoing research and development efforts aimed at improving efficiency, reducing costs, and expanding the range of feedstocks and applications. The versatility, efficiency, and environmental benefits of gasification make it a promising technology for sustainable energy production and resource management in the transition towards a low-carbon economy.
Biomass:
Biomass refers to organic materials derived from living or recently living organisms, such as plants, trees, agricultural residues, algae, and organic waste. It is a renewable and abundant energy resource that can be converted into various forms of energy, including heat, electricity, and biofuels, through processes such as combustion, gasification, pyrolysis, and biochemical conversion. Biomass plays a significant role in the transition to a sustainable and low-carbon energy system due to its carbon-neutral nature and potential to replace fossil fuels in energy production and other applications.
The utilization of biomass for energy dates back to ancient times when humans used wood and other biomass materials for cooking, heating, and lighting. Today, biomass remains a vital energy resource, contributing to the global energy mix and providing numerous environmental, economic, and social benefits:
- Renewable Energy Source: Biomass is considered renewable because it can be replenished through natural processes such as photosynthesis and regrowth of plants. Unlike fossil fuels, which are finite and non-renewable, biomass can be sustainably managed and harvested to meet current and future energy needs without depleting natural resources.
- Carbon Neutrality: Biomass combustion and conversion processes release carbon dioxide (CO2) into the atmosphere, but the carbon emitted is part of the natural carbon cycle. Since biomass crops absorb CO2 during their growth phase, they effectively offset the CO2 emissions from their combustion, making biomass energy carbon-neutral over its lifecycle. This carbon neutrality distinguishes biomass from fossil fuels, which release additional CO2 stored underground and contribute to global warming.
- Waste Management and Recycling: Biomass energy production provides an opportunity to divert organic waste materials, such as agricultural residues, forestry residues, and food waste, from landfills and incinerators. By converting these waste materials into energy, biomass helps reduce methane emissions from decomposing organic matter and minimizes environmental pollution and landfill space requirements.
- Rural Development and Economic Opportunities: Biomass energy production can stimulate economic growth and create employment opportunities, particularly in rural areas where biomass resources are abundant. Biomass cultivation, harvesting, processing, and conversion activities generate jobs along the supply chain, supporting local economies and fostering sustainable development.
- Energy Security and Diversification: Biomass offers a decentralized and distributed energy source that can be produced and utilized locally, reducing reliance on imported fossil fuels and enhancing energy security. Biomass resources are available in diverse forms and locations worldwide, providing opportunities for energy diversification and resilience against supply disruptions.
- Bioenergy Applications: Biomass can be converted into various forms of bioenergy, including solid biomass (e.g., wood pellets, agricultural residues), liquid biofuels (e.g., ethanol, biodiesel), biogas (produced through anaerobic digestion of organic waste), and syngas (produced through biomass gasification). These bioenergy sources can be used for heating, electricity generation, transportation fuels, and industrial processes, offering versatile and sustainable alternatives to fossil fuels.
Overall, biomass represents a valuable renewable energy resource with significant potential to contribute to climate change mitigation, environmental protection, and sustainable development. However, effective biomass utilization requires careful consideration of environmental, social, and economic factors, as well as technological advancements and policy support to maximize its benefits while minimizing potential risks and trade-offs.
Syngas:
Syngas, short for synthesis gas, is a versatile mixture of gases primarily composed of hydrogen (H2) and carbon monoxide (CO), along with varying amounts of carbon dioxide (CO2), methane (CH4), and other trace gases. It is produced through the gasification of carbon-containing feedstocks such as biomass, coal, or natural gas, typically in the presence of a controlled amount of oxygen, steam, or a combination of both. Syngas serves as a valuable intermediate in various energy conversion processes and industrial applications due to its combustible nature and chemical reactivity.
The composition of syngas can vary depending on factors such as the feedstock type, gasification process conditions, and gasification technology employed. However, the primary constituents of syngas include:
- Hydrogen (H2): Hydrogen is a key component of syngas and serves as a clean and versatile energy carrier in various applications. It can be used directly as a fuel for heating, power generation, or transportation, or as a feedstock for chemical synthesis processes such as ammonia production, methanol synthesis, and hydrocarbon reforming.
- Carbon Monoxide (CO): Carbon monoxide is another important component of syngas and is often used as a reducing agent in chemical processes such as steel production, methanol synthesis, and Fischer-Tropsch synthesis. It can also be converted into hydrogen through water-gas shift reactions for further utilization in fuel cells or ammonia synthesis.
- Carbon Dioxide (CO2): Carbon dioxide is a byproduct of the gasification process and is typically present in syngas at varying concentrations. While CO2 is considered a greenhouse gas and contributes to global warming, it can also be captured and sequestered to reduce emissions or utilized in applications such as enhanced oil recovery, carbonation of concrete, or algae cultivation for biofuel production.
- Methane (CH4): Methane is a minor component of syngas and is typically produced through secondary reactions such as methanation or tar reforming. While methane can be utilized as a fuel for heating or power generation, its presence in syngas may need to be minimized to avoid undesirable combustion properties or emissions.
Syngas finds numerous applications across various industries, including:
- Power Generation: Syngas can be combusted in gas turbines, reciprocating engines, or fuel cells to generate electricity. Combined heat and power (CHP) systems can maximize energy efficiency by utilizing waste heat for heating or industrial processes.
- Biofuels Production: Syngas serves as a feedstock for the production of biofuels such as ethanol, methanol, biodiesel, and synthetic diesel through catalytic processes such as Fischer-Tropsch synthesis or methanol synthesis.
- Chemical Synthesis: Syngas is a key precursor for the synthesis of various chemicals and industrial products, including ammonia, methanol, hydrogen, synthetic natural gas (SNG), and higher alcohols.
- Hydrogen Production: Syngas can be converted into hydrogen through water-gas shift reactions or steam reforming processes, providing a clean and versatile energy carrier for fuel cell vehicles, industrial processes, and chemical manufacturing.
Overall, syngas represents a valuable intermediate in the utilization of carbon-containing feedstocks for energy production and industrial applications. Its versatility, combustibility, and chemical reactivity make it an essential component of the transition to a more sustainable and low-carbon energy system. Continued research and development efforts are focused on advancing syngas production technologies, improving process efficiency, and expanding its range of applications to address global energy and environmental challenges.
Reactor:
In the context of gasification and biomass energy production, a reactor refers to a vessel or system designed to facilitate the conversion of feedstock into useful products such as syngas, biofuels, or chemicals through controlled chemical reactions. Reactors play a central role in various biomass conversion processes, including gasification, pyrolysis, fermentation, and catalytic conversion, by providing the necessary conditions for biomass transformation while optimizing process efficiency, product yields, and quality.
The design and operation of biomass reactors are influenced by several factors, including feedstock characteristics, desired products, reaction kinetics, thermodynamics, heat and mass transfer phenomena, and process requirements. Reactor technologies range from simple batch reactors to complex continuous-flow systems, each offering distinct advantages and limitations depending on the specific application and scale of operation.
Here are some common types of reactors used in biomass conversion processes:
- Fixed-Bed Reactor:
- In a fixed-bed reactor, biomass feedstock is loaded into a stationary bed within the reactor vessel, where it undergoes conversion reactions under controlled temperature, pressure, and gas flow conditions.
- Fixed-bed reactors offer simplicity, reliability, and ease of operation, making them suitable for small-scale gasification, pyrolysis, and biochar production applications.
- However, fixed-bed reactors may suffer from limited heat and mass transfer, uneven temperature distribution, and bed agglomeration issues, particularly with high-moisture or high-ash feedstocks.
- Fluidized Bed Reactor:
- In a fluidized bed reactor, biomass feedstock is suspended and fluidized by a stream of gas (e.g., air, steam) flowing upward through the reactor vessel. The fluidized bed enhances mixing, heat transfer, and gas-solid contact, leading to efficient biomass conversion.
- Fluidized bed reactors offer high reaction rates, uniform temperature distribution, and tolerance to variations in feedstock properties, making them suitable for biomass gasification, pyrolysis, and combustion processes.
- However, fluidized bed reactors may require complex gas-solid separation systems and are susceptible to erosion and abrasion of reactor components.
- Entrained-Flow Reactor:
- In an entrained-flow reactor, finely ground biomass feedstock is entrained by a high-velocity stream of gas (e.g., oxygen, steam) and injected into a reaction chamber, where rapid conversion reactions occur in a turbulent, high-temperature environment.
- Entrained-flow reactors offer high reaction rates, high thermal efficiency, and flexibility in feedstock selection, making them suitable for biomass gasification and fast pyrolysis applications.
- However, entrained-flow reactors require robust refractory materials and precise control of gas flow and temperature to maintain stable operation.
- Hybrid Reactor:
- Hybrid reactors combine features of different reactor types to leverage their respective advantages and overcome limitations. For example, hybrid gasifiers may combine a fixed-bed or fluidized bed for biomass pyrolysis and partial oxidation with an entrained-flow reactor for complete gasification of char and tar.
- Hybrid reactors aim to achieve high conversion efficiency, improved product quality, and enhanced process flexibility for a wide range of biomass conversion applications.
Reactor design considerations include reactor geometry, size, shape, configuration, material selection, insulation, heating methods, gas distribution, mixing, residence time, and process control strategies. Advanced computational modeling, simulation, and experimental testing techniques are employed to optimize reactor designs and improve performance, reliability, and cost-effectiveness. Ongoing research and development efforts are focused on advancing reactor technologies, enhancing process efficiency, and enabling the widespread adoption of biomass-to-energy conversion systems for sustainable energy production.
Feedstock:
Feedstock refers to the raw material or biomass resource used as a primary input in various conversion processes to produce energy, fuels, chemicals, or other value-added products. In the context of biomass energy production, feedstock encompasses a wide range of organic materials derived from biological sources, including plants, trees, crops, agricultural residues, forestry residues, algae, and organic waste.
The selection of feedstock plays a critical role in determining the overall efficiency, economics, and environmental sustainability of biomass conversion processes. Different feedstocks have unique characteristics, including composition, moisture content, energy density, ash content, and availability, which influence their suitability for specific applications and conversion technologies.
Here are some common types of biomass feedstocks used in biomass energy production:
- Woody Biomass:
- Woody biomass includes trees, branches, bark, and wood residues from forestry operations, sawmills, and timber processing industries.
- Woody biomass is a widely available feedstock with high energy density and relatively low moisture content, making it suitable for combustion, gasification, and biochar production.
- Agricultural Residues:
- Agricultural residues are byproducts of crop cultivation and harvesting processes, such as straw, stalks, husks, shells, and bagasse.
- Agricultural residues are abundant, widely distributed, and often available at low cost, making them attractive feedstocks for bioenergy production, particularly in rural areas.
- Energy Crops:
- Energy crops are specifically grown for biomass energy production and include fast-growing plants such as switchgrass, miscanthus, willow, and poplar.
- Energy crops offer high biomass yields, rapid growth rates, and efficient use of land and resources, making them promising feedstocks for biofuels and biopower production.
- Municipal Solid Waste (MSW):
- Municipal solid waste consists of household, commercial, and industrial waste materials, including paper, cardboard, plastics, food scraps, and yard waste.
- MSW can be processed through mechanical or biological treatments to recover organic fractions suitable for anaerobic digestion, composting, or thermal conversion into energy.
- Algal Biomass:
- Algal biomass refers to microalgae or macroalgae cultivated in aquatic environments for biofuel production, wastewater treatment, carbon capture, and other applications.
- Algae offer high growth rates, efficient carbon fixation, and the ability to thrive in diverse environments, making them promising feedstocks for biofuel and bioproducts production.
The choice of feedstock depends on factors such as feedstock availability, cost, sustainability, environmental impact, conversion technology requirements, and end-product specifications. Sustainable feedstock sourcing practices, including land management, crop rotation, agroforestry, and waste-to-energy initiatives, are essential to ensure the long-term viability and environmental integrity of biomass energy production. Additionally, advancements in feedstock preprocessing, densification, storage, and logistics are needed to improve feedstock quality, handling, and supply chain efficiency for biomass conversion processes.
Tar:
Tar, also known as volatile organic compounds (VOCs) or tars, refers to a complex mixture of organic compounds produced during the thermal decomposition and gasification of biomass feedstocks. Tars are formed as byproducts of pyrolysis, combustion, and gasification reactions, particularly at elevated temperatures (>400°C), when organic molecules undergo incomplete conversion into gases such as carbon monoxide (CO), hydrogen (H2), methane (CH4), and carbon dioxide (CO2).
The composition and properties of tar vary depending on factors such as the feedstock type, gasification conditions, reactor design, residence time, and gasification technology employed. Tars consist of a wide range of hydrocarbons, oxygenated compounds, nitrogen-containing compounds, and trace elements, with molecular weights ranging from light volatiles to heavy, high-molecular-weight compounds.
Tars pose several challenges in biomass gasification and syngas utilization processes:
- Equipment Fouling: Tar compounds can condense and deposit on the surfaces of gasification reactor walls, heat exchangers, syngas pipelines, and downstream equipment, leading to fouling, plugging, and corrosion issues. Tar deposits reduce heat transfer efficiency, increase pressure drop, and degrade equipment performance, requiring frequent maintenance and cleaning to restore operation.
- Catalyst Deactivation: Tars can poison or deactivate catalyst materials used in downstream syngas cleanup, conditioning, and utilization processes. Catalyst deactivation occurs due to adsorption, deposition, and coking of tar compounds on the catalyst surface, inhibiting active sites and reducing catalytic activity and selectivity.
- Syngas Contamination: Tars present in syngas streams can contaminate downstream utilization pathways such as engines, turbines, fuel cells, and chemical reactors, leading to decreased efficiency, increased emissions, and equipment damage. Tar contaminants may cause fouling, erosion, corrosion, and catalyst poisoning, affecting system reliability and performance.
Several methods and technologies are employed for tar removal and mitigation in biomass gasification systems:
- Tar Cracking: Thermal or catalytic decomposition of tar molecules at high temperatures (>800°C) promotes the conversion of heavy tar compounds into lighter gases such as CO, H2, and methane (CH4). Tar cracking occurs naturally in the gasification reactor and downstream equipment, but additional tar cracking catalysts or reactors may be employed to enhance tar conversion efficiency.
- Tar Filtration: Passage of the raw syngas stream through porous filter media (e.g., ceramic or metallic filters) captures and removes tar particles and condensable compounds. Tar filtration is effective for removing coarse tar particles but may require periodic cleaning or replacement of filter elements.
- Catalytic Tar Reforming: Catalyst materials promote the cracking and reforming of tar compounds into simpler hydrocarbons and syngas components at lower temperatures and with higher selectivity. Tar reforming catalysts enhance tar conversion efficiency and minimize catalyst deactivation due to fouling or poisoning.
- Tar Scrubbing: Contacting the raw syngas stream with a liquid scrubbing solution (e.g., water, oil, solvents) absorbs tar compounds and condensable vapors. Tar scrubbing effectively removes tar contaminants but may require additional downstream processing steps to recover and recycle the scrubbing solution.
Tar removal technologies are essential for ensuring the reliability, efficiency, and environmental sustainability of biomass gasification systems. Effective tar removal minimizes equipment fouling, improves syngas quality, and enhances the economic viability of syngas utilization pathways for power generation, biofuels production, chemical synthesis, and industrial applications. Ongoing research and development efforts are focused on advancing tar removal technologies, optimizing process integration, and reducing costs to enable the widespread adoption of biomass-to-energy conversion systems for sustainable energy production.
Particulate:
Particulate matter (PM), often referred to simply as particulate, is a complex mixture of tiny solid particles and liquid droplets suspended in air. In the context of biomass gasification and energy production, particulate matter is generated during the thermal conversion of biomass feedstocks and can include a variety of organic and inorganic compounds, such as ash, char, soot, tar, and aerosols.
The formation and characteristics of particulate matter depend on various factors, including the composition and properties of the biomass feedstock, gasification process conditions (e.g., temperature, pressure, residence time), reactor design, gas composition, and combustion kinetics. Particulate matter can vary in size, shape, density, chemical composition, and reactivity, influencing its behavior, transport, and environmental impacts.
Particulate matter poses several challenges and considerations in biomass gasification and energy production systems:
- Air Quality and Health Effects: Inhalation of particulate matter can pose significant health risks, particularly for vulnerable populations such as children, the elderly, and individuals with respiratory or cardiovascular conditions. Fine particulate matter (PM2.5) can penetrate deep into the lungs and bloodstream, causing respiratory problems, cardiovascular diseases, and premature mortality. Therefore, minimizing particulate emissions from biomass gasification processes is essential to protect public health and ensure compliance with air quality regulations.
- Environmental Impacts: Particulate matter emissions from biomass gasification can contribute to environmental pollution, including smog formation, visibility impairment, acid deposition, and ecosystem damage. Particulate matter can also serve as a carrier for hazardous pollutants such as heavy metals, polycyclic aromatic hydrocarbons (PAHs), and dioxins, which pose risks to human health and the environment.
- Equipment Fouling: Particulate matter can deposit on the surfaces of gasification reactor walls, heat exchangers, syngas pipelines, and downstream equipment, leading to fouling, plugging, and corrosion issues. Particulate deposits reduce heat transfer efficiency, increase pressure drop, and degrade equipment performance, necessitating frequent maintenance and cleaning to restore operation.
- Syngas Quality and Utilization: Particulate matter present in syngas streams can contaminate downstream utilization pathways such as engines, turbines, fuel cells, and chemical reactors, leading to decreased efficiency, increased emissions, and equipment damage. Particulate contaminants may cause fouling, erosion, corrosion, and catalyst deactivation, affecting system reliability and performance.
To mitigate the impacts of particulate matter emissions from biomass gasification processes, various control measures and technologies are employed:
- Particulate Removal Systems: Mechanical collectors, such as cyclones, electrostatic precipitators (ESPs), fabric filters (baghouses), and wet scrubbers, are used to capture and remove particulate matter from gas streams. These systems rely on principles such as inertia, electrostatic attraction, filtration, and impaction to separate particles from the gas phase.
- Gasification Process Optimization: Adjusting gasification process parameters, such as temperature, residence time, and gas flow rates, can influence particulate formation and emissions. Optimizing process conditions can minimize the generation of fine particulate matter and promote the conversion of volatile organic compounds into syngas constituents.
- Fuel Preparation and Handling: Preprocessing biomass feedstocks through drying, grinding, pelletizing, or densification can reduce moisture content, particle size, and variability, leading to more uniform combustion or gasification behavior and lower particulate emissions.
- Advanced Gasification Technologies: Advanced gasification technologies, such as fluidized bed reactors, entrained-flow reactors, and hybrid gasification systems, offer improved mixing, heat transfer, and gas-solid contact, leading to enhanced combustion efficiency and reduced particulate emissions.
- Particulate Monitoring and Control: Continuous monitoring of particulate emissions from biomass gasification systems is essential to assess compliance with regulatory standards, optimize process performance, and identify opportunities for emission reduction. Real-time particulate monitoring technologies, such as optical instruments, laser-based sensors, and electrochemical sensors, enable rapid detection and response to changes in particulate levels.
Overall, minimizing particulate matter emissions from biomass gasification processes is essential to protect human health, safeguard the environment, and ensure the sustainability of biomass energy production. Effective particulate control measures and technologies enable the development of cleaner and more efficient biomass gasification systems for sustainable energy generation and resource utilization.
Fluidized Bed Reactor:
A fluidized bed reactor is a type of reactor used in various chemical, petrochemical, and energy production processes, including biomass gasification, combustion, pyrolysis, and catalytic reactions. It operates on the principle of fluidization, where a bed of solid particles is suspended and behaves like a fluid when subjected to a flow of gas. In biomass gasification, fluidized bed reactors are widely used due to their ability to handle a wide range of feedstocks, high reaction rates, efficient heat and mass transfer, and flexibility in operation.
Here’s how a fluidized bed reactor works in the context of biomass gasification:
- Bed Material: The reactor contains a bed of inert solid particles, typically sand, alumina, or silica, which serves as the support medium for biomass feedstock. The particle size and density are carefully selected to achieve fluidization under the desired operating conditions.
- Gas Flow: A gas, typically air, oxygen, steam, or a mixture of gases, is introduced into the bottom of the reactor and passes through the bed of solid particles. The upward flow of gas fluidizes the bed, causing the particles to behave like a fluid with characteristics similar to boiling water.
- Biomass Feedstock Injection: Biomass feedstock, such as wood chips, agricultural residues, or energy crops, is injected into the fluidized bed reactor either directly or through a feeding mechanism. The biomass particles mix with the fluidized bed and undergo thermal decomposition and gasification reactions in the presence of the gasification agent.
- Reaction Zone: Within the fluidized bed reactor, biomass feedstock undergoes a series of complex thermochemical reactions, including drying, pyrolysis, combustion, and gasification. These reactions occur at elevated temperatures (typically 700°C to 1000°C) and in the presence of limited oxygen or steam to produce a mixture of gases known as syngas.
- Heat Transfer: Heat generated by exothermic gasification reactions is transferred to the surrounding bed material and biomass particles, promoting thermal decomposition and gasification. The fluidized bed configuration enhances heat transfer efficiency by ensuring intimate contact between the solid particles and the reacting gases.
- Syngas Production: The primary product of biomass gasification in a fluidized bed reactor is syngas, a mixture of hydrogen (H2), carbon monoxide (CO), carbon dioxide (CO2), methane (CH4), and other trace gases. The composition of syngas depends on factors such as the biomass feedstock, gasification conditions, and reactor design.
- Gas-Solid Separation: After gasification reactions occur, the syngas rises through the fluidized bed and exits the reactor through an outlet at the top. Particulate matter, ash, and unreacted char particles are entrained in the gas stream and may be separated using cyclones, filters, or other gas-solid separation devices before further processing or utilization.
Fluidized bed reactors offer several advantages for biomass gasification applications:
- High Reaction Rates: Fluidized bed reactors provide excellent mixing and gas-solid contact, leading to rapid biomass conversion and high reaction rates.
- Flexibility: Fluidized bed reactors can accommodate a wide range of biomass feedstocks, including low-quality or high-moisture materials, without significant changes to the reactor design.
- Good Heat Transfer: The fluidized bed configuration enhances heat transfer efficiency, allowing for effective thermal decomposition and gasification of biomass feedstock.
- Reduced Tar Formation: Fluidized bed reactors promote tar cracking and reforming reactions, minimizing tar formation and improving syngas quality compared to other gasification technologies.
However, fluidized bed reactors also present some challenges, including the potential for bed agglomeration, particle attrition, elutriation of fine particles, and erosion of reactor components. Proper reactor design, operation, and maintenance are essential to mitigate these issues and ensure the reliable and efficient operation of fluidized bed biomass gasification systems. Ongoing research and development efforts are focused on advancing fluidized bed reactor technologies, optimizing process performance, and expanding their applications in sustainable energy production.
Gasification:
Gasification is a thermochemical conversion process that transforms carbonaceous materials, such as biomass, coal, or organic waste, into a mixture of gases known as syngas (synthesis gas) through partial oxidation in a controlled environment. This process involves the reaction of the carbonaceous feedstock with a gasification agent, typically air, oxygen, steam, or a combination thereof, at elevated temperatures (> 700°C) and pressure. Gasification offers a versatile and efficient means of converting a wide range of feedstocks into a valuable energy carrier and chemical precursor.
Here’s how the gasification process works:
- Feedstock Preparation: The carbonaceous feedstock, such as biomass (e.g., wood chips, agricultural residues) or coal, is first prepared by sizing, drying, and grinding to facilitate handling, transportation, and feeding into the gasification reactor. Feedstock preparation may also involve removing contaminants and moisture to improve gasification efficiency.
- Gasification Reactor: The prepared feedstock is fed into a gasification reactor, which can take various forms depending on the specific technology employed. Common gasification reactor designs include fixed-bed reactors, fluidized bed reactors, entrained-flow reactors, and hybrid configurations. The reactor provides the necessary conditions for the thermochemical conversion of the feedstock into syngas, including elevated temperatures, controlled oxygen or steam supply, and sufficient residence time.
- Thermochemical Reactions: Within the gasification reactor, the carbonaceous feedstock undergoes a series of complex thermochemical reactions in the presence of the gasification agent. These reactions include:
- Drying: Removal of moisture from the feedstock at temperatures below 100°C.
- Pyrolysis: Thermal decomposition of organic matter in the absence of oxygen to produce volatile gases, tar, and char.
- Combustion: Oxidation of char and volatile gases in the presence of oxygen to release heat and produce carbon dioxide (CO2) and water vapor (H2O).
- Gasification: Reaction of carbon (C) with steam (H2O) or carbon dioxide (CO2) to produce hydrogen (H2) and carbon monoxide (CO) via the water-gas shift reaction or the carbon-gas shift reaction, respectively.
- Syngas Production: The primary product of gasification is syngas, a mixture of hydrogen (H2), carbon monoxide (CO), carbon dioxide (CO2), methane (CH4), and other trace gases. The composition of syngas depends on factors such as the feedstock type, gasification conditions, and reactor design. Syngas has a variety of potential applications, including power generation, heat production, biofuels synthesis, chemical manufacturing, and as a feedstock for hydrogen production.
- Gas Cleaning and Conditioning: The raw syngas produced from the gasification reactor typically contains impurities such as tar, particulate matter, sulfur compounds, and trace metals, which must be removed or reduced to acceptable levels before further utilization. Gas cleaning and conditioning processes may include filtration, scrubbing, catalytic conversion, and gas cooling to improve syngas quality and stability.
- Syngas Utilization: Cleaned and conditioned syngas can be utilized in various downstream processes and applications, including:
- Power Generation: Syngas can be combusted in gas turbines, reciprocating engines, or fuel cells to generate electricity and heat in combined heat and power (CHP) systems.
- Biofuels Production: Syngas serves as a feedstock for the production of biofuels such as ethanol, methanol, synthetic diesel, and Fischer-Tropsch fuels through catalytic synthesis processes.
- Chemical Synthesis: Syngas is a key precursor for the synthesis of various chemicals and industrial products, including ammonia, methanol, hydrogen, synthetic natural gas (SNG), and higher alcohols.
Gasification offers several advantages over traditional combustion technologies, including higher energy efficiency, lower emissions, and greater fuel flexibility. It enables the conversion of a wide range of feedstocks, including low-grade and waste materials, into valuable energy products, contributing to resource utilization, waste management, and renewable energy production goals. Ongoing research and development efforts are focused on advancing gasification technologies, improving process efficiency, and expanding the range of feedstocks and applications for sustainable energy production.
Syngas:
Syngas, short for synthesis gas, is a mixture of gases primarily composed of hydrogen (H2) and carbon monoxide (CO), along with varying amounts of carbon dioxide (CO2), methane (CH4), water vapor (H2O), and trace impurities. It is produced through the gasification of carbonaceous feedstocks such as biomass, coal, natural gas, or organic waste in the presence of a gasification agent, typically air, oxygen, steam, or a combination thereof. Syngas serves as a versatile intermediate product that can be utilized in a wide range of industrial processes and energy applications.
Here are some key characteristics and applications of syngas:
- Composition: The composition of syngas varies depending on factors such as the type of feedstock, gasification process conditions, and reactor design. Typically, syngas contains hydrogen (H2) and carbon monoxide (CO) as the primary components, with hydrogen content ranging from 15% to 50% by volume and carbon monoxide content ranging from 20% to 40% by volume. The ratio of hydrogen to carbon monoxide, known as the H2/CO ratio, influences the potential applications of syngas.
- Energy Content: Syngas has a high energy content due to the presence of hydrogen and carbon monoxide, which can be combusted or converted into other fuels and chemicals through various synthesis processes. The calorific value of syngas depends on its composition and typically ranges from 4 to 12 megajoules per cubic meter (MJ/m³), depending on the hydrogen content.
- Applications:
- Power Generation: Syngas can be used as a fuel for power generation in gas turbines, reciprocating engines, or fuel cells. Combined heat and power (CHP) systems utilize syngas to produce both electricity and heat, improving overall energy efficiency.
- Biofuels Production: Syngas serves as a feedstock for the production of biofuels such as ethanol, methanol, synthetic diesel, and Fischer-Tropsch fuels through catalytic synthesis processes. These biofuels can be used as transportation fuels or blended with conventional fuels to reduce greenhouse gas emissions.
- Chemical Synthesis: Syngas is a key precursor for the synthesis of various chemicals and industrial products, including ammonia, methanol, hydrogen, synthetic natural gas (SNG), and higher alcohols. These chemicals are used in fertilizer production, petrochemical manufacturing, hydrogen production, and other industrial processes.
- Hydrogen Production: Syngas can be converted into pure hydrogen (H2) through processes such as water-gas shift reaction (WGSR) or steam methane reforming (SMR). Hydrogen is a clean and versatile energy carrier used in fuel cells, ammonia production, refining, and chemical synthesis.
- Carbon Capture and Utilization (CCU): Syngas can serve as a feedstock for carbon capture and utilization (CCU) processes, where carbon dioxide (CO2) is captured from syngas streams and converted into value-added products such as synthetic fuels, chemicals, and building materials.
Syngas production can be tailored to meet specific requirements for different applications by adjusting gasification process parameters, such as feedstock composition, gasification agent ratio, temperature, pressure, and residence time. Advances in gasification technology, catalyst development, and process optimization continue to expand the range of syngas-based applications and contribute to the transition toward cleaner and more sustainable energy systems.
Biomass Gasification Plant:
A biomass gasification plant is a facility that converts biomass feedstocks into syngas (synthesis gas) through the gasification process. These plants play a crucial role in the production of renewable energy, biofuels, and biochemicals by utilizing organic materials such as wood, agricultural residues, energy crops, and organic waste as feedstocks. Biomass gasification plants typically consist of several key components and processes designed to efficiently convert biomass into syngas while minimizing environmental impacts.
Here are the main components and processes involved in a biomass gasification plant:
- Feedstock Handling and Preparation: The biomass feedstock, sourced from forestry operations, agriculture, or organic waste streams, is transported to the gasification plant and undergoes various preparation steps. This may include chipping, shredding, grinding, or drying to optimize feedstock characteristics such as particle size, moisture content, and chemical composition. Proper feedstock preparation ensures uniform feeding and efficient conversion in the gasification process.
- Gasification Reactor: The heart of the biomass gasification plant is the gasification reactor, where the biomass feedstock undergoes thermochemical conversion to produce syngas. Gasification reactors can take various forms, including fixed-bed, fluidized bed, entrained-flow, or hybrid configurations. These reactors operate at elevated temperatures (>700°C) and in the presence of a gasification agent (air, oxygen, steam) to promote the partial oxidation and decomposition of biomass into syngas constituents.
- Gas Cleaning and Conditioning: The raw syngas produced from the gasification reactor contains impurities such as tar, particulate matter, sulfur compounds, and trace metals, which must be removed or reduced to acceptable levels before further utilization. Gas cleaning and conditioning processes may include filtration, scrubbing, catalytic conversion, and cooling to improve syngas quality, stability, and compatibility with downstream applications.
- Syngas Utilization: Cleaned and conditioned syngas can be utilized in various downstream processes and applications, including:
- Power Generation: Syngas can be combusted in gas turbines, reciprocating engines, or fuel cells to generate electricity and heat in combined heat and power (CHP) systems.
- Biofuels Production: Syngas serves as a feedstock for the production of biofuels such as ethanol, methanol, synthetic diesel, and Fischer-Tropsch fuels through catalytic synthesis processes.
- Chemical Synthesis: Syngas is a key precursor for the synthesis of various chemicals and industrial products, including ammonia, methanol, hydrogen, synthetic natural gas (SNG), and higher alcohols.
- Hydrogen Production: Syngas can be converted into pure hydrogen (H2) through processes such as water-gas shift reaction (WGSR) or steam methane reforming (SMR) for use in fuel cells, refining, and chemical synthesis.
- Residue Management: Residues generated during the gasification process, such as ash, char, and tar, are collected and managed to minimize environmental impacts and maximize resource recovery. Ash may be utilized as a soil amendment or construction material, while char can be recycled as a soil conditioner or carbon source. Tar and other liquid byproducts may undergo further processing or treatment for disposal or value-added utilization.
Biomass gasification plants offer several advantages over traditional combustion-based systems, including higher energy efficiency, lower emissions, and greater fuel flexibility. They enable the conversion of diverse biomass feedstocks into valuable energy products, contributing to renewable energy deployment, waste reduction, and sustainable development goals. Ongoing research and development efforts focus on advancing gasification technologies, optimizing process efficiency, and expanding the range of feedstocks and applications for biomass gasification plants.
Biomass Gasification Process:
Biomass gasification is a thermochemical conversion process that converts biomass feedstocks into a gaseous mixture known as syngas (synthesis gas). This process involves the partial oxidation of biomass in a controlled environment to produce a combustible gas containing hydrogen (H2), carbon monoxide (CO), carbon dioxide (CO2), methane (CH4), and other trace gases. The syngas produced can be used for various energy applications, including power generation, heat production, biofuels synthesis, and chemical manufacturing.
Here’s a detailed overview of the biomass gasification process:
- Feedstock Preparation: Biomass feedstocks such as wood chips, agricultural residues, energy crops, or organic waste are first collected, sorted, and prepared for gasification. Feedstock preparation may involve shredding, chipping, drying, and sizing to optimize characteristics such as particle size, moisture content, and chemical composition. Proper feedstock preparation ensures efficient handling, feeding, and conversion in the gasification reactor.
- Gasification Reactor: The prepared biomass feedstock is fed into a gasification reactor, where it undergoes thermochemical conversion in the presence of a gasification agent. Gasification reactors can be classified into various types, including fixed-bed, fluidized bed, entrained-flow, and hybrid configurations. These reactors operate at elevated temperatures (>700°C) and in controlled atmospheres to promote the partial oxidation and decomposition of biomass into syngas constituents.
- Gasification Reactions: Within the gasification reactor, several thermochemical reactions occur sequentially, resulting in the production of syngas:
- Drying: Moisture present in the biomass feedstock is first evaporated and removed at temperatures below 100°C.
- Pyrolysis: The dried biomass undergoes thermal decomposition in the absence of oxygen to produce volatile gases, tar, and char. Pyrolysis occurs at temperatures typically ranging from 200°C to 700°C, depending on the biomass composition and heating rate.
- Char Gasification: The char residue from pyrolysis reacts with a gasification agent (air, oxygen, steam) at high temperatures (>700°C) to produce syngas constituents, primarily hydrogen (H2) and carbon monoxide (CO). The gasification reactions involve the partial oxidation of carbon (C) with oxygen (O2), water vapor (H2O), or carbon dioxide (CO2) to generate syngas and heat.
- Gas Cleaning and Conditioning: The raw syngas produced from the gasification reactor contains impurities such as tar, particulate matter, sulfur compounds, and trace metals, which must be removed or reduced to acceptable levels before further utilization. Gas cleaning and conditioning processes may include filtration, scrubbing, catalytic conversion, and cooling to improve syngas quality, stability, and compatibility with downstream applications.
- Syngas Utilization: Cleaned and conditioned syngas can be utilized in various downstream processes and applications, including:
- Power Generation: Syngas can be combusted in gas turbines, reciprocating engines, or fuel cells to generate electricity and heat in combined heat and power (CHP) systems.
- Biofuels Production: Syngas serves as a feedstock for the production of biofuels such as ethanol, methanol, synthetic diesel, and Fischer-Tropsch fuels through catalytic synthesis processes.
- Chemical Synthesis: Syngas is a key precursor for the synthesis of various chemicals and industrial products, including ammonia, methanol, hydrogen, synthetic natural gas (SNG), and higher alcohols.
Biomass gasification offers several advantages over traditional combustion-based systems, including higher energy efficiency, lower emissions, and greater fuel flexibility. It enables the conversion of diverse biomass feedstocks into valuable energy products, contributing to renewable energy deployment, waste reduction, and sustainable development goals. Ongoing research and development efforts focus on advancing gasification technologies, optimizing process efficiency, and expanding the range of feedstocks and applications for biomass gasification.
Syngas Cleanup:
Syngas cleanup is a crucial step in the biomass gasification process, aimed at removing impurities and contaminants from the raw syngas produced in the gasification reactor. The syngas generated from biomass gasification typically contains various undesired components, including tar, particulate matter, sulfur compounds, nitrogen oxides (NOx), trace metals, and ash particles. These impurities can have detrimental effects on downstream equipment, catalysts, and processes if not properly removed or reduced to acceptable levels. Syngas cleanup technologies are employed to enhance the quality, stability, and compatibility of syngas for subsequent utilization in power generation, biofuels production, chemical synthesis, and other applications.
Here are some key aspects of syngas cleanup:
- Tar Removal: Tar is a complex mixture of organic compounds formed during the pyrolysis and gasification of biomass feedstocks. Tar can cause fouling, corrosion, and catalyst deactivation in downstream equipment and processes. Various tar removal techniques are employed in syngas cleanup, including tar cracking, tar reforming, tar condensation, and tar scrubbing. These methods aim to decompose or remove tar compounds through thermal, catalytic, or physical processes to improve syngas quality and stability.
- Particulate Removal: Particulate matter, including char particles, ash, and unburned carbon residues, may be present in the raw syngas stream from biomass gasification. Particulate removal is essential to prevent fouling, erosion, and abrasion in downstream equipment such as gas turbines, engines, and heat exchangers. Filtration, cyclones, electrostatic precipitators, and scrubbers are commonly used to remove particulate matter from syngas streams through mechanical or electrostatic separation methods.
- Sulfur and Nitrogen Removal: Sulfur compounds (e.g., hydrogen sulfide, sulfur dioxide) and nitrogen oxides (NOx) can be present in syngas streams as impurities or byproducts of biomass gasification. These compounds are corrosive, toxic, and can inhibit catalysts in downstream processes. Syngas cleanup technologies such as desulfurization and denitrification processes are employed to remove sulfur and nitrogen compounds from syngas through chemical absorption, adsorption, or catalytic conversion reactions.
- Trace Metal Removal: Trace metals, such as mercury, arsenic, lead, and cadmium, may be present in biomass feedstocks or introduced during gasification processes. These metals can poison catalysts, promote corrosion, and pose environmental and health risks if emitted into the atmosphere. Syngas cleanup systems utilize sorbents, adsorbents, or catalytic converters to capture and remove trace metal contaminants from syngas streams through chemical or physical processes.
- Gas Cooling and Conditioning: Syngas cleanup processes may involve gas cooling and conditioning to adjust temperature, pressure, and moisture levels for downstream utilization or storage. Cooling syngas helps condense water vapor and other volatile compounds, facilitating their removal and improving syngas quality. Gas conditioning systems may include heat exchangers, condensers, and compressors to control syngas temperature, pressure, and humidity.
Syngas cleanup technologies play a critical role in maximizing the efficiency, reliability, and environmental performance of biomass gasification systems. By removing impurities and contaminants from syngas streams, these technologies enable the production of clean and high-quality syngas suitable for a wide range of energy and chemical applications. Ongoing research and development efforts focus on advancing syngas cleanup technologies, optimizing process performance, and reducing costs to enable the widespread deployment of biomass gasification for renewable energy production and sustainable development.
Syngas Utilization:
Syngas, produced through biomass gasification, is a versatile energy carrier that can be utilized in various industrial processes and applications. Its composition, primarily consisting of hydrogen (H2) and carbon monoxide (CO), along with other gases such as carbon dioxide (CO2), methane (CH4), and trace impurities, makes it suitable for power generation, biofuels production, chemical synthesis, and other uses. Syngas utilization plays a pivotal role in the development of sustainable energy systems by harnessing renewable resources and reducing greenhouse gas emissions.
Here are some key applications of syngas utilization:
- Power Generation:Syngas can be combusted in gas turbines, reciprocating engines, or fuel cells to generate electricity and heat in combined heat and power (CHP) systems. Gasification-based power plants utilize syngas as a fuel to drive electricity generators, providing a reliable and renewable source of energy for grid-connected and off-grid applications. The high energy content of syngas and the efficiency of modern power generation technologies contribute to the competitiveness of biomass gasification in the electricity market.
- Biofuels Production:Syngas serves as a feedstock for the production of biofuels such as ethanol, methanol, synthetic diesel, and Fischer-Tropsch fuels through catalytic synthesis processes. These biofuels offer cleaner alternatives to conventional fossil fuels, with reduced greenhouse gas emissions and lower environmental impact. Syngas-derived biofuels can be blended with petroleum-based fuels or used as standalone alternatives in transportation, heating, and industrial applications, contributing to energy security and sustainability objectives.
- Chemical Synthesis:Syngas is a key precursor for the synthesis of various chemicals and industrial products, including ammonia, methanol, hydrogen, synthetic natural gas (SNG), and higher alcohols. These chemicals serve as building blocks for a wide range of downstream industries, including agriculture, pharmaceuticals, plastics, and manufacturing. Syngas-derived chemicals offer renewable alternatives to petrochemical-based products, reducing dependence on fossil fuels and mitigating environmental impacts associated with chemical production.
- Hydrogen Production:Syngas can be converted into pure hydrogen (H2) through processes such as water-gas shift reaction (WGSR) or steam methane reforming (SMR). Hydrogen is a clean and versatile energy carrier used in fuel cells, ammonia production, refining, and chemical synthesis. Syngas-derived hydrogen plays a crucial role in decarbonizing transportation, industry, and power sectors by enabling the transition to hydrogen-based energy systems and facilitating renewable integration.
- Carbon Capture and Utilization (CCU):Syngas can serve as a feedstock for carbon capture and utilization (CCU) processes, where carbon dioxide (CO2) is captured from syngas streams and converted into value-added products such as synthetic fuels, chemicals, and building materials. CCU technologies offer a pathway to mitigate greenhouse gas emissions by recycling CO2 into useful commodities, thereby closing the carbon cycle and promoting circular economy principles.
Syngas utilization represents a key strategy for maximizing the value and sustainability of biomass gasification systems. By converting renewable biomass feedstocks into clean energy products and industrial commodities, syngas enables the transition towards a low-carbon economy and contributes to global efforts to mitigate climate change and secure energy supplies. Continued innovation, investment, and deployment of syngas utilization technologies are essential for realizing the full potential of biomass gasification as a cornerstone of sustainable energy systems.
Gasification Biomass Feedstock:
In the context of biomass gasification, the term “feedstock” refers to the organic material used as the primary input for the gasification process. The selection of an appropriate feedstock is critical to the efficiency, economics, and environmental sustainability of biomass gasification systems. A wide range of biomass feedstocks can be utilized for gasification, including:
- Wood and Forestry Residues:Wood biomass, including logs, chips, sawdust, bark, and forestry residues, is one of the most common feedstocks for biomass gasification. These materials are readily available from forestry operations, sawmills, and wood processing industries. Wood biomass offers advantages such as high energy density, low moisture content, and relatively uniform composition, making it suitable for gasification processes.
- Agricultural Residues:Agricultural residues, such as straw, husks, stalks, and bagasse, are abundant byproducts of crop cultivation and harvesting. These residues can be used as feedstocks for biomass gasification, providing a renewable source of energy while reducing agricultural waste and emissions. Agricultural residues vary in composition and availability depending on crop types, seasons, and geographic regions.
- Energy Crops:Energy crops are specifically cultivated for biomass production and energy generation purposes. These crops, including switchgrass, miscanthus, willow, and poplar, offer high biomass yields and rapid growth rates, making them suitable feedstocks for biomass gasification. Energy crops can be grown on marginal lands unsuitable for food crops, contributing to sustainable land use practices and biomass supply chains.
- Organic Waste:Organic waste materials, such as municipal solid waste (MSW), food waste, sewage sludge, and animal manure, can be utilized as feedstocks for biomass gasification. Recycling organic waste streams into energy products reduces landfilling, methane emissions, and environmental pollution while providing renewable energy and resource recovery opportunities. Organic waste feedstocks may require preprocessing and conditioning to remove contaminants and improve gasification performance.
- Algae and Aquatic Biomass:Algae and aquatic biomass, including microalgae, seaweed, and aquatic plants, are potential feedstocks for biomass gasification. These biomass sources offer high productivity, rapid growth rates, and minimal land requirements compared to terrestrial crops. Algae cultivation can utilize non-arable land, saline water, and wastewater resources, providing additional environmental benefits and ecosystem services.
- Biodegradable Byproducts:Biodegradable byproducts from industries such as pulp and paper, food processing, and bioethanol production can serve as feedstocks for biomass gasification. These byproducts, including black liquor, spent grains, and lignocellulosic residues, contain organic carbon suitable for energy recovery and value-added utilization. Gasification of biodegradable byproducts can improve resource efficiency, reduce waste disposal costs, and enhance overall process sustainability.
The selection of a biomass feedstock for gasification depends on factors such as feedstock availability, cost, quality, energy content, logistics, and environmental considerations. Feedstock characteristics such as moisture content, ash content, and chemical composition influence gasification performance, syngas quality, and downstream applications. Integrating diverse feedstocks into biomass gasification systems can enhance feedstock flexibility, supply chain resilience, and overall system sustainability. Ongoing research and development efforts focus on optimizing feedstock utilization, improving gasification technology, and advancing biomass-to-energy conversion pathways for renewable energy production.
Biomass Gasification Reactor:
The biomass gasification reactor is a critical component of biomass gasification systems, where the thermochemical conversion of biomass feedstocks into syngas (synthesis gas) occurs. The design and operation of the gasification reactor significantly influence gasification performance, syngas composition, and overall system efficiency. Various reactor configurations and technologies are employed to achieve efficient biomass conversion while minimizing environmental impacts and maximizing syngas quality.
Here are the key aspects of biomass gasification reactors:
- Reactor Types:Biomass gasification reactors can be classified into several types based on their design, operating principles, and flow regimes. Common reactor configurations include:
- Fixed-Bed Gasifiers: Biomass feedstock is placed in a fixed bed within the reactor, and gasification reactions occur as a downward-moving gasification agent (air, oxygen, steam) flows through the bed. Fixed-bed gasifiers include updraft, downdraft, and cross-draft configurations, each offering unique advantages in terms of tar reduction, heat transfer, and syngas quality.
- Fluidized Bed Gasifiers: Biomass particles are suspended and fluidized by a gasification agent (typically air or steam) within the reactor, promoting intimate contact between the biomass and the gas phase. Fluidized bed gasifiers offer high heat and mass transfer rates, uniform temperature distribution, and enhanced tar cracking capabilities, making them suitable for a wide range of biomass feedstocks.
- Entrained-Flow Gasifiers: Biomass feedstock is entrained and rapidly converted into syngas as a high-velocity gasification agent (oxygen or steam) carries the biomass particles through the reactor. Entrained-flow gasifiers operate at high temperatures and pressures, enabling efficient biomass conversion, tar destruction, and syngas cleanup. They are commonly used in large-scale gasification plants for power generation and biofuels production.
- Operating Conditions:Gasification reactor operating conditions, including temperature, pressure, residence time, and gasification agent ratio, play a crucial role in determining gasification performance and syngas composition. Elevated temperatures (>700°C) are typically required to initiate and sustain gasification reactions, promoting the thermal decomposition of biomass into syngas constituents. Control of residence time and gasification agent flow rates ensures sufficient contact between the biomass and the gas phase, optimizing conversion efficiency and syngas yield.
- Gasification Mechanisms:Biomass gasification involves several thermochemical reactions, including pyrolysis, oxidation, reforming, and gasification. Pyrolysis converts biomass into volatile gases, tar, and char at elevated temperatures in the absence of oxygen. Oxidation reactions consume oxygen and produce heat, promoting the partial oxidation of carbon in the biomass to carbon monoxide (CO) and hydrogen (H2). Reforming reactions further convert tar and volatile hydrocarbons into CO and H2 through steam reforming, water-gas shift, and tar cracking mechanisms.
- Tar Reduction and Management:Tar compounds, formed during biomass pyrolysis and gasification, can condense and accumulate in gasification reactors, causing fouling, corrosion, and catalyst deactivation. Tar reduction and management strategies are employed to mitigate these issues and improve syngas quality. These strategies include reactor design modifications, operating parameter adjustments, tar cracking catalysts, and downstream tar removal systems such as scrubbers and filters.
- Syngas Quality and Cleanup:The design and operation of the gasification reactor significantly influence syngas composition, quality, and contaminants levels. Gasification reactor technologies that promote efficient biomass conversion and tar destruction contribute to cleaner syngas with reduced tar, particulate matter, sulfur compounds, and trace contaminants. Syngas cleanup systems further refine and condition the raw syngas to meet specific quality requirements for downstream applications, such as power generation, biofuels production, and chemical synthesis.
The selection of a biomass gasification reactor depends on factors such as feedstock characteristics, scale of operation, desired syngas quality, and process requirements. Advances in reactor design, materials, and control systems continue to improve gasification performance, flexibility, and reliability, driving the deployment of biomass gasification as a key technology for renewable energy production and sustainable development.
Gasification Process Efficiency:
Gasification process efficiency refers to the effectiveness with which biomass feedstocks are converted into syngas (synthesis gas) while minimizing energy losses and environmental impacts. Improving gasification process efficiency is essential for maximizing the utilization of biomass resources, enhancing energy conversion performance, and achieving economic viability in biomass-to-energy systems. Several factors influence the efficiency of the gasification process:
- Feedstock Characteristics:The composition, moisture content, particle size, and chemical properties of biomass feedstocks significantly impact gasification process efficiency. High-quality feedstocks with low moisture content, uniform particle size distribution, and favorable chemical composition promote efficient biomass conversion and syngas production. Proper feedstock preparation and handling are essential to optimize gasification performance and minimize energy losses.
- Gasification Reactor Design:The design and configuration of the gasification reactor play a crucial role in determining gasification process efficiency. Factors such as reactor type (e.g., fixed-bed, fluidized bed, entrained-flow), operating parameters (e.g., temperature, pressure, residence time), and gasification agent selection influence biomass conversion kinetics, syngas yield, and tar formation. Advanced reactor designs and technologies that enhance heat and mass transfer, minimize heat losses, and promote uniform biomass conversion contribute to improved gasification efficiency.
- Gasification Agent:The choice of gasification agent (e.g., air, oxygen, steam) affects gasification process efficiency and syngas composition. Oxygen-blown gasification systems offer higher reaction rates and syngas yields compared to air-blown systems due to the absence of nitrogen dilution and reduced gas volume. Steam addition promotes steam reforming reactions, increases hydrogen yield, and reduces tar formation, leading to cleaner syngas production. Selecting the appropriate gasification agent based on process requirements and feedstock characteristics is essential for optimizing gasification efficiency.
- Operating Conditions:Gasification process efficiency is influenced by operating conditions such as temperature, pressure, gasification agent ratio, and residence time. Elevated temperatures (>700°C) promote biomass pyrolysis, char gasification, and tar cracking reactions, leading to higher syngas yields and cleaner gasification products. Control of residence time and gasification agent flow rates ensures sufficient contact between the biomass and the gas phase, optimizing conversion efficiency and energy utilization.
- Syngas Cleanup and Conditioning:Efficient syngas cleanup and conditioning systems are essential for improving gasification process efficiency and syngas quality. Tar removal, particulate filtration, sulfur and nitrogen removal, and trace metal capture contribute to cleaner syngas streams with reduced contaminants levels. Gas cooling and conditioning technologies adjust syngas temperature, pressure, and humidity for downstream utilization or storage, minimizing energy losses and enhancing syngas compatibility with end-use applications.
- Waste Heat Recovery:Waste heat recovery systems capture and utilize heat generated during the gasification process for various purposes, such as preheating biomass feedstocks, generating steam for process heating, or producing electricity through steam turbines or organic Rankine cycles. Efficient utilization of waste heat improves overall energy efficiency, reduces energy costs, and enhances the economic viability of biomass gasification systems.
Optimizing gasification process efficiency requires a holistic approach that integrates feedstock selection, reactor design, operating parameters, syngas cleanup, and waste heat utilization. Continuous research, development, and innovation efforts focus on advancing gasification technologies, enhancing system performance, and reducing environmental impacts to enable the widespread deployment of biomass gasification for renewable energy production and sustainable development.
Gasification Biomass to Energy Conversion:
Gasification biomass to energy conversion refers to the process of converting biomass feedstocks into useful energy products, primarily in the form of syngas (synthesis gas), through thermochemical gasification reactions. This process involves the transformation of organic materials into a combustible gas mixture containing hydrogen (H2), carbon monoxide (CO), carbon dioxide (CO2), methane (CH4), and other trace gases. The syngas produced can be utilized for various energy applications, including power generation, heat production, biofuels synthesis, and chemical manufacturing. Here’s a detailed explanation of gasification biomass to energy conversion:
- Biomass Feedstock Preparation:The biomass feedstock undergoes preprocessing and preparation to optimize its characteristics for gasification. This may include drying to reduce moisture content, size reduction (chipping, shredding) to increase surface area and improve handling, and removal of contaminants and impurities. Proper feedstock preparation ensures efficient conversion and high-quality syngas production.
- Gasification Reactor Operation:The prepared biomass feedstock is fed into a gasification reactor, where it undergoes thermochemical conversion in the presence of a gasification agent (air, oxygen, steam). The gasification reactor operates at elevated temperatures (>700°C) and controlled conditions to promote the partial oxidation and decomposition of biomass into syngas constituents. Various reactor designs, such as fixed-bed, fluidized bed, and entrained-flow gasifiers, are employed to achieve efficient biomass conversion.
- Thermochemical Reactions:Within the gasification reactor, several thermochemical reactions occur sequentially, leading to the production of syngas:
- Drying and Pyrolysis: Moisture present in the biomass is first evaporated and removed, followed by thermal decomposition (pyrolysis) of the biomass into volatile gases, tar, and char in the absence of oxygen.
- Char Gasification: The char residue from pyrolysis reacts with the gasification agent (oxygen, steam) at high temperatures to produce syngas constituents, primarily hydrogen (H2) and carbon monoxide (CO), through partial oxidation and reforming reactions.
- Syngas Cleanup and Conditioning:The raw syngas produced from the gasification reactor contains impurities such as tar, particulate matter, sulfur compounds, and trace metals, which must be removed or reduced to acceptable levels before utilization. Syngas cleanup and conditioning systems, including filtration, scrubbing, catalytic conversion, and cooling, are employed to improve syngas quality, stability, and compatibility with downstream applications.
- Syngas Utilization:Cleaned and conditioned syngas can be utilized in various energy conversion processes and applications, including:
- Power Generation: Syngas can be combusted in gas turbines, reciprocating engines, or fuel cells to generate electricity and heat in combined heat and power (CHP) systems.
- Biofuels Production: Syngas serves as a feedstock for the production of biofuels such as ethanol, methanol, synthetic diesel, and Fischer-Tropsch fuels through catalytic synthesis processes.
- Chemical Synthesis: Syngas is a key precursor for the synthesis of various chemicals and industrial products, including ammonia, methanol, hydrogen, synthetic natural gas (SNG), and higher alcohols.
Gasification biomass to energy conversion offers several advantages over conventional combustion-based systems, including higher energy efficiency, lower emissions, and greater fuel flexibility. It enables the conversion of diverse biomass feedstocks into valuable energy products, contributing to renewable energy deployment, waste reduction, and sustainable development goals. Ongoing research and development efforts focus on advancing gasification technologies, optimizing process efficiency, and expanding the range of feedstocks and applications for biomass-to-energy conversion.
Biomass Gasification Economics:
Biomass gasification offers a promising avenue for renewable energy production, but its economic viability depends on various factors, including feedstock costs, capital investment, operating expenses, energy market prices, and government incentives. Understanding the economics of biomass gasification is crucial for evaluating project feasibility, estimating returns on investment, and informing decision-making processes. Here’s an overview of the key economic aspects of biomass gasification:
- Feedstock Costs:The cost of biomass feedstock constitutes a significant portion of the overall economics of biomass gasification. Feedstock costs depend on factors such as feedstock type, availability, transportation distance, seasonality, and market demand. Low-cost feedstocks, such as forestry residues, agricultural residues, and waste materials, enhance the economic competitiveness of biomass gasification projects.
- Capital Investment:The capital investment required for biomass gasification projects includes costs associated with plant construction, equipment procurement, site preparation, infrastructure development, and engineering and design services. Capital costs vary depending on project scale, technology selection, site-specific conditions, regulatory requirements, and project financing terms. Access to financing options, grants, subsidies, and incentives can mitigate upfront capital investment requirements and improve project economics.
- Operating Expenses:Operating expenses (OPEX) encompass the ongoing costs associated with biomass gasification plant operation, maintenance, labor, utilities, consumables, and feedstock procurement. OPEX also include costs related to syngas cleanup, conditioning, and product distribution. Optimizing operating expenses through efficient plant design, process integration, and maintenance practices is essential for maximizing project profitability and long-term viability.
- Energy Market Prices:Biomass gasification projects generate revenue by selling syngas, electricity, heat, biofuels, or chemical products to energy markets, industrial consumers, utilities, or end-users. Energy market prices for these products are influenced by factors such as commodity prices, supply and demand dynamics, regulatory policies, and market competition. Analyzing energy market prices and demand forecasts is essential for revenue projections and financial modeling of biomass gasification projects.
- Revenue Streams:Biomass gasification projects may generate revenue from multiple streams, including electricity sales, renewable energy credits (RECs), capacity payments, heat sales, biofuels production, chemical sales, carbon credits, and byproduct utilization. Diversifying revenue streams can enhance project resilience to market fluctuations and regulatory changes, mitigate financial risks, and improve overall project economics.
- Financial Metrics:Various financial metrics are used to assess the economic performance and viability of biomass gasification projects, including net present value (NPV), internal rate of return (IRR), payback period, levelized cost of energy (LCOE), return on investment (ROI), and cash flow analysis. These metrics evaluate project economics, profitability, risk-adjusted returns, and sensitivity to key parameters such as feedstock costs, energy prices, and discount rates.
- Policy and Regulatory Support:Government policies, incentives, subsidies, and regulatory frameworks play a crucial role in shaping the economic landscape for biomass gasification projects. Supportive policies, such as renewable energy mandates, tax credits, feed-in tariffs, and grants, can significantly improve the financial attractiveness and competitiveness of biomass gasification investments. Understanding and leveraging policy incentives are essential for maximizing project economics and attracting investment.
Overall, the economics of biomass gasification projects depend on a complex interplay of feedstock availability, technology costs, energy market dynamics, policy support, and project-specific factors. Conducting comprehensive techno-economic assessments, risk analyses, and financial modeling is essential for evaluating project feasibility, identifying investment opportunities, and optimizing the economic performance of biomass gasification ventures.
Biomass Gasification Environmental Impacts:
Biomass gasification offers a renewable and potentially sustainable pathway for energy production; however, it also has environmental implications that need careful consideration. Understanding and mitigating these impacts are crucial for ensuring that biomass gasification contributes positively to overall environmental goals. Here are the key environmental impacts associated with biomass gasification:
- Greenhouse Gas Emissions:Biomass gasification is often considered carbon-neutral because the carbon dioxide (CO2) emitted during gasification is offset by the carbon absorbed by the biomass during its growth. However, incomplete combustion or inefficient gasification processes can result in emissions of greenhouse gases such as methane (CH4) and nitrous oxide (N2O), which have higher global warming potentials than CO2. Minimizing emissions through proper gasification technology selection, operation, and emissions control measures is essential for mitigating climate impacts.
- Air Quality:Biomass gasification can emit air pollutants such as particulate matter (PM), volatile organic compounds (VOCs), nitrogen oxides (NOx), sulfur dioxide (SO2), and carbon monoxide (CO), especially during startup, shutdown, or under suboptimal operating conditions. These pollutants can contribute to local air quality degradation, respiratory health issues, and environmental pollution. Implementing effective emission control technologies, such as electrostatic precipitators, scrubbers, and catalytic converters, can reduce air pollutant emissions from gasification plants.
- Land Use and Biodiversity:Biomass feedstock production for gasification may compete with land use for food crops, natural habitats, and ecosystem services, leading to land conversion, habitat loss, and biodiversity impacts. Sustainable land management practices, including agroforestry, crop rotation, and land restoration, can minimize negative land use impacts and promote ecosystem resilience. Utilizing waste biomass and marginal lands for feedstock production can reduce pressure on agricultural land and mitigate land use conflicts.
- Water Resources:Biomass gasification requires water for feedstock preparation, reactor cooling, syngas cleanup, and wastewater treatment. Water consumption and discharge from gasification plants can affect local water resources, aquatic ecosystems, and water quality. Implementing water conservation measures, recycling wastewater streams, and minimizing chemical usage can reduce the water footprint and environmental impact of biomass gasification operations.
- Solid Waste Management:Biomass gasification produces solid residues, such as ash, char, and tar, which require proper management to prevent environmental contamination and ecosystem disruption. Ash disposal, tar handling, and byproduct utilization should be managed to minimize land, air, and water pollution risks. Recycling ash for soil amendment, utilizing char for carbon sequestration, and converting tar into value-added products can enhance resource efficiency and reduce waste generation.
- Ecological Footprint:Biomass feedstock production, harvesting, transportation, and processing activities contribute to the ecological footprint associated with biomass gasification. Assessing and minimizing the ecological impacts of these activities, such as soil erosion, habitat fragmentation, and invasive species introduction, are essential for ensuring the sustainability of biomass supply chains. Adopting sustainable forestry practices, promoting biodiversity conservation, and enhancing ecosystem services can mitigate ecological footprint impacts.
Addressing the environmental impacts of biomass gasification requires a holistic approach that integrates technology innovation, policy support, stakeholder engagement, and best management practices. Implementing environmental management systems, conducting life cycle assessments, and adhering to regulatory requirements are essential for ensuring the sustainable development of biomass gasification projects. By mitigating environmental risks and maximizing environmental co-benefits, biomass gasification can contribute to a more sustainable and resilient energy future.
Renewable Energy
Renewable energy refers to energy derived from naturally replenished and sustainable sources that are continuously available or can be replenished within a relatively short timeframe. Unlike fossil fuels, which are finite and non-renewable, renewable energy sources offer significant environmental benefits, including lower greenhouse gas emissions, reduced air pollution, and decreased reliance on imported fuels. Here’s a detailed overview of renewable energy:
- Types of Renewable Energy Sources:
- Solar Energy: Solar energy harnesses the power of sunlight using photovoltaic (PV) panels or concentrated solar power (CSP) systems to generate electricity or heat water for residential, commercial, and industrial applications.
- Wind Energy: Wind energy utilizes wind turbines to convert kinetic energy from wind into electricity, with onshore and offshore wind farms being prominent sources of renewable electricity generation.
- Hydropower: Hydropower harnesses the energy of flowing or falling water to generate electricity through hydroelectric dams, run-of-river systems, or pumped storage facilities, providing reliable and dispatchable power.
- Biomass Energy: Biomass energy utilizes organic materials such as wood, agricultural residues, and organic waste to produce heat, electricity, or biofuels through combustion, gasification, or anaerobic digestion processes.
- Geothermal Energy: Geothermal energy taps into heat stored beneath the Earth’s surface to generate electricity or provide direct heating and cooling through geothermal power plants or geothermal heat pumps.
- Environmental Benefits:Renewable energy sources offer several environmental advantages compared to conventional fossil fuels:
- Reduced Greenhouse Gas Emissions: Renewable energy sources produce minimal or no greenhouse gas emissions during electricity generation, helping mitigate climate change and reduce global warming.
- Improved Air Quality: By displacing fossil fuel combustion, renewable energy technologies reduce emissions of air pollutants such as sulfur dioxide (SO2), nitrogen oxides (NOx), particulate matter (PM), and volatile organic compounds (VOCs), leading to cleaner air and healthier communities.
- Conservation of Natural Resources: Renewable energy sources utilize abundant and locally available resources such as sunlight, wind, water, and organic matter, reducing dependence on finite and environmentally damaging fossil fuels.
- Protection of Ecosystems: Unlike conventional energy extraction and production methods, renewable energy projects typically have lower environmental impacts on land, water, and wildlife habitats, preserving biodiversity and ecosystem services.
- Technological Advancements:Rapid advancements in renewable energy technologies have led to cost reductions, efficiency improvements, and widespread deployment:
- Solar Photovoltaics: Advances in PV panel efficiency, manufacturing processes, and installation techniques have made solar energy increasingly competitive with conventional electricity sources, driving significant growth in solar installations worldwide.
- Wind Turbines: Larger, more efficient wind turbines with advanced blade designs and tower configurations have enhanced the performance and cost-effectiveness of wind energy, making it one of the fastest-growing renewable energy sources globally.
- Energy Storage: Innovations in battery storage technologies, such as lithium-ion batteries, flow batteries, and thermal storage systems, enable the integration of intermittent renewable energy sources like solar and wind into the grid, enhancing reliability and grid stability.
- Grid Integration: Smart grid technologies, digital controls, and predictive analytics facilitate the integration of renewable energy resources into the electricity grid, enabling better management of supply-demand dynamics and optimization of energy flows.
- Policy Support and Market Trends:Government policies, incentives, and regulations play a crucial role in driving the adoption of renewable energy:
- Renewable Portfolio Standards (RPS): Many countries and regions have implemented RPS mandates, requiring utilities to procure a certain percentage of their electricity from renewable sources, thereby stimulating demand for renewable energy projects.
- Feed-in Tariffs (FITs): FIT programs offer long-term contracts and guaranteed payments to renewable energy producers, providing revenue certainty and investment incentives for project developers.
- Carbon Pricing: Carbon pricing mechanisms such as carbon taxes or cap-and-trade systems create financial incentives for reducing greenhouse gas emissions and transitioning to low-carbon energy sources, including renewables.
- Market Liberalization: Deregulation of energy markets and competitive procurement processes have enabled renewable energy technologies to compete on a level playing field with conventional fossil fuels, driving down costs and accelerating market penetration.
- Challenges and Future Outlook:Despite significant progress, renewable energy deployment still faces several challenges:
- Intermittency and Variability: The intermittent nature of renewable energy sources such as solar and wind poses challenges for grid integration and energy system reliability, requiring advances in energy storage, grid flexibility, and demand response technologies.
- Infrastructure and Investment: The scale-up of renewable energy infrastructure, including transmission lines, grid interconnections, and storage facilities, requires significant investments and regulatory approvals, which can pose barriers to project development.
- Policy Uncertainty: Shifting political landscapes, changes in government priorities, and evolving regulatory frameworks create uncertainty for renewable energy investors and developers, impacting project economics and market dynamics.
Energy Efficiency:
Energy efficiency refers to the utilization of energy resources in a way that maximizes output while minimizing input, waste, and environmental impacts. It involves the adoption of technologies, practices, and behaviors that reduce energy consumption and optimize energy use across various sectors, including buildings, transportation, industry, and agriculture. Here’s a comprehensive overview of energy efficiency:
- Benefits of Energy Efficiency:
- Cost Savings: Improving energy efficiency reduces energy consumption and lowers energy bills for consumers, businesses, and industries, leading to cost savings and improved economic competitiveness.
- Environmental Benefits: Energy efficiency reduces greenhouse gas emissions, air pollutants, and resource depletion associated with energy production and consumption, mitigating climate change, air pollution, and environmental degradation.
- Energy Security: Enhancing energy efficiency reduces dependence on imported fossil fuels and enhances energy independence, resilience, and security by diversifying energy sources and reducing energy demand.
- Job Creation: Energy efficiency initiatives create jobs in manufacturing, construction, engineering, and service industries, supporting local economies and fostering innovation and entrepreneurship in clean energy sectors.
- Health and Comfort: Energy-efficient buildings, appliances, and transportation systems enhance indoor air quality, thermal comfort, and occupant well-being, reducing health risks associated with indoor pollutants and temperature extremes.
- Key Areas of Energy Efficiency:
- Buildings: Energy-efficient building design, insulation, lighting, HVAC (heating, ventilation, and air conditioning) systems, and appliances reduce energy demand for space heating, cooling, lighting, and appliances, optimizing energy use in residential, commercial, and institutional buildings.
- Transportation: Improving vehicle fuel efficiency, promoting public transportation, adopting alternative fuels, optimizing logistics, and encouraging active transportation modes such as walking and cycling reduce energy consumption and emissions from transportation activities.
- Industry: Industrial energy efficiency measures such as process optimization, equipment upgrades, cogeneration, waste heat recovery, and energy management systems improve productivity, reduce energy intensity, and enhance competitiveness in manufacturing and industrial processes.
- Electricity Generation: Increasing the efficiency of power generation technologies, upgrading transmission and distribution infrastructure, promoting combined heat and power (CHP) systems, and integrating renewable energy sources optimize energy production and delivery, reducing losses and enhancing grid reliability.
- Agriculture: Energy-efficient irrigation systems, precision agriculture technologies, renewable energy adoption, and sustainable farming practices reduce energy inputs, enhance resource efficiency, and promote climate-smart agriculture in rural communities.
- Energy Efficiency Technologies and Practices:
- Energy Management Systems (EMS): EMS platforms monitor, control, and optimize energy consumption in buildings, industrial facilities, and transportation fleets, providing real-time data, analytics, and insights for energy efficiency improvements.
- Energy-Efficient Appliances: ENERGY STAR-rated appliances, LED lighting, high-efficiency HVAC systems, smart thermostats, and energy-efficient motors and pumps reduce energy consumption and operating costs in residential, commercial, and industrial sectors.
- Building Retrofits: Energy retrofit projects incorporate energy-saving measures such as insulation upgrades, window replacements, HVAC system retrofits, and lighting retrofits to improve building energy performance, comfort, and sustainability.
- Demand-Side Management (DSM): DSM programs incentivize energy consumers to reduce peak demand, shift energy usage to off-peak hours, and participate in demand response initiatives, balancing supply and demand and optimizing grid operations.
- Industrial Best Practices: Industrial energy efficiency measures include process optimization, energy audits, heat recovery, cogeneration, variable speed drives, and lean manufacturing practices, reducing energy waste and improving production efficiency.
- Transportation Solutions: Transportation energy efficiency solutions include fuel-efficient vehicles, hybrid and electric vehicles (EVs), public transit, carpooling, ride-sharing, bicycle infrastructure, and urban planning strategies that promote compact, walkable communities.
- Policy and Regulatory Frameworks:
- Energy Efficiency Standards: Mandatory energy efficiency standards and labeling programs establish minimum efficiency requirements for appliances, equipment, vehicles, and buildings, promoting market transformation and consumer awareness.
- Financial Incentives: Financial incentives such as rebates, tax credits, grants, and low-interest loans incentivize energy efficiency investments, overcome financial barriers, and accelerate the adoption of energy-efficient technologies and practices.
- Building Codes and Regulations: Building energy codes, zoning ordinances, and green building certifications mandate energy-efficient design, construction, and renovation practices, ensuring compliance with energy performance requirements and promoting sustainable development.
- Utility Programs: Utility-sponsored energy efficiency programs offer rebates, incentives, and technical assistance to customers for implementing energy-saving measures, reducing energy consumption, and achieving utility demand-side management goals.
- Challenges and Future Directions:
- Market Barriers: Challenges such as upfront costs, split incentives, information asymmetry, and market failures hinder energy efficiency investments and adoption, requiring targeted policies, financial mechanisms, and capacity-building initiatives to overcome barriers.
- Technology Innovation: Continued research, development, and deployment of energy-efficient technologies, materials, and systems are essential for advancing energy efficiency, enhancing performance, and reducing costs across all sectors.
- Behavioral Change: Promoting energy-saving behaviors, raising awareness, and fostering a culture of energy conservation through education, outreach, and social marketing campaigns empower individuals, communities, and organizations to embrace energy efficiency as a societal norm.
- Integrated Approaches: Adopting integrated, systems-level approaches to energy efficiency planning, design, and implementation ensures holistic solutions that optimize energy use, enhance resilience, and maximize co-benefits across economic, social, and environmental dimensions.
Investing in energy efficiency is a cost-effective and scalable strategy for addressing energy security, climate change, and sustainable development challenges. By leveraging technological innovations, policy interventions, and stakeholder engagement, energy efficiency can play a pivotal role in transitioning to a cleaner, more resilient, and sustainable energy future.
Clean Energy:
Clean energy refers to energy sources and technologies that produce minimal or no harmful emissions of greenhouse gases, air pollutants, and other environmental contaminants during energy generation and use. It encompasses renewable energy sources such as solar, wind, hydroelectric, geothermal, and biomass, as well as low-emission technologies including nuclear power and carbon capture and storage (CCS). Here’s a detailed exploration of clean energy:
- Renewable Energy Sources:
- Solar Energy: Solar power harnesses sunlight using photovoltaic (PV) panels or concentrated solar power (CSP) systems to generate electricity and heat water for residential, commercial, and industrial applications. Solar energy is abundant, widely available, and emits no greenhouse gases during operation.
- Wind Energy: Wind turbines convert kinetic energy from wind into electricity, providing a scalable and cost-effective source of renewable power. Onshore and offshore wind farms harness wind resources to generate clean electricity, reduce carbon emissions, and enhance energy security.
- Hydropower: Hydropower utilizes flowing or falling water to generate electricity through hydroelectric dams, run-of-river systems, and pumped storage facilities. Hydropower is a mature and reliable source of renewable energy, providing flexible and dispatchable power to meet electricity demand.
- Geothermal Energy: Geothermal power taps into heat stored beneath the Earth’s surface to produce electricity and provide heating and cooling for buildings. Geothermal power plants harness geothermal reservoirs and geothermal heat pumps utilize shallow ground heat for space conditioning.
- Biomass Energy: Biomass energy utilizes organic materials such as wood, agricultural residues, and organic waste to generate heat, electricity, or biofuels through combustion, gasification, or anaerobic digestion processes. Biomass is a versatile and renewable energy source with potential for carbon neutrality.
- Low-Emission Technologies:
- Nuclear Power: Nuclear energy produces electricity through controlled nuclear reactions, emitting minimal greenhouse gases and air pollutants compared to fossil fuels. Nuclear power plants provide baseload electricity and contribute to energy diversification and decarbonization efforts.
- Carbon Capture and Storage (CCS): CCS technologies capture carbon dioxide (CO2) emissions from industrial facilities and power plants, compressing and transporting CO2 for underground storage in geological formations. CCS mitigates carbon emissions from fossil fuel combustion and industrial processes, enabling cleaner energy production.
- Benefits of Clean Energy:
- Climate Mitigation: Clean energy technologies reduce greenhouse gas emissions, mitigate climate change, and support global efforts to limit global warming to well below 2 degrees Celsius above pre-industrial levels, as outlined in the Paris Agreement.
- Air Quality Improvement: By displacing fossil fuel combustion, clean energy sources reduce emissions of air pollutants such as sulfur dioxide (SO2), nitrogen oxides (NOx), particulate matter (PM), and volatile organic compounds (VOCs), improving air quality and public health.
- Energy Security: Clean energy diversifies energy sources, reduces dependence on imported fossil fuels, and enhances energy resilience and security by promoting indigenous and domestically available energy resources.
- Economic Development: Clean energy investments create jobs, stimulate economic growth, and drive innovation and entrepreneurship in clean energy sectors such as renewable energy, energy efficiency, and advanced technologies.
- Environmental Protection: Clean energy technologies minimize environmental impacts on land, water, and ecosystems, preserving biodiversity, protecting natural resources, and promoting sustainable development.
- Challenges and Opportunities:
- Intermittency and Grid Integration: Intermittent renewable energy sources such as solar and wind require grid integration, energy storage, and demand response solutions to address variability, optimize energy supply-demand balance, and enhance grid reliability and stability.
- Technology Costs and Deployment: While clean energy costs have declined significantly in recent years, upfront capital costs, financing barriers, and policy uncertainties can hinder widespread deployment and adoption of clean energy technologies.
- Policy Support and Market Incentives: Government policies, incentives, and regulatory frameworks play a crucial role in driving clean energy deployment, attracting investment, and stimulating market demand for clean energy technologies.
- Research and Innovation: Continued research, development, and demonstration of clean energy technologies, materials, and systems are essential for advancing technological innovation, improving performance, and reducing costs across all clean energy sectors.
Clean energy is a cornerstone of sustainable development, offering a pathway to address energy-related challenges while safeguarding environmental and public health. By accelerating the transition to clean energy, governments, businesses, and communities can achieve multiple economic, social, and environmental benefits, contributing to a more resilient, equitable, and sustainable energy future
Green Energy:
Green energy, also known as renewable energy or clean energy, refers to energy derived from naturally replenished and environmentally sustainable sources that produce minimal or no harmful emissions during electricity generation or use. Green energy technologies harness natural resources such as sunlight, wind, water, geothermal heat, and biomass to produce electricity, heat, and transportation fuels, offering a cleaner and more sustainable alternative to fossil fuels. Here’s a comprehensive overview of green energy:
- Types of Green Energy Sources:
- Solar Energy: Solar power utilizes photovoltaic (PV) panels or concentrated solar power (CSP) systems to convert sunlight into electricity, heating, and cooling for residential, commercial, and industrial applications. Solar energy is abundant, widely distributed, and emits no greenhouse gases during operation.
- Wind Energy: Wind turbines capture kinetic energy from wind to generate electricity, providing a scalable and cost-effective source of renewable power. Onshore and offshore wind farms harness wind resources to reduce carbon emissions and enhance energy security.
- Hydropower: Hydropower harnesses the energy of flowing or falling water to generate electricity through hydroelectric dams, run-of-river systems, and pumped storage facilities. Hydropower is a mature and reliable source of renewable energy, providing flexible and dispatchable power to meet electricity demand.
- Geothermal Energy: Geothermal power taps into heat stored beneath the Earth’s surface to produce electricity and provide heating and cooling for buildings. Geothermal power plants harness geothermal reservoirs, while geothermal heat pumps utilize shallow ground heat for space conditioning.
- Biomass Energy: Biomass energy utilizes organic materials such as wood, agricultural residues, and organic waste to generate heat, electricity, or biofuels through combustion, gasification, or anaerobic digestion processes. Biomass is a versatile and renewable energy source with potential for carbon neutrality.
- Environmental Benefits of Green Energy:
- Climate Mitigation: Green energy sources reduce greenhouse gas emissions, mitigate climate change, and support global efforts to limit global warming to well below 2 degrees Celsius above pre-industrial levels, as outlined in the Paris Agreement.
- Air Quality Improvement: By displacing fossil fuel combustion, green energy technologies reduce emissions of air pollutants such as sulfur dioxide (SO2), nitrogen oxides (NOx), particulate matter (PM), and volatile organic compounds (VOCs), improving air quality and public health.
- Resource Conservation: Green energy sources utilize abundant and locally available resources such as sunlight, wind, water, and organic matter, reducing dependence on finite and environmentally damaging fossil fuels and promoting sustainable resource management.
- Biodiversity Protection: Unlike conventional energy extraction and production methods, green energy projects typically have lower environmental impacts on land, water, and wildlife habitats, preserving biodiversity and ecosystem services.
- Economic and Social Benefits:
- Job Creation: Green energy investments create jobs in manufacturing, construction, installation, maintenance, and service industries, supporting local economies and fostering innovation and entrepreneurship in clean energy sectors.
- Energy Access: Green energy technologies offer opportunities to expand access to affordable, reliable, and clean energy services for underserved communities, rural areas, and developing countries, enhancing energy equity and social inclusion.
- Energy Independence: Green energy diversifies energy sources, reduces dependence on imported fossil fuels, and enhances energy resilience and security by promoting indigenous and domestically available energy resources.
- Challenges and Opportunities:
- Intermittency and Grid Integration: Intermittent renewable energy sources such as solar and wind require grid integration, energy storage, and demand response solutions to address variability, optimize energy supply-demand balance, and enhance grid reliability and stability.
- Technology Costs and Deployment: While green energy costs have declined significantly in recent years, upfront capital costs, financing barriers, and policy uncertainties can hinder widespread deployment and adoption of green energy technologies.
- Policy Support and Market Incentives: Government policies, incentives, and regulatory frameworks play a crucial role in driving green energy deployment, attracting investment, and stimulating market demand for renewable energy technologies.
- Research and Innovation: Continued research, development, and demonstration of green energy technologies, materials, and systems are essential for advancing technological innovation, improving performance, and reducing costs across all renewable energy sectors.
Green energy is a key enabler of sustainable development, offering a pathway to address energy-related challenges while safeguarding environmental and public health. By accelerating the transition to green energy, governments, businesses, and communities can achieve multiple economic, social, and environmental benefits, contributing to a more resilient, equitable, and sustainable energy future.
Low-Carbon Energy:
Low-carbon energy refers to energy sources and technologies that produce minimal greenhouse gas emissions, particularly carbon dioxide (CO2), during electricity generation or use. By reducing carbon emissions, low-carbon energy plays a crucial role in mitigating climate change and transitioning to a more sustainable and environmentally friendly energy system. Here’s an in-depth exploration of low-carbon energy:
- Types of Low-Carbon Energy Sources:
- Renewable Energy: Renewable energy sources such as solar, wind, hydropower, geothermal, and biomass produce minimal or no greenhouse gas emissions during electricity generation. These clean energy sources offer scalable and environmentally sustainable alternatives to fossil fuels.
- Nuclear Energy: Nuclear power plants generate electricity through controlled nuclear reactions, emitting negligible amounts of CO2 during operation. While nuclear energy does produce radioactive waste and poses safety and security concerns, it is considered a low-carbon energy option.
- Carbon Capture and Storage (CCS): CCS technologies capture CO2 emissions from industrial facilities and power plants, compressing and storing CO2 underground in geological formations to prevent it from entering the atmosphere. CCS enables the continued use of fossil fuels while reducing carbon emissions.
- Environmental Benefits of Low-Carbon Energy:
- Climate Mitigation: Low-carbon energy sources reduce greenhouse gas emissions, mitigate climate change, and support global efforts to limit global warming to well below 2 degrees Celsius above pre-industrial levels, as outlined in the Paris Agreement.
- Air Quality Improvement: By displacing fossil fuel combustion, low-carbon energy technologies reduce emissions of air pollutants such as sulfur dioxide (SO2), nitrogen oxides (NOx), particulate matter (PM), and volatile organic compounds (VOCs), improving air quality and public health.
- Resource Conservation: Low-carbon energy sources utilize abundant and domestically available resources such as sunlight, wind, water, and nuclear fuel, reducing dependence on imported fossil fuels and promoting energy security and resource sustainability.
- Economic and Social Benefits:
- Job Creation: Investments in low-carbon energy projects create jobs in manufacturing, construction, installation, maintenance, and service industries, supporting local economies and fostering innovation and entrepreneurship in clean energy sectors.
- Energy Access: Low-carbon energy technologies offer opportunities to expand access to affordable, reliable, and clean energy services for underserved communities, rural areas, and developing countries, enhancing energy equity and social inclusion.
- Energy Independence: Low-carbon energy diversifies energy sources, reduces dependence on imported fossil fuels, and enhances energy resilience and security by promoting indigenous and domestically available energy resources.
- Challenges and Opportunities:
- Cost Competitiveness: While low-carbon energy technologies have become increasingly cost-competitive with conventional fossil fuels, upfront capital costs, financing barriers, and policy uncertainties can hinder widespread deployment and adoption.
- Grid Integration: Intermittent renewable energy sources such as solar and wind require grid integration, energy storage, and demand response solutions to address variability, optimize energy supply-demand balance, and enhance grid reliability and stability.
- Policy Support and Market Incentives: Government policies, incentives, and regulatory frameworks play a crucial role in driving low-carbon energy deployment, attracting investment, and stimulating market demand for clean energy technologies.
- Research and Innovation: Continued research, development, and demonstration of low-carbon energy technologies, materials, and systems are essential for advancing technological innovation, improving performance, and reducing costs across all clean energy sectors.
Low-carbon energy is a cornerstone of sustainable development, offering a pathway to address energy-related challenges while safeguarding environmental and public health. By accelerating the transition to low-carbon energy, governments, businesses, and communities can achieve multiple economic, social, and environmental benefits, contributing to a more resilient, equitable, and sustainable energy future.
Renewable Energy:
Renewable energy refers to energy derived from naturally replenished sources that are continuously available and environmentally sustainable over the long term. Unlike fossil fuels, which are finite and contribute to environmental degradation and climate change, renewable energy sources harness natural processes such as sunlight, wind, water, geothermal heat, and biomass to generate electricity, heat, and transportation fuels. Here’s a detailed exploration of renewable energy:
- Types of Renewable Energy Sources:
- Solar Energy: Solar power utilizes photovoltaic (PV) panels or concentrated solar power (CSP) systems to convert sunlight into electricity and heat water for residential, commercial, and industrial applications. Solar energy is abundant, widely available, and emits no greenhouse gases during operation.
- Wind Energy: Wind turbines capture kinetic energy from wind to generate electricity, providing a scalable and cost-effective source of renewable power. Onshore and offshore wind farms harness wind resources to reduce carbon emissions and enhance energy security.
- Hydropower: Hydropower harnesses the energy of flowing or falling water to generate electricity through hydroelectric dams, run-of-river systems, and pumped storage facilities. Hydropower is a mature and reliable source of renewable energy, providing flexible and dispatchable power to meet electricity demand.
- Geothermal Energy: Geothermal power taps into heat stored beneath the Earth’s surface to produce electricity and provide heating and cooling for buildings. Geothermal power plants harness geothermal reservoirs, while geothermal heat pumps utilize shallow ground heat for space conditioning.
- Biomass Energy: Biomass energy utilizes organic materials such as wood, agricultural residues, and organic waste to generate heat, electricity, or biofuels through combustion, gasification, or anaerobic digestion processes. Biomass is a versatile and renewable energy source with potential for carbon neutrality.
- Environmental Benefits of Renewable Energy:
- Climate Mitigation: Renewable energy sources reduce greenhouse gas emissions, mitigate climate change, and support global efforts to limit global warming to well below 2 degrees Celsius above pre-industrial levels, as outlined in the Paris Agreement.
- Air Quality Improvement: By displacing fossil fuel combustion, renewable energy technologies reduce emissions of air pollutants such as sulfur dioxide (SO2), nitrogen oxides (NOx), particulate matter (PM), and volatile organic compounds (VOCs), improving air quality and public health.
- Resource Conservation: Renewable energy sources utilize abundant and domestically available resources such as sunlight, wind, water, and organic matter, reducing dependence on imported fossil fuels and promoting energy security and resource sustainability.
- Economic and Social Benefits:
- Job Creation: Investments in renewable energy projects create jobs in manufacturing, construction, installation, maintenance, and service industries, supporting local economies and fostering innovation and entrepreneurship in clean energy sectors.
- Energy Access: Renewable energy technologies offer opportunities to expand access to affordable, reliable, and clean energy services for underserved communities, rural areas, and developing countries, enhancing energy equity and social inclusion.
- Energy Independence: Renewable energy diversifies energy sources, reduces dependence on imported fossil fuels, and enhances energy resilience and security by promoting indigenous and domestically available energy resources.
- Challenges and Opportunities:
- Intermittency and Grid Integration: Intermittent renewable energy sources such as solar and wind require grid integration, energy storage, and demand response solutions to address variability, optimize energy supply-demand balance, and enhance grid reliability and stability.
- Technology Costs and Deployment: While renewable energy costs have declined significantly in recent years, upfront capital costs, financing barriers, and policy uncertainties can hinder widespread deployment and adoption of renewable energy technologies.
- Policy Support and Market Incentives: Government policies, incentives, and regulatory frameworks play a crucial role in driving renewable energy deployment, attracting investment, and stimulating market demand for clean energy technologies.
- Research and Innovation: Continued research, development, and demonstration of renewable energy technologies, materials, and systems are essential for advancing technological innovation, improving performance, and reducing costs across all renewable energy sectors.
Renewable energy is a key enabler of sustainable development, offering a pathway to address energy-related challenges while safeguarding environmental and public health. By accelerating the transition to renewable energy, governments, businesses, and communities can achieve multiple economic, social, and environmental benefits, contributing to a more resilient, equitable, and sustainable energy future.
Solar Energy:
Solar energy is the radiant light and heat from the Sun that is harnessed using various technologies to generate electricity, provide heating and cooling, and power various applications. It is one of the most abundant and widely available renewable energy sources, offering a clean and sustainable alternative to fossil fuels. Here’s a comprehensive overview of solar energy:
- Photovoltaic (PV) Technology:
- Photovoltaic technology converts sunlight directly into electricity using semiconductor materials such as silicon. When sunlight strikes the PV cells, electrons are freed, creating an electric current. PV cells are combined to form solar panels, which can be installed on rooftops, ground-mounted systems, and solar farms.
- Solar panels are available in different types, including monocrystalline, polycrystalline, and thin-film, each offering unique characteristics in terms of efficiency, durability, and cost.
- Concentrated Solar Power (CSP) Systems:
- Concentrated solar power systems use mirrors or lenses to concentrate sunlight onto a small area, typically a solar receiver or heat exchanger. The concentrated sunlight is used to generate high-temperature heat, which drives a conventional steam turbine or engine to produce electricity.
- CSP systems can incorporate various technologies such as parabolic troughs, power towers, and dish/engine systems, each with its advantages and applications.
- Solar Heating and Cooling:
- Solar thermal technologies harness solar energy to provide heating and cooling for residential, commercial, and industrial applications. Solar water heaters use sunlight to heat water for domestic use, space heating, and swimming pools, reducing energy consumption and costs.
- Solar air conditioning systems utilize solar collectors to absorb heat from the air and convert it into chilled water or cool air for cooling buildings, improving indoor comfort and energy efficiency.
- Off-Grid and Grid-Connected Systems:
- Off-grid solar systems, also known as standalone or remote systems, are not connected to the electricity grid and rely on batteries or other energy storage technologies to store excess energy for use during periods of low sunlight. They are commonly used in remote areas where grid electricity is unavailable or unreliable.
- Grid-connected solar systems, also known as grid-tied or grid-interconnected systems, are connected to the electricity grid, allowing surplus energy to be exported to the grid when production exceeds demand and imported when demand exceeds production. Grid-connected systems often benefit from net metering programs, where users receive credits for excess electricity fed into the grid.
- Environmental Benefits of Solar Energy:
- Solar energy is a clean and renewable energy source that produces no greenhouse gas emissions or air pollutants during operation, reducing environmental impact and mitigating climate change.
- By displacing fossil fuel-based electricity generation, solar energy helps reduce dependence on finite and environmentally damaging resources such as coal, oil, and natural gas, promoting sustainable resource management and environmental conservation.
- Economic and Social Benefits:
- Solar energy investments create jobs in manufacturing, installation, maintenance, and service industries, supporting local economies and driving economic growth and development.
- Solar energy offers opportunities for energy independence and resilience by diversifying energy sources, reducing reliance on imported fossil fuels, and enhancing energy security and stability.
- Challenges and Opportunities:
- Despite significant cost reductions and technological advancements, solar energy still faces challenges such as intermittency, energy storage, and grid integration, requiring continued research, innovation, and investment to address.
- Policy support, financial incentives, and regulatory frameworks play a crucial role in driving solar energy deployment, attracting investment, and stimulating market demand for solar technologies.
- Collaboration between governments, businesses, academia, and civil society is essential to overcome barriers, unlock opportunities, and accelerate the transition to a solar-powered future that is sustainable, equitable, and resilient.
Solar energy is a key pillar of the global energy transition, offering a reliable, sustainable, and cost-effective solution to meet growing energy demand while mitigating climate change and promoting environmental stewardship. By harnessing the power of the Sun, we can create a brighter and more sustainable future for generations to come.
Wind Energy:
Wind energy is the conversion of wind power into a useful form of energy, such as electricity, through the use of wind turbines. It is a renewable and sustainable energy source that harnesses the kinetic energy of moving air masses to generate electricity. Here’s a detailed exploration of wind energy:
- Wind Turbine Technology:
- Wind turbines consist of rotor blades attached to a hub, which is mounted on a tall tower. As the wind blows, it causes the rotor blades to rotate, driving a generator to produce electricity. Modern wind turbines can vary in size, from small turbines for residential or community use to large utility-scale turbines used in wind farms.
- Horizontal-axis wind turbines (HAWTs) are the most common type of wind turbine, with the rotor shaft oriented horizontally to the ground. Vertical-axis wind turbines (VAWTs) have their rotor shaft positioned vertically, offering advantages such as easier maintenance and lower wind speed requirements.
- Onshore and Offshore Wind Farms:
- Onshore wind farms are located on land and typically consist of multiple wind turbines installed in suitable windy areas such as plains, hills, or coastal regions. Onshore wind farms can vary in size and capacity, ranging from small community projects to large utility-scale installations.
- Offshore wind farms are located in bodies of water, such as oceans or large lakes, and often feature larger turbines and higher wind speeds compared to onshore sites. Offshore wind farms can be installed near the coastline or further offshore, utilizing fixed-bottom or floating turbine technology.
- Environmental Benefits of Wind Energy:
- Wind energy is a clean and renewable energy source that produces no greenhouse gas emissions or air pollutants during operation, reducing environmental impact and mitigating climate change.
- By displacing fossil fuel-based electricity generation, wind energy helps reduce dependence on finite and environmentally damaging resources such as coal, oil, and natural gas, promoting sustainable resource management and environmental conservation.
- Wind energy projects can coexist with agricultural and recreational land uses, providing additional income to landowners through land lease agreements and contributing to local economic development and job creation in rural communities.
- Economic and Social Benefits:
- Wind energy investments create jobs in manufacturing, construction, installation, maintenance, and service industries, supporting local economies and driving economic growth and development.
- Wind energy offers opportunities for energy independence and resilience by diversifying energy sources, reducing reliance on imported fossil fuels, and enhancing energy security and stability.
- Wind energy projects can provide additional revenue streams to landowners, local governments, and communities through lease payments, tax revenues, and community benefit funds, supporting infrastructure development, public services, and quality of life improvements.
- Challenges and Opportunities:
- Despite significant cost reductions and technological advancements, wind energy still faces challenges such as intermittency, grid integration, and land use conflicts, requiring continued research, innovation, and investment to address.
- Policy support, financial incentives, and regulatory frameworks play a crucial role in driving wind energy deployment, attracting investment, and stimulating market demand for wind technologies.
- Collaboration between governments, businesses, academia, and civil society is essential to overcome barriers, unlock opportunities, and accelerate the transition to a wind-powered future that is sustainable, equitable, and resilient.
Wind energy is a key component of the global energy transition, offering a reliable, sustainable, and cost-effective solution to meet growing energy demand while mitigating climate change and promoting environmental stewardship. By harnessing the power of the wind, we can create a cleaner, greener, and more sustainable energy future for generations to come.
Hydropower:
Hydropower, also known as hydroelectric power, is the generation of electricity from flowing or falling water. It is one of the oldest and most widely used renewable energy sources, providing clean, reliable, and sustainable electricity generation across the globe. Here’s a comprehensive overview of hydropower:
- Types of Hydropower Systems:
- Conventional Hydropower: Conventional hydropower systems utilize dams and reservoirs to store water, which is released through turbines to generate electricity. This technology is commonly used in large-scale hydroelectric dams, which can provide baseload or peaking power to meet electricity demand.
- Run-of-River Hydropower: Run-of-river hydropower systems do not require large reservoirs and instead use the natural flow of rivers to generate electricity. These systems typically involve diverting a portion of the river flow through a turbine, with minimal impact on river ecosystems and aquatic habitats.
- Pumped Storage Hydropower: Pumped storage hydropower facilities store excess electricity by pumping water from a lower reservoir to a higher reservoir during periods of low demand. When electricity demand is high, water is released from the upper reservoir to the lower reservoir through turbines, generating electricity.
- Environmental Benefits of Hydropower:
- Low Greenhouse Gas Emissions: Hydropower is a low-carbon energy source that produces minimal greenhouse gas emissions during operation, making it an important tool for mitigating climate change and reducing reliance on fossil fuels.
- Fish Habitat Restoration: Some hydropower projects incorporate fish passage facilities and habitat restoration measures to mitigate the impacts on fish migration and spawning habitats, promoting biodiversity conservation and ecosystem resilience.
- Flood Control and Water Management: Hydropower dams and reservoirs can provide flood control benefits by regulating river flows and storing excess water during periods of high precipitation, reducing the risk of flooding downstream and supporting water management and irrigation purposes.
- Social and Economic Benefits:
- Job Creation and Economic Development: Hydropower projects create jobs in construction, operation, maintenance, and related industries, supporting local economies and driving economic growth and development in rural and remote areas.
- Energy Security and Reliability: Hydropower provides reliable and dispatchable electricity generation, helping to stabilize grid operation and enhance energy security by complementing intermittent renewable energy sources such as solar and wind.
- Water Supply and Recreation: Hydropower reservoirs can serve multiple purposes, including water supply for drinking, irrigation, and industrial use, as well as recreational activities such as boating, fishing, and tourism, contributing to quality of life and community well-being.
- Challenges and Opportunities:
- Environmental Impacts: Large-scale hydropower projects can have significant environmental impacts, including habitat disruption, alteration of river ecosystems, and displacement of communities. Sustainable hydropower development requires careful planning, stakeholder engagement, and environmental impact assessments to minimize adverse effects and maximize benefits.
- Climate Change Resilience: Climate change poses challenges to hydropower operations, including changes in precipitation patterns, river flows, and reservoir levels. Adaptation measures such as improved water management, infrastructure upgrades, and flexible operation strategies are essential to enhance resilience and mitigate climate risks.
- Social Acceptance and Indigenous Rights: Hydropower projects often face opposition from local communities, indigenous peoples, and environmental advocates due to concerns about land tenure, cultural heritage, and socio-economic impacts. Meaningful consultation, consent, and benefit-sharing mechanisms are critical to address social conflicts and uphold human rights principles.
Hydropower remains a valuable and versatile renewable energy resource, offering a range of environmental, social, and economic benefits. By adopting sustainable practices, investing in modern technologies, and promoting inclusive decision-making processes, hydropower can play a vital role in the transition to a cleaner, greener, and more resilient energy future.
Geothermal Energy:
Geothermal energy is a renewable energy source derived from heat stored beneath the Earth’s surface. It harnesses the natural heat emanating from the Earth’s core, magma, and radioactive decay of minerals to generate electricity, provide heating and cooling, and power various industrial processes. Here’s an in-depth exploration of geothermal energy:
- Geothermal Resources:
- Hydrothermal Resources: Hydrothermal systems consist of hot water and steam reservoirs located near active volcanic regions or tectonic plate boundaries. These reservoirs can be tapped through production wells, with the extracted fluids used directly for heating or converted into electricity through steam turbines.
- Enhanced Geothermal Systems (EGS): EGS utilize hydraulic fracturing and other stimulation techniques to create artificial geothermal reservoirs in hot, dry rock formations located deeper underground. EGS can potentially expand the geographic availability of geothermal energy and increase resource extraction rates.
- Geothermal Heat Pumps (GHP): Geothermal heat pumps utilize the stable temperature of the Earth’s subsurface to provide space heating, cooling, and hot water for residential, commercial, and institutional buildings. GHP systems circulate a fluid through underground pipes to transfer heat to or from the ground, offering energy-efficient climate control solutions.
- Electricity Generation:
- Binary Cycle Power Plants: Binary cycle power plants use geothermal hot water to heat a secondary fluid with a lower boiling point, such as isobutane or pentane. The vaporized secondary fluid drives a turbine-generator unit to produce electricity, while the condensed fluid is recirculated in a closed-loop system.
- Flash Steam Power Plants: Flash steam power plants utilize high-pressure geothermal reservoirs with temperatures exceeding 150°C (300°F). The hot water from the reservoir is released into a flash tank at reduced pressure, causing some of the water to instantly vaporize into steam. The steam is then used to drive a turbine-generator unit, with the remaining water reinjected into the reservoir.
- Dry Steam Power Plants: Dry steam power plants are the simplest and oldest form of geothermal power generation, relying on natural steam from the geothermal reservoir to drive a turbine-generator unit directly. Dry steam power plants are typically located near volcanic geothermal fields with abundant steam resources.
- Environmental Benefits of Geothermal Energy:
- Low Carbon Emissions: Geothermal energy is a low-carbon energy source that produces minimal greenhouse gas emissions during operation, making it an environmentally friendly alternative to fossil fuels for electricity generation and heating.
- Land Use Efficiency: Geothermal power plants have a small footprint compared to fossil fuel-based power plants, requiring relatively little land for infrastructure and resource extraction. Geothermal reservoirs can also support co-located land uses such as agriculture, conservation, and recreation.
- Baseload Power Generation: Geothermal power plants provide reliable and dispatchable electricity generation, capable of operating continuously at high capacity factors regardless of weather conditions or time of day. This baseload power capability enhances grid stability and complements intermittent renewable energy sources such as solar and wind.
- Economic and Social Benefits:
- Job Creation and Economic Development: Geothermal energy projects create jobs in exploration, drilling, construction, operation, maintenance, and service industries, supporting local economies and driving economic growth and development in geothermal-rich regions.
- Energy Access and Affordability: Geothermal heating systems and power plants offer opportunities to expand access to affordable, reliable, and clean energy services for underserved communities, rural areas, and developing countries, improving energy equity and social inclusion.
- Resource Independence and Energy Security: Geothermal energy diversifies energy sources, reduces dependence on imported fossil fuels, and enhances energy resilience and security by utilizing indigenous and domestically available geothermal resources.
- Challenges and Opportunities:
- Resource Exploration and Assessment: Geothermal resource exploration and assessment require significant upfront investment in geological surveys, drilling, and reservoir modeling to identify and characterize suitable sites for development.
- Technology Development and Innovation: Continued research, development, and demonstration of advanced drilling techniques, reservoir engineering methods, and heat extraction technologies are essential to improve geothermal resource utilization, increase energy extraction efficiency, and reduce costs.
- Regulatory Frameworks and Market Incentives: Government policies, regulatory frameworks, and financial incentives play a crucial role in driving geothermal energy deployment, attracting investment, and stimulating market demand for geothermal technologies.
- Stakeholder Engagement and Community Consultation: Meaningful consultation, engagement, and benefit-sharing mechanisms with local communities, indigenous peoples, and other stakeholders are critical to address social, cultural, and environmental concerns, uphold human rights, and ensure sustainable geothermal development.
Geothermal energy offers a reliable, renewable, and environmentally sustainable energy solution with significant potential to mitigate climate change, enhance energy security, and foster economic development. By harnessing the Earth’s natural heat, we can create a cleaner, greener, and more resilient energy future for generations to come.
Biomass Energy:
Biomass energy is derived from organic materials such as wood, agricultural residues, organic waste, and dedicated energy crops. It is a renewable and sustainable energy source that can be used to generate heat, electricity, and biofuels through various conversion processes. Here’s an in-depth exploration of biomass energy:
- Types of Biomass Feedstocks:
- Woody Biomass: Woody biomass includes trees, branches, bark, and wood residues from forestry operations, sawmills, and wood processing industries. It can be sourced from forest thinning, logging residues, urban wood waste, and dedicated energy plantations.
- Agricultural Biomass: Agricultural residues such as crop residues (e.g., corn stover, wheat straw), sugarcane bagasse, rice husks, and animal manure are abundant sources of biomass energy. These residues are generated during agricultural activities such as harvesting, processing, and livestock farming.
- Organic Waste: Organic waste materials such as food waste, yard waste, municipal solid waste (MSW), and wastewater sludge can be converted into biogas through anaerobic digestion or composted to produce compost for soil amendment and fertilizer.
- Energy Crops: Dedicated energy crops such as switchgrass, miscanthus, willow, and poplar are grown specifically for biomass energy production. These fast-growing, high-yielding crops can be cultivated on marginal lands and offer potential for sustainable bioenergy feedstock production.
- Biomass Conversion Technologies:
- Combustion: Biomass combustion involves burning organic materials in boilers or furnaces to produce heat for space heating, water heating, industrial processes, and electricity generation through steam turbines or organic Rankine cycle (ORC) systems.
- Gasification: Biomass gasification converts solid biomass into synthesis gas (syngas) by heating it in a low-oxygen environment. The syngas can be used directly as a fuel for heat and power generation, or further processed into biofuels such as hydrogen, methane, or liquid biofuels.
- Anaerobic Digestion: Anaerobic digestion (AD) breaks down organic matter in the absence of oxygen, producing biogas (a mixture of methane and carbon dioxide) and digestate. Biogas can be used as a renewable fuel for combined heat and power (CHP) generation, vehicle fuel, or upgraded to biomethane for injection into the natural gas grid.
- Pyrolysis: Biomass pyrolysis involves heating organic materials in the absence of oxygen to produce biochar, bio-oil, and syngas. Biochar can be used as a soil amendment to improve soil fertility and carbon sequestration, while bio-oil can be further refined into liquid biofuels or specialty chemicals.
- Environmental Benefits of Biomass Energy:
- Carbon Neutrality: Biomass energy is considered carbon-neutral since the carbon dioxide (CO2) released during combustion or decomposition is offset by the CO2 absorbed during the growth of biomass feedstocks. This closed carbon cycle helps mitigate climate change and reduce net greenhouse gas emissions.
- Waste Reduction and Recycling: Biomass energy provides an environmentally sustainable solution for managing organic waste materials, diverting them from landfills and incinerators while generating valuable renewable energy and bio-based products.
- Resource Efficiency: Biomass energy utilizes locally available and renewable biomass feedstocks, reducing dependence on imported fossil fuels and promoting resource efficiency, circular economy principles, and sustainable biomass management practices.
- Economic and Social Benefits:
- Rural Development: Biomass energy projects create jobs in biomass production, harvesting, transportation, processing, and energy generation, supporting rural economies and revitalizing agricultural and forestry sectors.
- Energy Access and Affordability: Biomass energy provides decentralized and off-grid energy solutions for remote communities, developing countries, and regions with limited access to modern energy services, improving energy equity and social inclusion.
- Bioeconomy Development: Biomass energy contributes to the emergence of a bioeconomy based on renewable resources, bio-based products, and bioenergy technologies, fostering innovation, entrepreneurship, and sustainable development in bioenergy sectors.
- Challenges and Opportunities:
- Sustainability and Environmental Impacts: Sustainable biomass production and harvesting practices are essential to prevent deforestation, land degradation, biodiversity loss, and negative environmental impacts associated with intensive biomass extraction.
- Technology Efficiency and Scale-Up: Biomass conversion technologies require continuous improvements in efficiency, reliability, and scalability to compete with conventional energy sources and meet growing energy demand sustainably.
- Policy Support and Market Incentives: Government policies, incentives, and regulatory frameworks play a crucial role in driving biomass energy deployment, attracting investment, and stimulating market demand for bioenergy technologies and products.
- Community Engagement and Stakeholder Collaboration: Meaningful consultation, engagement, and partnership with local communities, indigenous peoples, and other stakeholders are essential to address social, cultural, and environmental concerns, uphold human rights, and ensure sustainable biomass energy development.
Biomass energy offers a versatile, renewable, and environmentally sustainable energy solution with significant potential to mitigate climate change, promote rural development, and foster a more resilient and inclusive energy future. By harnessing the energy stored in organic materials, we can create a cleaner, greener, and more sustainable world for future generations.
Biofuels:
Biofuels are liquid, gaseous, or solid fuels derived from biomass resources such as plants, algae, and organic waste. They are considered renewable and sustainable alternatives to fossil fuels for transportation, heating, and industrial applications. Here’s a comprehensive exploration of biofuels:
- Types of Biofuels:
- First-Generation Biofuels: First-generation biofuels are produced from food crops, vegetable oils, and animal fats through conventional conversion processes such as fermentation, transesterification, and esterification. Common first-generation biofuels include ethanol, biodiesel, and vegetable oil.
- Second-Generation Biofuels: Second-generation biofuels are produced from non-food biomass feedstocks such as agricultural residues, forestry residues, energy crops, and organic waste. They utilize advanced conversion technologies such as biochemical or thermochemical processes to extract energy-rich components such as cellulose, hemicellulose, and lignin.
- Third-Generation Biofuels: Third-generation biofuels are produced from algae, microorganisms, or aquatic plants cultivated in ponds, bioreactors, or open ocean systems. They offer potential advantages such as high productivity, low land use, and efficient nutrient recycling compared to terrestrial biomass feedstocks.
- Advanced Biofuels: Advanced biofuels encompass a diverse range of bio-based fuels derived from lignocellulosic biomass, waste feedstocks, or microbial conversion processes. They include cellulosic ethanol, renewable diesel, biobutanol, renewable jet fuel, and biomethane, among others.
- Biofuel Production Processes:
- Fermentation: Fermentation processes convert sugars or starches from biomass feedstocks into ethanol or other biofuels using microorganisms such as yeast or bacteria. Feedstocks such as sugarcane, corn, and sugar beets are commonly used for ethanol production, while lignocellulosic biomass can be enzymatically hydrolyzed into fermentable sugars.
- Transesterification: Transesterification processes convert vegetable oils, animal fats, or recycled cooking oils into biodiesel by reacting them with alcohol (e.g., methanol or ethanol) and a catalyst (e.g., sodium hydroxide or potassium hydroxide). Biodiesel can be blended with diesel fuel or used as a pure fuel (B100) in diesel engines.
- Pyrolysis: Pyrolysis processes thermally decompose biomass feedstocks into bio-oil, syngas, and biochar in the absence of oxygen. Bio-oil can be further upgraded into renewable fuels such as gasoline, diesel, and jet fuel through hydrotreating or catalytic cracking processes.
- Hydrothermal Liquefaction (HTL): Hydrothermal liquefaction processes convert wet biomass feedstocks such as algae, sewage sludge, or organic waste into biocrude oil under high temperature and pressure conditions. The biocrude oil can be refined into transportation fuels or specialty chemicals.
- Environmental Benefits of Biofuels:
- Greenhouse Gas Reduction: Biofuels offer significant greenhouse gas emission reductions compared to fossil fuels, as the carbon dioxide (CO2) released during combustion is offset by the CO2 absorbed during biomass growth. Advanced biofuels from non-food feedstocks can achieve even greater emission reductions.
- Air Quality Improvement: Biofuels produce fewer air pollutants such as sulfur dioxide (SO2), nitrogen oxides (NOx), particulate matter (PM), and volatile organic compounds (VOCs) compared to conventional petroleum fuels, contributing to improved air quality and public health.
- Renewable Resource Utilization: Biofuels utilize renewable biomass resources such as agricultural residues, forestry residues, and organic waste, reducing dependence on finite and environmentally damaging fossil fuels and promoting sustainable resource management practices.
- Economic and Social Benefits:
- Energy Security and Diversification: Biofuels diversify the energy mix, reduce dependence on imported fossil fuels, and enhance energy security by utilizing locally available and domestically produced biomass resources.
- Rural Development: Biofuel production creates jobs in farming, forestry, transportation, processing, and distribution sectors, supporting rural economies, revitalizing agricultural communities, and reducing rural poverty.
- Technological Innovation and Industry Growth: Biofuels stimulate innovation, research, and development in bioenergy technologies, biorefining processes, and feedstock production systems, fostering a dynamic and competitive biofuels industry.
- Challenges and Opportunities:
- Feedstock Availability and Sustainability: Sustainable biomass supply chains require careful management of feedstock production, harvesting, transportation, and processing to prevent environmental degradation, land use conflicts, and competition with food production.
- Land Use and Biodiversity Impacts: Biofuel expansion can lead to land use change, deforestation, habitat loss, and biodiversity decline, particularly if it involves the conversion of natural ecosystems or high-conservation-value areas.
- Technology Advancement and Cost Reduction: Continued research, development, and deployment of advanced biofuel technologies are essential to improve conversion efficiency, reduce production costs, and enhance the competitiveness of bio
Biogas:
Biogas is a renewable energy source produced through the anaerobic digestion of organic matter such as agricultural residues, food waste, sewage sludge, and animal manure. It consists primarily of methane (CH4) and carbon dioxide (CO2), with small amounts of other gases such as hydrogen sulfide (H2S), nitrogen (N2), and trace impurities. Here’s a comprehensive overview of biogas:
- Anaerobic Digestion Process:
- Feedstock Preparation: Organic feedstocks are collected, sorted, and shredded to increase surface area and facilitate microbial digestion. Common feedstocks include crop residues, livestock manure, food waste, and wastewater sludge.
- Digestion: The shredded feedstock is loaded into anaerobic digesters, which are sealed, oxygen-free vessels or lagoons where microbial consortia break down organic matter through biochemical reactions. Anaerobic bacteria metabolize complex organic compounds into biogas and digestate.
- Biogas Production: Anaerobic digestion produces biogas, a mixture of methane (50-70%), carbon dioxide (30-50%), and trace gases. Methane is the primary component of biogas and serves as a renewable fuel for heat and power generation, vehicle fuel, or upgraded to biomethane for injection into the natural gas grid.
- Digestate Management: The residual material left after anaerobic digestion, known as digestate, is rich in nutrients and organic matter and can be used as a soil conditioner, fertilizer, or livestock bedding. Digestate may undergo further treatment such as dewatering, composting, or land application to enhance its agronomic value.
- Biogas Utilization Technologies:
- Combined Heat and Power (CHP): Biogas can be used as a fuel for combined heat and power (CHP) systems, also known as cogeneration units, to generate electricity and heat simultaneously. CHP systems are commonly used in agricultural, industrial, and municipal facilities to meet on-site energy needs.
- Biogas Upgrading: Biogas can be upgraded to biomethane through purification processes such as pressure swing adsorption (PSA), water scrubbing, or membrane separation to remove impurities such as CO2, H2S, and moisture. Biomethane can be injected into the natural gas grid or used as a vehicle fuel (compressed natural gas – CNG or liquefied natural gas – LNG).
- Direct Combustion: Biogas can be combusted directly in boilers, furnaces, or gas turbines to produce heat for space heating, water heating, industrial processes, or district heating systems. Direct combustion is a simple and cost-effective method of utilizing biogas for thermal energy applications.
- Biochemical Conversion: Biogas can be converted into liquid biofuels such as biomethanol, bioethanol, or biodiesel through biochemical processes such as fermentation, catalytic conversion, or microbial electrolysis. These biofuels can be used as transportation fuels or blended with conventional fuels to reduce emissions and dependency on fossil fuels.
- Environmental Benefits of Biogas:
- Greenhouse Gas Reduction: Biogas production reduces methane emissions from organic waste decomposition, as methane is captured and utilized as a renewable energy source. By displacing fossil fuels, biogas also helps mitigate climate change and reduce net greenhouse gas emissions.
- Waste Management and Pollution Prevention: Anaerobic digestion of organic waste materials reduces odor emissions, pathogens, and air and water pollution associated with landfilling or open-air decomposition. Biogas production diverts organic waste from landfills, mitigating environmental risks and improving waste management practices.
- Nutrient Recycling and Soil Health: Digestate from anaerobic digestion is a valuable source of organic matter, nitrogen, phosphorus, and micronutrients that can improve soil fertility, structure, and water retention capacity. Land application of digestate reduces the need for synthetic fertilizers and promotes sustainable agriculture practices.
- Economic and Social Benefits:
- Rural Development: Biogas projects create jobs in feedstock collection, digester operation, maintenance, and biogas utilization, supporting rural economies, revitalizing agricultural communities, and reducing dependency on imported energy sources.
- Energy Access and Affordability: Biogas provides decentralized and off-grid energy solutions for rural households, communities, and small-scale enterprises, improving energy access, reducing energy poverty, and enhancing quality of life.
- Waste Valorization and Circular Economy: Biogas production transforms organic waste into a valuable renewable energy resource, promoting circular economy principles, resource efficiency, and waste valorization. Biogas projects contribute to sustainable development goals by addressing environmental, social, and economic challenges.
- Challenges and Opportunities:
- Feedstock Availability and Quality: Biogas production relies on a steady supply of organic feedstocks with consistent quality, moisture content, and nutrient composition. Ensuring feedstock availability and diversity is essential to sustain biogas production and optimize digester performance.
- Technology Efficiency and Reliability: Anaerobic digestion technologies require continuous monitoring, maintenance, and optimization to maximize biogas yield, minimize process downtime, and ensure stable digester operation. Advances in digester design, materials, and control systems can improve efficiency and reliability.
- Policy Support and Market Incentives: Government policies, regulations, and financial incentives play a crucial role in promoting biogas deployment, stimulating investment, and creating favorable market conditions for biogas projects. Supportive policies may include feed-in tariffs, renewable energy mandates, tax incentives, and carbon pricing mechanisms.
- Community Engagement and Stakeholder Collaboration: Meaningful consultation, engagement, and partnership with local communities, farmers, waste management operators, and other stakeholders are essential to address social, cultural, and environmental concerns, build trust, and ensure the success of biogas projects.
Biogas offers a sustainable and renewable energy solution for waste management, greenhouse gas mitigation, rural development, and energy access. By harnessing the energy potential of organic waste materials, we can create cleaner, greener, and more resilient communities while advancing towards a circular bioeconomy.
Renewable Energy Policies:
Renewable energy policies encompass a range of government measures, regulations, incentives, and targets aimed at promoting the development, deployment, and integration of renewable energy sources into the energy mix. These policies are designed to accelerate the transition to a low-carbon economy, mitigate climate change, enhance energy security, and stimulate economic growth. Here’s an in-depth exploration of renewable energy policies:
- Feed-in Tariffs (FiTs):
- Overview: Feed-in tariffs are regulatory mechanisms that guarantee renewable energy producers a fixed, long-term payment for the electricity they generate and feed into the grid. FiTs are typically set above the market price of electricity to incentivize renewable energy investment and provide revenue certainty for project developers.
- Key Features: FiTs are often differentiated by technology, scale, and project location to reflect the varying costs and benefits of renewable energy generation. They may include degression rates to gradually reduce tariff levels over time as technology costs decline and deployment targets are met.
- Benefits: Feed-in tariffs provide stable and predictable revenue streams for renewable energy projects, attract investment, drive technology deployment, and accelerate the transition to renewable energy sources. They promote market growth, innovation, and competition while reducing reliance on fossil fuels and carbon-intensive energy sources.
- Renewable Portfolio Standards (RPS):
- Overview: Renewable portfolio standards, also known as renewable energy mandates or targets, require utilities, electricity suppliers, or energy consumers to procure a specified percentage or amount of electricity from renewable sources by a certain deadline. RPS policies vary by jurisdiction and may include carve-outs for specific technologies or sectors.
- Key Features: RPS policies set renewable energy targets and compliance obligations, establish eligibility criteria for qualifying renewable energy sources, and may include penalties for non-compliance. They provide a market-driven approach to renewable energy deployment and create demand for renewable energy certificates (RECs) to demonstrate compliance.
- Benefits: Renewable portfolio standards create market demand for renewable energy, drive investment in renewable energy projects, stimulate job creation, and reduce greenhouse gas emissions. They diversify the energy mix, enhance energy security, and promote renewable energy integration into the electricity grid.
- Net Metering:
- Overview: Net metering policies allow renewable energy system owners to offset their electricity consumption with on-site generation and receive credit for any excess electricity fed back into the grid. Net metering enables bi-directional energy flows between renewable energy producers and utilities, effectively reducing electricity bills and promoting self-consumption.
- Key Features: Net metering regulations specify eligibility criteria, metering arrangements, compensation rates, and billing mechanisms for renewable energy customers. Some net metering programs offer one-to-one credit for exported electricity, while others provide lower compensation rates or impose capacity limits.
- Benefits: Net metering encourages distributed renewable energy generation, empowers energy consumers to become prosumers, and fosters the adoption of rooftop solar, wind, and other distributed generation technologies. It promotes grid stability, resilience, and decentralization while reducing transmission and distribution losses.
- Tax Incentives and Grants:
- Overview: Tax incentives, grants, rebates, and subsidies are financial mechanisms used to incentivize renewable energy investment, development, and deployment. These incentives may include investment tax credits (ITCs), production tax credits (PTCs), accelerated depreciation, cash grants, and direct subsidies for renewable energy projects.
- Key Features: Tax incentives and grants vary by jurisdiction, technology, project size, and eligibility criteria. They provide financial support to renewable energy developers, investors, and consumers, offsetting upfront costs, reducing payback periods, and improving project economics.
- Benefits: Tax incentives and grants stimulate renewable energy investment, leverage private capital, and accelerate project development while reducing the cost of renewable energy technologies for end-users. They promote job creation, economic growth, and technology innovation while advancing national energy and climate goals.
- Green Procurement Policies:
- Overview: Green procurement policies require government agencies, public institutions, and corporations to purchase renewable energy, energy-efficient products, and environmentally sustainable services. These policies support market demand for renewable energy, drive market transformation, and demonstrate leadership in sustainability.
- Key Features: Green procurement policies may include renewable energy procurement targets, green building standards, energy efficiency requirements, and sustainability criteria for procurement contracts. They encourage energy savings, emission reductions, and resource conservation across public and private sectors.
- Benefits: Green procurement policies create demand-pull effects for renewable energy technologies, stimulate market growth, and expand renewable energy deployment beyond regulatory mandates. They demonstrate corporate social responsibility, reduce environmental footprints, and foster sustainable consumption and production patterns.
- Research and Development Funding:
- Overview: Research and development (R&D) funding support innovation, technology development, and demonstration projects in renewable energy and clean technology sectors. Government agencies, research institutions, and private entities invest in R&D to advance renewable energy technologies, improve performance, and reduce costs.
- Key Features: R&D funding programs provide grants, loans, contracts, and cooperative agreements to support renewable energy research, pilot projects,
Renewable Energy Integration:
Renewable energy integration refers to the process of incorporating variable renewable energy sources such as solar, wind, and hydroelectric power into the electricity grid while maintaining system reliability, stability, and efficiency. As the share of renewable energy in the energy mix continues to grow, effective integration strategies become increasingly important to ensure a smooth transition to a sustainable and low-carbon energy future. Here’s a detailed exploration of renewable energy integration:
- Grid Flexibility and Resilience:
- Demand Response: Demand response programs incentivize consumers to adjust their electricity consumption in response to supply fluctuations, grid conditions, or price signals. Demand-side management strategies such as time-of-use pricing, smart meters, and energy efficiency measures help balance supply and demand in real-time, reducing peak load and enhancing grid flexibility.
- Energy Storage: Energy storage technologies such as batteries, pumped hydro storage, and thermal storage systems play a crucial role in buffering variable renewable energy output, smoothing fluctuations, and providing grid ancillary services. Energy storage facilities store excess renewable energy during periods of high generation and discharge it when demand exceeds supply, optimizing grid operation and stability.
- Grid Interconnection: Grid interconnection enables the exchange of electricity between different regions, balancing renewable energy variability and load diversity. Interconnected grids, transmission corridors, and high-voltage power lines facilitate renewable energy integration by enabling surplus generation to be transmitted to areas with high demand or stored for future use.
- Forecasting and Predictive Analytics:
- Weather Forecasting: Accurate weather forecasting tools and predictive analytics help anticipate renewable energy generation patterns, solar irradiance, wind speeds, and hydrological conditions. Weather forecast models, satellite imagery, and meteorological data enable grid operators, energy traders, and renewable energy developers to optimize generation schedules, anticipate grid imbalances, and manage energy resources effectively.
- Load Forecasting: Load forecasting models predict electricity demand patterns, consumption trends, and peak load periods based on historical data, socio-economic factors, and market dynamics. Load forecasting tools assist grid operators in matching renewable energy supply with demand, optimizing dispatch schedules, and minimizing curtailment or spillage of renewable energy resources.
- Market Integration: Wholesale electricity markets, power exchanges, and energy trading platforms facilitate the integration of renewable energy into the grid by providing transparent pricing signals, market mechanisms, and financial instruments for buying, selling, and hedging renewable energy contracts. Market-based approaches promote competition, efficiency, and investment in renewable energy infrastructure.
- Grid Modernization and Digitalization:
- Smart Grid Technologies: Smart grid technologies such as advanced metering infrastructure (AMI), grid automation, and distributed energy management systems enable real-time monitoring, control, and optimization of grid operations. Smart grids improve grid reliability, resilience, and efficiency by facilitating two-way communication between grid assets, renewable energy generators, and energy consumers.
- Decentralized Energy Systems: Decentralized energy systems, microgrids, and community-based renewable energy projects enhance energy autonomy, resilience, and self-sufficiency at the local level. Distributed generation, energy storage, and demand-side resources empower communities to manage their energy supply, reduce dependence on centralized infrastructure, and respond to grid disruptions or emergencies.
- Data Analytics and AI: Data analytics, machine learning, and artificial intelligence (AI) technologies analyze vast amounts of data from sensors, meters, and grid devices to optimize renewable energy integration, grid stability, and asset performance. AI-driven algorithms forecast renewable energy output, automate grid operations, and identify anomalies or inefficiencies in real-time, improving system reliability and response.
- Regulatory and Policy Frameworks:
- Grid Codes and Standards: Grid codes, technical standards, and interconnection requirements specify the technical requirements, performance criteria, and operating protocols for integrating renewable energy into the grid. Grid codes ensure the safe, reliable, and interoperable operation of renewable energy systems, grid-connected inverters, and protection devices.
- Market Design and Incentives: Market design reforms, capacity markets, and renewable energy incentives encourage investment in renewable energy infrastructure, grid upgrades, and energy storage projects. Policy instruments such as feed-in tariffs, renewable energy certificates (RECs), and carbon pricing mechanisms create market signals and financial incentives for renewable energy deployment.
- Energy Transition Roadmaps: Energy transition roadmaps, national energy strategies, and long-term decarbonization plans provide policy guidance, regulatory certainty, and investment signals for renewable energy integration. Governments, utilities, and stakeholders collaborate to set renewable energy targets, phase out fossil fuel subsidies, and implement supportive policies for renewable energy transition.
- International Cooperation and Knowledge Sharing:
- Global Collaboration: International organizations, multilateral initiatives, and bilateral partnerships facilitate knowledge sharing, capacity building, and technology transfer for renewable energy integration. Platforms such as the International Renewable Energy Agency (IRENA), Clean Energy Ministerial (CEM), and International Energy Agency (IEA) promote best practices, policy harmonization, and collaboration
Renewable Energy Technologies:
Renewable energy technologies encompass a diverse array of technologies that harness natural resources such as sunlight, wind, water, biomass, and geothermal heat to produce clean and sustainable energy. These technologies play a critical role in mitigating climate change, enhancing energy security, and promoting sustainable development. Here’s an in-depth exploration of renewable energy technologies:
- Solar Photovoltaic (PV) Systems:
- Overview: Solar photovoltaic systems convert sunlight directly into electricity using semiconductor materials (e.g., silicon) that generate an electric current when exposed to light. Solar PV systems range from small-scale rooftop installations to utility-scale solar farms and can be deployed in various configurations, including grid-connected, off-grid, and hybrid systems.
- Key Features: Solar PV technologies include monocrystalline, polycrystalline, and thin-film solar cells, each with different efficiencies, costs, and performance characteristics. Solar PV systems require solar panels, inverters, mounting structures, and balance-of-system components to convert and deliver electricity to end-users or the grid.
- Benefits: Solar PV is abundant, scalable, and modular, making it suitable for diverse applications and geographic regions. Solar energy is renewable, emissions-free, and inexhaustible, reducing greenhouse gas emissions, air pollution, and dependency on fossil fuels. Solar PV also offers energy independence, grid resilience, and decentralized power generation.
- Wind Turbines:
- Overview: Wind turbines harness kinetic energy from wind to drive rotating blades connected to a generator, converting mechanical energy into electricity. Wind turbines come in various designs, including horizontal-axis and vertical-axis configurations, and can be deployed onshore, offshore, or in distributed wind systems.
- Key Features: Modern wind turbines feature tall towers, aerodynamic rotor blades, and pitch or yaw control systems to maximize energy capture and performance. Wind farms consist of multiple turbines interconnected through electrical cables to form wind power plants that feed electricity into the grid or serve local communities.
- Benefits: Wind energy is abundant, renewable, and cost-competitive, offering predictable electricity generation and long-term price stability. Wind power reduces carbon emissions, water consumption, and environmental impacts compared to fossil fuel-based electricity generation. Offshore wind farms utilize expansive ocean resources and offer higher wind speeds and capacity factors than onshore installations.
- Hydropower Systems:
- Overview: Hydropower systems harness the potential energy of flowing water to drive turbines connected to generators, converting gravitational energy into electricity. Hydropower technologies include conventional dams, run-of-river schemes, pumped storage facilities, and small-scale hydro installations.
- Key Features: Hydropower plants vary in size, capacity, and operation mode, with storage reservoirs, intake structures, penstocks, turbines, and spillways comprising their main components. Pumped storage hydropower systems store and release water between upper and lower reservoirs to balance grid demand and supply fluctuations.
- Benefits: Hydropower is a mature, reliable, and dispatchable renewable energy source that provides baseload, peaking, and ancillary services to the grid. Hydropower plants offer long lifespans, low operating costs, and flexibility in responding to changing electricity demand and market conditions. Small-scale hydro projects empower rural communities and promote decentralized energy access.
- Bioenergy Technologies:
- Overview: Bioenergy technologies utilize organic biomass feedstocks such as agricultural residues, forestry waste, energy crops, and organic waste to produce heat, electricity, biofuels, and bioproducts. Bioenergy pathways include combustion, gasification, fermentation, and biochemical conversion processes.
- Key Features: Bioenergy systems encompass biomass boilers, cogeneration plants, biogas digesters, biofuel refineries, and bio-based chemical production facilities. Biomass feedstocks undergo thermochemical or biochemical conversion to release energy-rich components such as sugars, lignin, cellulose, and hemicellulose.
- Benefits: Bioenergy provides dispatchable, storable, and versatile renewable energy solutions for heating, power generation, transportation, and industrial applications. Biofuels such as ethanol, biodiesel, and biogas offer carbon-neutral alternatives to fossil fuels, reducing greenhouse gas emissions and promoting sustainable resource management practices.
- Geothermal Power Plants:
- Overview: Geothermal power plants utilize heat from the Earth’s crust to generate electricity through steam or hot water extraction from geothermal reservoirs. Geothermal resources are categorized as hydrothermal (conventional) or enhanced (engineered) geothermal systems (EGS) based on geological conditions and resource characteristics.
- Key Features: Geothermal power plants include dry steam, flash steam, binary cycle, and enhanced geothermal systems (EGS) technologies, each with specific drilling, extraction, and power generation methods. Geothermal wells tap into high-temperature reservoirs to produce steam or hot water that drives turbines connected to generators.
- Benefits: Geothermal energy is reliable, baseload, and emissions-free, providing continuous and predictable electricity generation with minimal environmental impact. Geothermal power plants have small land footprints and can be located near demand centers, reducing transmission losses and grid congestion. EGS technologies unlock new geothermal resources and expand geothermal potential worldwide.
- Ocean Energy Technologies:
- Overview: Ocean energy technologies harness
Ocean Energy Technologies:
Ocean energy technologies harness the vast energy potential of the world’s oceans to generate electricity from various renewable sources, including waves, tides, currents, and thermal gradients. These technologies offer a promising avenue for sustainable energy production, with the potential to provide clean and reliable power to coastal regions and island nations. Here’s an in-depth exploration of ocean energy technologies:
- Wave Energy Converters (WECs):
- Overview: Wave energy converters capture the kinetic energy of ocean waves and convert it into electricity through various mechanical, hydraulic, or pneumatic systems. WECs come in different designs, including point absorbers, oscillating water columns, attenuators, and overtopping devices, each optimized for specific wave conditions and deployment scenarios.
- Key Features: Wave energy converters typically consist of floating or fixed structures equipped with wave-activated mechanisms, power take-off (PTO) systems, and electrical generators. WECs harness wave motion, pressure differentials, or wave-induced oscillations to drive turbines, pistons, or oscillating bodies, producing electricity that is transmitted to shore via subsea cables.
- Benefits: Wave energy is abundant, predictable, and highly concentrated along coastlines, offering a continuous and renewable energy resource with minimal environmental impact. Wave energy converters complement other renewable energy sources, such as wind and solar, providing reliable power generation and grid stability.
- Tidal Energy Systems:
- Overview: Tidal energy systems capture the kinetic energy of ocean tides and currents to generate electricity using tidal turbines, underwater turbines, or tidal barrages. Tidal energy technologies exploit the gravitational forces of the moon and sun, causing periodic changes in sea levels and water velocities in coastal areas.
- Key Features: Tidal turbines are installed on the seabed or mounted on floating platforms to extract energy from tidal flows, while tidal barrages consist of dams or sluice gates that impound water during high tide and release it through turbines during ebb tide. Tidal range and velocity dictate the design, location, and efficiency of tidal energy systems.
- Benefits: Tidal energy is predictable, reliable, and highly concentrated near coastlines, offering a stable and dispatchable renewable energy resource with low variability. Tidal power plants provide baseload electricity generation, grid stability, and flood control benefits, enhancing coastal resilience and energy security.
- Ocean Current Turbines:
- Overview: Ocean current turbines or marine hydrokinetic (MHK) devices capture energy from ocean currents and underwater streams to generate electricity using underwater turbines or underwater kites. Ocean currents result from temperature gradients, wind patterns, and planetary forces, creating continuous flows of kinetic energy in ocean basins.
- Key Features: Ocean current turbines are anchored to the seabed or tethered to floating platforms to exploit horizontal or vertical currents at various depths. Turbines are equipped with rotors, blades, or hydrofoils that rotate or oscillate in response to water flow, driving electrical generators and producing renewable electricity.
- Benefits: Ocean current energy is abundant, persistent, and uniform, offering a reliable and predictable energy source with minimal visual and environmental impacts. Ocean current turbines operate continuously, providing steady electricity generation and grid stability without intermittency or seasonality.
- Ocean Thermal Energy Conversion (OTEC):
- Overview: Ocean thermal energy conversion utilizes temperature differences between warm surface waters and cold deep waters to drive a heat engine and generate electricity. OTEC systems rely on the thermodynamic properties of seawater, employing closed-cycle, open-cycle, or hybrid configurations to produce power.
- Key Features: OTEC plants consist of surface heat exchangers, evaporators, turbines, condensers, and cold water pipes that circulate seawater and working fluids such as ammonia or hydrocarbons. Warm surface seawater vaporizes a working fluid with a low boiling point, expanding and driving a turbine to generate electricity, while cold deep seawater condenses the vapor back into liquid.
- Benefits: OTEC offers a continuous, baseload renewable energy source with high capacity factors and minimal environmental impact. OTEC systems can provide desalinated freshwater, air conditioning, and aquaculture benefits alongside electricity generation, promoting integrated ocean resource utilization and sustainable development.
- Salinity Gradient Power (SGP):
- Overview: Salinity gradient power, also known as blue energy or osmotic power, exploits the energy potential of salinity gradients between freshwater and seawater to generate electricity through reverse electrodialysis (RED) or pressure retarded osmosis (PRO) processes. SGP utilizes membrane technologies and ion exchange to extract energy from the mixing of saline and fresh waters.
- Key Features: SGP systems consist of semipermeable membranes, ion-selective electrodes, and pressure differentials that facilitate ion transport and electricity generation. RED systems utilize alternating ion-exchange membranes to separate ions and generate electrical currents, while PRO systems utilize osmotic pressure differentials to drive turbines and produce power.
- Benefits: SGP offers a renewable energy source that exploits naturally occurring salinity gradients in
Renewable Energy Storage:
Renewable energy storage technologies play a crucial role in facilitating the integration of intermittent renewable energy sources such as solar and wind power into the electricity grid, ensuring grid stability, reliability, and resilience. These technologies enable the efficient capture, conversion, and storage of surplus renewable energy during periods of high generation for later use during periods of high demand or low generation. Here’s a comprehensive exploration of renewable energy storage technologies:
- Battery Energy Storage Systems (BESS):
- Overview: Battery energy storage systems store electricity in chemical form using rechargeable batteries, which can be charged or discharged based on grid conditions and energy demand. BESSs are modular, scalable, and versatile, making them suitable for various applications ranging from grid stabilization to residential energy management.
- Key Features: BESSs utilize lithium-ion, lead-acid, flow, or sodium-ion batteries with different energy densities, cycling capabilities, and lifespans. Battery management systems (BMS) monitor and control battery performance, charging rates, and state-of-charge (SOC) levels to optimize energy storage and grid services.
- Benefits: BESSs provide fast response times, grid frequency regulation, and peak shaving capabilities, enhancing grid stability and reliability. Battery storage systems mitigate renewable energy intermittency, enable renewable energy firming, and facilitate renewable energy self-consumption for residential, commercial, and industrial users.
- Pumped Hydroelectric Storage (PHS):
- Overview: Pumped hydroelectric storage facilities store energy by pumping water from lower to upper reservoirs during periods of low demand or surplus generation and release it through turbines to generate electricity during peak demand or low renewable energy output. PHS is the most mature and widely deployed grid-scale energy storage technology globally.
- Key Features: PHS systems consist of upper and lower reservoirs, reversible turbines, pumps, and penstocks that facilitate water transfer and energy conversion. PHS plants have high energy efficiency, rapid response times, and large storage capacities, making them ideal for bulk energy storage and grid balancing.
- Benefits: PHS provides grid stability, load balancing, and ancillary services such as frequency regulation and reserve capacity, supporting renewable energy integration and system reliability. PHS facilities have long lifespans, low operational costs, and high round-trip efficiency, making them cost-effective and environmentally sustainable energy storage solutions.
- Compressed Air Energy Storage (CAES):
- Overview: Compressed air energy storage systems store energy by compressing ambient air into underground caverns or aboveground tanks during off-peak periods and expanding it through turbines to generate electricity during peak demand or low renewable energy output. CAES is a flexible and scalable grid-scale energy storage technology.
- Key Features: CAES facilities include compressors, storage vessels, heat exchangers, and turbines that compress, store, and expand air to drive generators and produce electricity. CAES plants can be adiabatic, diabatic, or isothermal depending on the method used to manage heat during compression and expansion processes.
- Benefits: CAES offers large-scale energy storage, grid stabilization, and dispatchable power generation capabilities, enhancing renewable energy integration and grid reliability. CAES systems have long lifespans, low environmental impact, and high energy efficiency, making them suitable for base-load and peaking power applications.
- Flywheel Energy Storage Systems (FESS):
- Overview: Flywheel energy storage systems store kinetic energy in a rotating mass (flywheel) and convert it back into electricity using a motor-generator assembly. FESSs provide short-duration, high-power energy storage solutions for grid stabilization, frequency regulation, and backup power applications.
- Key Features: FESSs consist of a flywheel rotor, magnetic bearings, vacuum chamber, and motor-generator unit that spin at high speeds to store and release energy. Advanced materials, such as carbon fiber or steel alloys, are used to manufacture lightweight and durable flywheels with minimal friction and energy loss.
- Benefits: FESSs offer rapid response times, high cycling capabilities, and maintenance-free operation, providing grid stability and reliability services for renewable energy integration. FESSs are scalable, modular, and environmentally friendly, with no hazardous chemicals or emissions, making them suitable for urban and remote deployments.
- Thermal Energy Storage (TES):
- Overview: Thermal energy storage systems store energy in the form of heat or cold using phase change materials (PCMs), molten salts, or chilled water during periods of excess renewable energy generation and release it for heating, cooling, or power generation when needed. TES technologies are versatile and applicable to various sectors, including buildings, industry, and power plants.
- Key Features: TES systems include sensible, latent, and thermochemical storage technologies that capture and release thermal energy through heat exchange processes. TES solutions can be integrated with solar thermal power plants, district heating networks, or HVAC systems to improve energy efficiency and demand flexibility.
- Benefits: TES provides grid flexibility, demand-side management, and energy cost savings by shifting electricity consumption from peak to off-peak periods. TES enhances renewable energy utilization, reduces curtailment, and optimizes renewable energy dispatch, improving system economics and environmental performance.
- Hydrogen Energy Storage:
- Overview: Hydrogen energy storage systems produce, store, and utilize hydrogen gas as an energy carrier for long-term energy storage and transportation applications. Hydrogen can be generated through electrolysis using surplus renewable electricity, stored in tanks or underground caverns, and converted back into electricity through fuel cells or combustion engines.
- Key Features: Hydrogen storage technologies include compressed hydrogen tanks, liquid hydrogen tanks, and underground hydrogen caverns that store hydrogen gas at high pressure or cryogenic temperatures. Hydrogen production methods, such as water electrolysis or steam methane reforming, determine the overall efficiency and environmental footprint of hydrogen energy storage.
- Benefits: Hydrogen energy storage offers high energy density, long-duration storage, and fuel versatility for various energy applications, including transportation, industry, and power generation. Hydrogen can be stored indefinitely and transported over long distances, making it a flexible and scalable energy carrier for renewable
Renewable Energy Grid Integration:
Renewable energy grid integration refers to the process of incorporating variable renewable energy sources such as solar, wind, and hydroelectric power into existing electricity grids while maintaining system stability, reliability, and efficiency. As the share of renewable energy in the global energy mix continues to increase, effective grid integration strategies become essential to maximize the benefits of clean energy generation and mitigate challenges associated with intermittency and variability. Here’s a comprehensive exploration of renewable energy grid integration:
- Grid Capacity and Reinforcement:
- Overview: Grid capacity and reinforcement involve upgrading and expanding electricity transmission and distribution infrastructure to accommodate the integration of large-scale renewable energy projects. This includes building new transmission lines, substations, and interconnections to connect renewable energy sites to the grid and enable the seamless flow of electricity.
- Key Features: Grid capacity upgrades may involve increasing line capacity, voltage levels, and system redundancy to handle the additional load from renewable energy sources. Reinforcement measures address grid congestion, voltage stability, and power quality issues to ensure reliable and efficient electricity transmission and distribution.
- Benefits: Grid capacity and reinforcement enhance renewable energy deployment by reducing transmission bottlenecks, minimizing curtailment, and improving grid reliability. Upgraded transmission infrastructure enables renewable energy resources to reach distant load centers, balancing supply and demand across regions and supporting energy market integration.
- Demand-Side Management (DSM):
- Overview: Demand-side management (DSM) involves modifying electricity consumption patterns and behaviors to align with renewable energy generation profiles and grid conditions. DSM strategies aim to shift electricity demand from peak to off-peak periods, optimize energy use, and reduce overall electricity consumption through efficiency measures and demand response programs.
- Key Features: DSM initiatives include time-of-use pricing, smart metering, energy efficiency programs, and load control measures that incentivize consumers to adjust their electricity usage based on price signals, supply availability, and environmental considerations. Demand response programs enable industrial, commercial, and residential customers to curtail or shift electricity demand during periods of high renewable energy output or grid constraints.
- Benefits: DSM reduces peak demand, grid stress, and energy costs while enhancing the flexibility and resilience of the electricity system. Demand-side management optimizes renewable energy utilization, minimizes the need for fossil fuel backup generation, and supports the integration of variable renewable energy sources into the grid.
- Energy Storage Systems:
- Overview: Energy storage systems (ESS) play a crucial role in balancing supply and demand, smoothing renewable energy fluctuations, and providing grid stability services. ESS store surplus renewable energy during periods of high generation and discharge it during periods of low generation or high demand, optimizing grid operation and maximizing renewable energy utilization.
- Key Features: Energy storage technologies include battery storage, pumped hydro storage, compressed air storage, and thermal storage systems that store energy in chemical, mechanical, or thermal forms. Energy storage systems provide grid ancillary services such as frequency regulation, voltage control, and black start capabilities to support grid integration and reliability.
- Benefits: Energy storage enhances renewable energy integration by mitigating intermittency, variability, and imbalances in supply and demand. Storage systems improve grid flexibility, resilience, and response times, enabling renewable energy to compete with conventional fossil fuel-based generation on reliability and dispatchability.
- Grid-Interactive Renewable Energy Systems (RES):
- Overview: Grid-interactive renewable energy systems enable renewable energy generators to interact dynamically with the grid, adjusting output levels and operating characteristics in response to grid signals and market conditions. Grid-interactive RES technologies include grid-connected solar PV, wind turbines, and hydroelectric generators equipped with power electronics and control systems.
- Key Features: Grid-interactive RES technologies incorporate inverters, converters, and grid-support functions that enable bidirectional power flow, voltage regulation, and frequency control. These systems comply with grid codes, standards, and interconnection requirements to ensure safe and stable integration with the electricity grid.
- Benefits: Grid-interactive RES technologies enhance grid stability, reliability, and efficiency by providing active power control, reactive power support, and grid support functions. These systems enable renewable energy generators to participate in energy markets, provide grid services, and contribute to system balancing and optimization efforts.
- Advanced Grid Modeling and Forecasting:
- Overview: Advanced grid modeling and forecasting techniques leverage data analytics, machine learning, and artificial intelligence to simulate, predict, and optimize renewable energy integration and grid operation. These modeling tools enable grid operators, planners, and stakeholders to assess the impact of renewable energy variability, forecast renewable energy output, and optimize grid dispatch and scheduling.
- Key Features: Grid modeling platforms integrate meteorological data, renewable energy forecasts, grid topology, and demand projections to simulate grid behavior under different scenarios. Advanced forecasting algorithms predict renewable energy generation, grid congestion, and market prices with high accuracy, enabling proactive grid management and optimization.
- Benefits: Advanced grid modeling and forecasting improve grid planning, operation, and reliability by providing actionable insights
Interconnection Standards:
Interconnection standards refer to the technical specifications, regulations, and protocols governing the integration of distributed energy resources (DERs) such as rooftop solar panels, wind turbines, and energy storage systems into the electricity grid. These standards ensure safe, reliable, and efficient grid operation while facilitating the seamless connection and interoperability of DERs with the existing grid infrastructure. Here’s an in-depth exploration of interconnection standards:
- Voltage and Frequency Compatibility:
- Interconnection standards define the voltage and frequency requirements for DERs to ensure compatibility with the grid. DERs must operate within specified voltage limits and frequency tolerances to maintain grid stability and prevent disruptions. Voltage regulation and frequency control mechanisms may be mandated to synchronize DER output with grid conditions.
- Grid Protection and Safety Requirements:
- Interconnection standards establish grid protection and safety requirements to prevent islanding, voltage surges, and other grid disturbances caused by DER operation. Anti-islanding protection mechanisms detect grid outages and disconnect DERs from the grid to prevent backfeeding and protect utility personnel and equipment during maintenance or emergencies.
- Technical Certification and Testing Procedures:
- Interconnection standards prescribe technical certification and testing procedures for DER equipment and components to ensure compliance with performance, safety, and reliability requirements. DER manufacturers and installers must adhere to standardized testing protocols, verification processes, and quality assurance measures to demonstrate equipment reliability and grid compatibility.
- Communication and Control Protocols:
- Interconnection standards specify communication and control protocols for DERs to enable grid monitoring, remote operation, and data exchange. Communication interfaces, data formats, and messaging protocols facilitate real-time monitoring, control, and coordination of DERs by grid operators, enabling grid integration and optimization.
- Grid Code Compliance:
- Interconnection standards mandate compliance with grid codes, technical requirements, and interconnection agreements established by regulatory authorities or grid operators. DER owners and developers must adhere to grid code provisions related to voltage regulation, reactive power control, fault ride-through capability, and grid support functions to ensure seamless integration and grid stability.
- Fault Ride-Through and Grid Support Functions:
- Interconnection standards require DERs to demonstrate fault ride-through capability and provide grid support functions such as voltage regulation, reactive power control, and frequency response during grid disturbances or abnormal operating conditions. DERs contribute to grid stability, resilience, and reliability by responding dynamically to grid signals and operating requirements.
- Interconnection Application and Approval Process:
- Interconnection standards establish the application, review, and approval process for connecting DERs to the grid. DER owners or developers must submit interconnection applications detailing system specifications, site conditions, and compliance with interconnection requirements. Grid operators evaluate interconnection requests, perform impact studies, and issue interconnection agreements outlining technical specifications, connection fees, and operational conditions.
- Grid Interconnection Capacity and Queue Management:
- Interconnection standards define grid interconnection capacity limits and queue management procedures to prioritize and allocate interconnection requests based on grid capacity constraints, system reliability, and operational priorities. Grid operators manage interconnection queues, coordinate system upgrades, and allocate transmission capacity to accommodate DER integration while maintaining grid stability and reliability.
- DER Performance Monitoring and Reporting:
- Interconnection standards require DER owners to monitor, report, and maintain performance data for grid-connected DERs to ensure compliance with interconnection requirements and operational standards. Performance monitoring systems track DER output, operating parameters, and grid interactions, enabling real-time performance assessment, troubleshooting, and optimization.
- Grid Resilience and Cybersecurity:
- Interconnection standards address grid resilience and cybersecurity concerns by implementing measures to protect DERs from cyber threats, physical attacks, and natural disasters. Cybersecurity protocols, access controls, and encryption standards safeguard DER communication networks and data systems, ensuring the integrity, confidentiality, and availability of grid operations and information.
Renewable Energy Adoption:
Renewable energy adoption refers to the widespread utilization of renewable energy sources such as solar, wind, hydroelectric, geothermal, and biomass to meet energy needs while reducing reliance on fossil fuels. The transition to renewable energy is driven by various factors including environmental concerns, energy security, economic benefits, and technological advancements.
At the heart of renewable energy adoption is the recognition of the finite nature of fossil fuels and the need to mitigate climate change by reducing greenhouse gas emissions. Unlike fossil fuels, renewable energy sources are abundant, widely distributed, and have minimal environmental impacts, making them a sustainable alternative for power generation.
Solar energy, harvested through photovoltaic panels or concentrated solar power plants, harnesses the sun’s abundant and free energy to generate electricity. Wind energy, captured by turbines, utilizes the kinetic energy of moving air to produce electricity. Hydroelectric power relies on the gravitational force of flowing or falling water to spin turbines and generate electricity. Geothermal energy taps into heat stored beneath the Earth’s surface to produce steam and drive turbines for electricity generation. Biomass energy, derived from organic materials such as wood, agricultural residues, and municipal solid waste, can be burned directly for heat or electricity production, or converted into biofuels like ethanol and biodiesel.
The adoption of renewable energy technologies is facilitated by supportive policies, incentives, and investments at the national, regional, and local levels. Governments around the world are implementing renewable portfolio standards, feed-in tariffs, tax credits, and subsidies to promote the deployment of renewable energy projects and accelerate the transition to a low-carbon economy.
Moreover, advancements in renewable energy technology have led to significant cost reductions and improved efficiency, making renewables increasingly competitive with conventional energy sources. Innovations in energy storage, grid integration, and digitalization are further enhancing the reliability and scalability of renewable energy systems, enabling their seamless integration into existing energy infrastructure.
Beyond environmental benefits, renewable energy adoption also offers numerous economic and social advantages. It stimulates job creation, promotes local economic development, and reduces energy poverty by providing affordable and reliable electricity access to underserved communities. Additionally, by diversifying energy sources and reducing reliance on imported fuels, renewable energy adoption enhances energy security and resilience to supply disruptions.
In conclusion, renewable energy adoption represents a transformative shift towards a more sustainable, resilient, and equitable energy future. By harnessing the abundant and inexhaustible energy resources of nature, societies can achieve energy independence, mitigate climate change, and build a cleaner, greener world for future generations.
Domestic Energy Production:
Domestic energy production refers to the extraction, processing, and utilization of energy resources within a country’s borders to meet the nation’s energy needs. It encompasses various forms of energy, including fossil fuels such as oil, natural gas, and coal, as well as renewable energy sources like solar, wind, hydroelectric, geothermal, and biomass.
The pursuit of domestic energy production is driven by multiple factors, including energy security, economic development, job creation, and environmental considerations. By reducing reliance on imported energy sources, countries aim to enhance their energy independence, mitigate supply disruptions, and safeguard national interests.
Fossil fuel extraction, particularly oil and natural gas drilling, has historically played a significant role in domestic energy production, powering transportation, electricity generation, and industrial processes. Advances in drilling technologies, such as hydraulic fracturing (fracking) and horizontal drilling, have unlocked vast reserves of shale gas and tight oil, leading to a resurgence in domestic energy production in countries like the United States.
Coal mining, while declining in many regions due to environmental concerns and market forces, still contributes to domestic energy production in countries with significant coal reserves. However, efforts to reduce greenhouse gas emissions and transition to cleaner energy sources are driving a shift away from coal towards cleaner alternatives.
In contrast, renewable energy sources offer opportunities for sustainable and environmentally friendly domestic energy production. Solar photovoltaic (PV) panels, wind turbines, hydroelectric dams, geothermal power plants, and biomass facilities can generate electricity locally, reducing dependence on fossil fuels and mitigating carbon emissions.
The development of domestic renewable energy resources requires investments in infrastructure, technology, and policy support to overcome technical, economic, and regulatory barriers. Governments play a crucial role in promoting renewable energy deployment through incentives, subsidies, tax credits, and renewable energy targets.
The benefits of domestic energy production extend beyond energy security to include economic growth, job creation, and environmental stewardship. By investing in clean energy infrastructure and innovation, countries can stimulate economic activity, create green jobs, and reduce their carbon footprint, contributing to global efforts to combat climate change.
However, it’s essential to recognize the environmental and social impacts associated with energy production, whether from fossil fuels or renewables. Sustainable energy development requires careful consideration of land use, water resources, wildlife habitat, and community engagement to minimize negative consequences and maximize benefits for present and future generations.
In conclusion, domestic energy production is a critical component of a country’s energy strategy, influencing its economic competitiveness, environmental sustainability, and national security. By diversifying energy sources, embracing innovation, and prioritizing sustainability, nations can achieve energy independence while addressing the challenges of the 21st century energy landscape.
Energy Efficiency Measures:
Energy efficiency measures encompass a wide range of strategies, technologies, and practices aimed at reducing energy consumption while maintaining or improving the quality and productivity of energy services. These measures focus on optimizing energy use across various sectors, including buildings, transportation, industry, and agriculture, to minimize waste, lower energy costs, and mitigate environmental impacts.
- Building Energy Efficiency:
- Building energy efficiency initiatives include improving insulation, upgrading HVAC systems, installing energy-efficient lighting, and utilizing smart building technologies to reduce energy consumption in residential, commercial, and institutional buildings.
- Transportation Efficiency:
- Transportation efficiency measures promote the use of fuel-efficient vehicles, public transit, carpooling, cycling, and walking to reduce fuel consumption, greenhouse gas emissions, and traffic congestion. Encouraging telecommuting and adopting eco-driving practices further contribute to transportation energy efficiency.
- Industrial Process Optimization:
- Industrial energy efficiency initiatives focus on optimizing manufacturing processes, upgrading equipment, implementing energy management systems, and recovering waste heat to improve energy productivity, reduce operating costs, and enhance competitiveness.
- Agricultural Practices:
- Energy-efficient agricultural practices include optimizing irrigation systems, utilizing energy-efficient equipment and machinery, adopting precision farming techniques, and implementing renewable energy solutions such as solar-powered irrigation pumps to reduce energy inputs and improve agricultural productivity.
- Appliance and Equipment Standards:
- Setting minimum energy performance standards for appliances, electronics, and industrial equipment encourages the adoption of energy-efficient technologies and drives market transformation towards more sustainable products and practices.
- Energy Management Systems:
- Energy management systems (EMS) enable real-time monitoring, control, and optimization of energy consumption in buildings, factories, and facilities. EMS platforms use data analytics, sensors, and automation to identify energy-saving opportunities, optimize equipment operation, and reduce energy waste.
- Behavioral Changes and Education:
- Promoting energy awareness, education, and behavioral changes among individuals, households, businesses, and organizations encourages energy-saving habits, such as turning off lights, unplugging electronics, adjusting thermostats, and using energy-efficient appliances.
- Government Incentives and Programs:
- Government incentives, rebates, tax credits, and financial assistance programs incentivize energy efficiency investments and support the adoption of energy-saving technologies and practices across various sectors.
- Energy Audits and Retrofits:
- Conducting energy audits and implementing retrofits in buildings, industrial facilities, and infrastructure systems identify energy inefficiencies, prioritize energy-saving opportunities, and optimize energy performance through upgrades, renovations, and improvements.
- Integrated Energy Planning:
- Integrated energy planning considers energy efficiency as a cornerstone of energy policy and infrastructure development, prioritizing energy conservation, demand-side management, and efficiency measures alongside supply-side investments and renewable energy deployment.
Energy efficiency measures offer significant economic, environmental, and social benefits by reducing energy bills, lowering greenhouse gas emissions, improving air quality, enhancing energy security, creating jobs, and promoting sustainable development. By embracing energy efficiency as a cornerstone of energy policy and practice, societies can achieve greater energy resilience, affordability, and sustainability for current and future generations.
Decentralized Energy Systems:
Decentralized energy systems, also known as distributed energy systems or distributed generation, represent a departure from traditional centralized power generation models by dispersing energy production and consumption across multiple smaller-scale sources and locations. These systems empower local communities, businesses, and individuals to generate, store, and manage their own energy, thereby increasing resilience, reliability, and sustainability while reducing reliance on centralized utilities. Here’s a detailed exploration of decentralized energy systems:
- Local Energy Generation:
- Decentralized energy systems enable local energy generation using a variety of renewable and non-renewable sources, including solar photovoltaics (PV), wind turbines, biomass facilities, micro-hydroelectric generators, combined heat and power (CHP) systems, and fuel cells. These distributed energy resources (DERs) can be deployed on-site or within close proximity to end-users, reducing transmission losses and improving efficiency.
- Microgrids:
- Microgrids are self-contained energy systems that can operate independently or in conjunction with the main grid. They integrate local generation, energy storage, and demand-side management technologies to supply electricity to specific areas or facilities. Microgrids enhance energy resilience by providing backup power during grid outages and enabling islanding capabilities to isolate from the main grid during emergencies.
- Energy Storage Solutions:
- Decentralized energy systems leverage energy storage solutions such as batteries, pumped hydro storage, flywheels, and thermal storage to store excess energy generated during periods of low demand or high renewable generation. Energy storage technologies enable load shifting, peak shaving, and grid stabilization, enhancing the flexibility and reliability of decentralized energy systems.
- Peer-to-Peer Energy Trading:
- Decentralized energy systems facilitate peer-to-peer energy trading platforms that enable producers and consumers to buy, sell, or exchange surplus energy directly with each other, bypassing traditional utility intermediaries. Blockchain technology and smart contracts facilitate transparent, secure, and automated transactions, empowering individuals and communities to participate in the energy market.
- Demand Response Programs:
- Decentralized energy systems employ demand response programs to incentivize consumers to adjust their electricity usage in response to grid conditions or price signals. Smart meters, energy management systems, and dynamic pricing schemes enable consumers to reduce peak demand, optimize energy consumption, and lower electricity bills while supporting grid stability and reliability.
- Resilience and Reliability:
- Decentralized energy systems enhance resilience and reliability by diversifying energy sources, reducing dependence on centralized infrastructure, and mitigating the risk of single points of failure. Local generation and storage capabilities provide backup power during emergencies, natural disasters, or grid disruptions, ensuring continuous energy supply to critical facilities and essential services.
- Environmental Benefits:
- Decentralized energy systems promote environmental sustainability by prioritizing renewable energy sources, reducing greenhouse gas emissions, and minimizing environmental impacts associated with centralized power generation, transmission, and distribution. Localized energy production reduces transmission losses and minimizes the need for long-distance energy transportation.
- Community Empowerment:
- Decentralized energy systems empower communities to take control of their energy destiny, enabling local decision-making, investment, and ownership of energy infrastructure. Community-owned renewable energy projects, cooperatives, and participatory governance models foster collaboration, social cohesion, and economic development while promoting energy democracy and equity.
- Scalability and Flexibility:
- Decentralized energy systems are inherently scalable and flexible, allowing for incremental deployment, expansion, and adaptation to changing energy needs, technologies, and market dynamics. Modular and plug-and-play solutions enable rapid deployment and integration of new energy resources, supporting the transition to a more sustainable and resilient energy future.
- Policy and Regulatory Support:
- Decentralized energy systems require supportive policy and regulatory frameworks that incentivize investment, innovation, and participation in distributed energy markets. Policies such as net metering, feed-in tariffs, energy storage incentives, and regulatory reforms facilitate the integration of decentralized energy resources into the broader energy system while ensuring fair compensation and grid access.
In conclusion, decentralized energy systems represent a paradigm shift towards a more democratic, resilient, and sustainable energy future. By decentralizing energy production, distribution, and decision-making, communities can unlock new opportunities for local empowerment, economic development, and environmental stewardship while building a more resilient and equitable energy infrastructure for all.
Energy Storage Technologies:
Energy storage technologies play a crucial role in modern energy systems by enabling the capture, storage, and utilization of energy for later use. These technologies provide flexibility, reliability, and resilience to electricity grids, facilitate the integration of renewable energy sources, and support various applications across different sectors. Here’s an in-depth exploration of energy storage technologies:
- Battery Energy Storage Systems (BESS):
- Battery energy storage systems store electricity in chemical form and release it as needed. Lithium-ion batteries, lead-acid batteries, flow batteries, and other advanced battery technologies are commonly used for grid-scale, commercial, and residential energy storage applications. BESS can provide backup power, load shifting, frequency regulation, and grid stabilization services.
- Pumped Hydroelectric Storage:
- Pumped hydroelectric storage facilities store energy by pumping water from a lower reservoir to an upper reservoir during periods of low electricity demand and release it through turbines to generate electricity during peak demand. Pumped hydro storage is the largest and most established form of grid-scale energy storage, offering high efficiency and long-duration storage capabilities.
- Flywheel Energy Storage:
- Flywheel energy storage systems store kinetic energy in a rotating mass (flywheel) and release it as electricity when needed. Flywheels offer fast response times, high power density, and short-duration energy storage for applications requiring rapid grid stabilization, frequency regulation, and uninterrupted power supply.
- Compressed Air Energy Storage (CAES):
- Compressed air energy storage systems store energy by compressing air into underground caverns or above-ground tanks during off-peak hours and releasing it through turbines to generate electricity during peak demand periods. CAES provides large-scale, cost-effective energy storage with high efficiency and long-duration capabilities.
- Thermal Energy Storage (TES):
- Thermal energy storage systems store heat or cold in materials such as water, molten salt, or phase change materials for later use in heating, cooling, or electricity generation applications. TES technologies include sensible heat storage, latent heat storage, and thermochemical storage, offering flexibility and versatility for various thermal energy storage applications.
- Hydrogen Energy Storage:
- Hydrogen energy storage involves converting electricity into hydrogen through electrolysis and storing it for later use in fuel cells or combustion engines to generate electricity or provide heat. Hydrogen storage enables long-duration energy storage and can be used for grid balancing, transportation, industrial processes, and renewable energy integration.
- Supercapacitors and Ultracapacitors:
- Supercapacitors and ultracapacitors store electrical energy in an electric field and release it rapidly when needed. These energy storage devices offer high power density, fast charging/discharging rates, and long cycle life, making them suitable for applications requiring short-duration, high-power energy storage, such as regenerative braking in electric vehicles and grid stabilization.
- Redox Flow Batteries:
- Redox flow batteries store energy in electrolyte solutions contained in separate tanks and release it through electrochemical reactions. Flow batteries offer scalable, long-duration energy storage solutions with rapid response times and flexible discharge profiles, making them suitable for grid-scale energy storage and renewable energy integration.
- Hybrid Energy Storage Systems:
- Hybrid energy storage systems combine multiple energy storage technologies, such as batteries, capacitors, and supercapacitors, to leverage their complementary characteristics and optimize performance for specific applications. Hybrid systems offer enhanced efficiency, reliability, and flexibility compared to individual storage technologies alone.
- Emerging Energy Storage Technologies:
- Ongoing research and development efforts are focused on developing new and advanced energy storage technologies, including solid-state batteries, organic redox flow batteries, advanced hydrogen storage materials, and novel thermal energy storage concepts. These emerging technologies hold the potential to further improve energy storage performance, efficiency, and cost-effectiveness, driving innovation and market growth in the energy storage sector.
Energy storage technologies are essential enablers of the transition to a more sustainable, reliable, and resilient energy future. By providing grid stability, renewable energy integration, and backup power
during times of peak demand or grid outages, energy storage technologies contribute to a more efficient and flexible energy system. As renewable energy sources such as solar and wind become increasingly prevalent, energy storage solutions play a critical role in balancing supply and demand, smoothing out fluctuations in renewable energy generation, and ensuring a reliable electricity supply.
Moreover, energy storage technologies offer numerous benefits across various sectors:
- Grid Stability and Reliability: Energy storage systems support grid stability by providing frequency regulation, voltage control, and grid balancing services. They help to smooth out fluctuations in electricity demand and supply, reduce grid congestion, and enhance the overall reliability of the electric grid.
- Renewable Energy Integration: Energy storage enables the seamless integration of variable renewable energy sources such as solar and wind into the grid by storing excess energy when generation exceeds demand and releasing it when needed. This improves grid flexibility, reduces curtailment of renewable energy, and maximizes renewable energy utilization.
- Peak Shaving and Load Management: Energy storage systems can reduce peak electricity demand and associated costs by storing excess energy during off-peak hours and discharging it during peak demand periods. This peak shaving capability helps to alleviate stress on the grid, avoid the need for expensive peaking power plants, and lower electricity bills for consumers.
- Backup Power and Resilience: Energy storage provides backup power solutions for critical facilities, essential services, and remote communities during grid outages or emergencies. Standalone or hybrid energy storage systems with renewable energy sources can ensure uninterrupted power supply, improve energy resilience, and enhance disaster preparedness.
- Electrification of Transportation: Energy storage plays a crucial role in electrifying transportation through battery electric vehicles (BEVs) and hybrid electric vehicles (HEVs). Advanced battery technologies enable longer driving ranges, faster charging times, and improved vehicle performance, accelerating the transition away from fossil fuel-powered vehicles towards cleaner, more sustainable transportation options.
- Industrial and Commercial Applications: Energy storage solutions support a wide range of industrial and commercial applications, including peak demand management, time-of-use optimization, uninterruptible power supply (UPS) systems, and grid ancillary services. These applications improve energy efficiency, reduce operating costs, and enhance business continuity for industrial and commercial customers.
Overall, energy storage technologies are essential enablers of the transition to a more sustainable, reliable, and resilient energy system. Continued innovation, investment, and deployment of energy storage solutions will play a critical role in addressing the challenges of climate change, enhancing energy security, and advancing the transition towards a low-carbon future.
Renewable Energy Policies:
Renewable energy policies are government initiatives, regulations, incentives, and targets designed to promote the deployment, integration, and adoption of renewable energy sources for electricity generation, heating, cooling, transportation, and other energy applications. These policies aim to accelerate the transition from fossil fuels to clean, sustainable, and low-carbon energy sources, mitigate climate change, enhance energy security, and stimulate economic growth. Here’s an in-depth exploration of renewable energy policies:
- Renewable Portfolio Standards (RPS):
- Renewable portfolio standards mandate that a certain percentage of electricity generation come from renewable sources within a specified timeframe. RPS policies set targets for renewable energy deployment, incentivize investment in renewable energy projects, and drive market demand for clean energy technologies.
- Feed-in Tariffs (FITs):
- Feed-in tariffs guarantee long-term, fixed prices for electricity generated from renewable sources, providing renewable energy producers with a stable revenue stream and a guaranteed market for their power. FITs encourage renewable energy investment, reduce financing costs, and promote the rapid deployment of renewable energy projects.
- Net Metering and Feed-in Premiums:
- Net metering allows renewable energy system owners to offset their electricity consumption by exporting surplus electricity to the grid and receiving credits or payment for the energy they generate. Feed-in premiums offer additional compensation for renewable energy producers, incentivizing distributed generation and grid-connected renewable energy systems.
- Renewable Energy Certificates (RECs):
- Renewable energy certificates represent the environmental attributes of renewable electricity generation and can be bought, sold, or traded separately from the physical electricity. RECs provide a market-based mechanism for utilities, businesses, and individuals to support renewable energy development and claim ownership of renewable energy attributes.
- Tax Incentives and Rebates:
- Tax incentives, rebates, grants, and subsidies reduce the upfront costs of renewable energy investments and make clean energy technologies more affordable for consumers, businesses, and utilities. These financial incentives stimulate demand for renewable energy systems, drive innovation, and create jobs in the renewable energy sector.
- Green Procurement Policies:
- Green procurement policies require government agencies, public institutions, and corporations to prioritize the purchase of renewable energy, energy-efficient products, and services. Green procurement promotes market demand for clean energy technologies, drives economies of scale, and fosters innovation in the renewable energy industry.
- Renewable Energy Targets and Mandates:
- Renewable energy targets and mandates set specific goals for renewable energy deployment, such as a percentage of total energy consumption or electricity generation, by a certain date. These targets provide clear signals to investors, developers, and stakeholders, driving investment in renewable energy projects and facilitating the transition to a low-carbon energy system.
- Carbon Pricing and Emissions Trading:
- Carbon pricing mechanisms, such as carbon taxes and emissions trading systems (cap-and-trade), internalize the external costs of carbon emissions and create financial incentives for reducing greenhouse gas emissions. Carbon pricing encourages investment in renewable energy, energy efficiency, and low-carbon technologies, accelerating the transition to a low-carbon economy.
- Research and Development Funding:
- Government funding for research, development, and demonstration (RD&D) of renewable energy technologies supports innovation, technology advancement, and cost reduction in the renewable energy sector. RD&D funding accelerates the commercialization and deployment of emerging renewable energy technologies, driving down costs and improving performance over time.
- Regulatory Reforms and Market Design:
- Regulatory reforms and market design initiatives promote the integration of renewable energy into electricity markets, grid operations, and energy infrastructure planning. These reforms include streamlining permitting processes, updating grid interconnection standards, and implementing market mechanisms that value the flexibility and reliability of renewable energy resources.
Renewable energy policies vary by country, region, and jurisdiction, reflecting local energy resources, political priorities, economic conditions, and societal preferences. Effective renewable energy policies require stakeholder engagement, coordination across government agencies, industry collaboration, and long-term commitment to achieving renewable energy targets and objectives. By implementing a diverse and comprehensive suite of renewable energy policies, policymakers can create an enabling environment for renewable energy development, drive investment in clean energy technologies, and accelerate the transition to a sustainable and resilient energy future.
Renewable Energy Financing:
Renewable energy financing encompasses a variety of mechanisms, instruments, and approaches used to fund the development, construction, and operation of renewable energy projects. Access to financing is essential for overcoming the high upfront costs associated with renewable energy technologies and accelerating their deployment on a global scale. Here’s a detailed exploration of renewable energy financing:
- Project Finance:
- Project finance is a common financing mechanism for renewable energy projects, where lenders provide non-recourse loans based on the project’s cash flow and assets rather than the creditworthiness of the project sponsor. Project finance structures mitigate risks for investors and lenders, enabling the development of large-scale renewable energy projects with long-term revenue streams.
- Venture Capital and Private Equity:
- Venture capital (VC) and private equity (PE) firms invest in early-stage renewable energy companies and startups, providing capital for technology development, market entry, and business expansion. VC and PE investments support innovation, entrepreneurship, and the commercialization of new renewable energy technologies and business models.
- Public-Private Partnerships (PPPs):
- Public-private partnerships involve collaboration between government agencies, private companies, and financial institutions to finance, develop, and operate renewable energy projects. PPPs leverage public sector resources, private sector expertise, and project finance mechanisms to address market barriers, attract investment, and deliver sustainable infrastructure projects.
- Green Bonds and Sustainable Debt:
- Green bonds and sustainable debt instruments raise capital for renewable energy projects by issuing bonds or loans with proceeds earmarked for environmentally beneficial projects. Green bonds are certified as green by independent third parties, providing investors with transparency and assurance that their funds are financing renewable energy initiatives.
- Crowdfunding and Peer-to-Peer Lending:
- Crowdfunding platforms and peer-to-peer lending networks allow individuals, communities, and organizations to invest directly in renewable energy projects through small contributions or loans. Crowdfunding democratizes access to renewable energy investment opportunities, empowers local communities, and fosters grassroots support for clean energy initiatives.
- Development Finance Institutions (DFIs):
- Development finance institutions, such as the World Bank, regional development banks, and national development banks, provide concessional loans, grants, and technical assistance to support renewable energy projects in developing countries. DFIs mobilize capital, de-risk investments, and promote sustainable development through targeted financing and capacity-building initiatives.
- Green Investment Banks (GIBs):
- Green investment banks specialize in financing renewable energy and environmental projects, offering tailored financial products, credit enhancements, and risk mitigation tools to accelerate investment in clean energy infrastructure. GIBs bridge the gap between public and private finance, catalyzing investment in renewable energy markets.
- Tax Incentives and Subsidies:
- Governments provide tax incentives, subsidies, grants, and rebates to incentivize investment in renewable energy projects and support the growth of the renewable energy industry. Tax credits for renewable energy production, investment tax credits (ITCs), accelerated depreciation schedules, and cash grants reduce the cost of capital and improve project economics for developers and investors.
- Asset-Backed Securitization:
- Asset-backed securitization involves bundling revenue-generating assets, such as renewable energy projects, into securities that can be sold to investors in capital markets. Securitization unlocks liquidity, diversifies risk, and enables project developers to access additional sources of funding for renewable energy development.
- Carbon Markets and Offsets:
- Carbon markets and offset programs create financial incentives for reducing greenhouse gas emissions by allowing emitters to purchase carbon credits generated from renewable energy projects or emission reduction initiatives. Carbon pricing mechanisms, such as cap-and-trade systems and carbon taxes, provide additional revenue streams for renewable energy projects and incentivize emission reductions.
Renewable energy financing requires collaboration between project developers, investors, financial institutions, governments, and other stakeholders to mobilize capital, manage risks, and deliver sustainable energy solutions. By leveraging diverse financing mechanisms and innovative financial instruments, policymakers and industry stakeholders can accelerate the transition to a low-carbon, resilient, and inclusive energy system for the benefit of present and future generations.
Community Solar Projects:
Community solar projects, also known as solar gardens or shared solar programs, are initiatives that enable multiple individuals, businesses, or organizations to collectively invest in, own, or subscribe to a shared solar photovoltaic (PV) system installed at a centralized location. These projects expand access to solar energy by allowing participants who are unable to install solar panels on their own property to benefit from clean, renewable energy generation. Here’s a comprehensive overview of community solar projects:
- Shared Ownership and Subscription Models:
- Community solar projects offer shared ownership or subscription models, where participants purchase or lease a portion of the solar PV system’s capacity and receive credits or discounts on their electricity bills based on the energy generated by their share of the system. This allows renters, homeowners with shaded roofs, and those with insufficient space or financial resources to access solar energy.
- Centralized Solar Arrays:
- Community solar projects typically consist of centralized solar arrays installed on rooftops, vacant land, or other suitable locations within the community. These solar arrays are interconnected to the local electricity grid, and the electricity generated is fed into the grid for distribution to participating subscribers or off-takers.
- Virtual Net Metering:
- Virtual net metering allows community solar subscribers to receive credits on their electricity bills for their share of the solar energy produced, offsetting a portion of their electricity consumption. Subscribers benefit from lower electricity costs while supporting renewable energy generation and reducing their carbon footprint.
- Equitable Access and Inclusion:
- Community solar projects promote equitable access to solar energy by removing barriers to participation, such as upfront costs, property ownership requirements, and shading constraints. These projects empower low-income households, renters, multifamily housing residents, and underserved communities to access the benefits of solar energy and reduce energy costs.
- Local Economic Development:
- Community solar projects contribute to local economic development by creating jobs, generating tax revenue, and stimulating economic activity in the communities where they are located. Solar installation, maintenance, and related services provide employment opportunities, support local businesses, and strengthen the resilience of the local economy.
- Educational and Outreach Initiatives:
- Community solar projects often include educational and outreach initiatives to raise awareness about solar energy, energy efficiency, and sustainable practices. Workshops, informational sessions, and community events engage residents, businesses, schools, and community organizations in learning about renewable energy and environmental stewardship.
- Partnerships and Collaboration:
- Community solar projects involve partnerships and collaboration between project developers, utilities, local governments, community organizations, and stakeholders to plan, finance, and implement shared solar initiatives. Collaboration facilitates access to financing, grid interconnection, permitting, and regulatory approvals, ensuring the success and sustainability of community solar projects.
- Scalability and Replicability:
- Community solar projects are scalable and replicable, allowing communities to develop multiple projects of varying sizes and configurations to meet local energy needs and goals. Standardized project development processes, financing models, and regulatory frameworks facilitate the expansion of community solar across regions and jurisdictions.
- Environmental Benefits:
- Community solar projects reduce greenhouse gas emissions, air pollution, and reliance on fossil fuels by displacing conventional electricity generation with clean, renewable solar energy. By promoting distributed generation and local renewable energy production, community solar contributes to climate mitigation, environmental conservation, and public health improvement.
- Policy Support and Regulatory Frameworks:
- Policy support and regulatory frameworks play a crucial role in enabling the development and growth of community solar projects. Policies such as virtual net metering, community solar incentives, renewable energy mandates, and streamlined permitting processes create an enabling environment for community solar deployment and adoption.
In conclusion, community solar projects represent a scalable, inclusive, and community-driven approach to expanding access to solar energy, promoting energy equity, and advancing the transition to a clean and sustainable energy future. By leveraging shared ownership, collaboration, and local engagement, community solar initiatives empower communities to harness the sun’s abundant energy resources and build a more resilient, equitable, and environmentally sustainable energy system.
Microgrid Technology:
Microgrid technology is a decentralized energy system that consists of interconnected loads and distributed energy resources (DERs), such as renewable energy sources, energy storage systems, and controllable loads, operating as a single, autonomous entity or network. Microgrids can operate in both grid-connected and islanded modes, providing energy resilience, reliability, and flexibility to support critical infrastructure, remote communities, and commercial or industrial facilities. Here’s a detailed exploration of microgrid technology:
- Grid-Connected and Islanded Operation:
- Microgrids can operate in grid-connected mode, where they are synchronized with the main utility grid and can import or export electricity as needed. In islanded mode, microgrids can disconnect from the main grid and operate independently, relying on local energy resources to meet demand and maintain power supply during grid outages or emergencies.
- Distributed Energy Resources (DERs):
- Microgrids integrate a diverse mix of distributed energy resources, including solar photovoltaics (PV), wind turbines, biomass generators, fuel cells, combined heat and power (CHP) systems, and energy storage technologies such as batteries, flywheels, and pumped hydro storage. DERs provide onsite generation, storage, and demand management capabilities, enhancing energy resilience and reducing dependence on centralized power sources.
- Control and Automation Systems:
- Microgrids are equipped with advanced control and automation systems that monitor, optimize, and manage the operation of DERs in real-time. Smart grid technologies, predictive analytics, and energy management algorithms enable microgrid controllers to balance supply and demand, prioritize critical loads, and optimize energy use for maximum efficiency and cost savings.
- Energy Storage Integration:
- Energy storage systems, such as batteries, play a crucial role in microgrid operation by storing excess energy during periods of low demand and releasing it when needed to balance supply and demand, improve grid stability, and provide backup power during grid outages. Energy storage enhances the flexibility, reliability, and resilience of microgrid systems.
- Renewable Energy Integration:
- Microgrids often incorporate renewable energy sources, such as solar PV, wind, and biomass, to reduce carbon emissions, lower operating costs, and increase energy independence. Renewable energy integration diversifies the microgrid’s energy portfolio, reduces reliance on fossil fuels, and contributes to environmental sustainability.
- Demand Response and Load Management:
- Microgrids leverage demand response strategies and load management techniques to optimize energy use, reduce peak demand, and minimize electricity costs. Demand response programs incentivize consumers to adjust their energy consumption patterns in response to price signals or grid conditions, helping to balance supply and demand in real-time.
- Resilience and Reliability:
- Microgrids enhance energy resilience and reliability by reducing the impact of grid disturbances, blackouts, and natural disasters. By isolating critical loads and maintaining power supply during emergencies, microgrids support essential services, critical infrastructure, and community resilience in the face of unforeseen events.
- Scalability and Modularity:
- Microgrid systems are scalable and modular, allowing for flexible deployment and expansion to meet changing energy needs and accommodate future growth. Modular components, standardized interfaces, and plug-and-play integration enable rapid deployment and easy integration of new DERs into existing microgrid infrastructure.
- Remote and Off-Grid Applications:
- Microgrids are well-suited for remote and off-grid applications, such as rural electrification, remote communities, military bases, and industrial sites located in remote or isolated areas. Off-grid microgrids provide reliable power supply where grid connectivity is unavailable or unreliable, enabling economic development and improving quality of life for underserved populations.
- Policy and Regulatory Frameworks:
- Policy and regulatory frameworks play a critical role in enabling the deployment and operation of microgrid technology. Supportive policies, incentives, and regulatory reforms, such as microgrid tariffs, interconnection standards, and grid modernization initiatives, create an enabling environment for microgrid development, investment, and innovation.
Microgrid technology represents a transformative approach to energy management, offering resilience, reliability, and sustainability benefits for a wide range of applications. By integrating distributed energy resources, advanced control systems, and grid-edge technologies, microgrids empower communities, businesses, and institutions to take control of their energy future and build a more resilient, sustainable, and decentralized energy system.
Smart Grid Infrastructure:
Smart grid infrastructure refers to the modernization and enhancement of electricity grids through the integration of advanced technologies, digital communication systems, and intelligent devices to improve grid efficiency, reliability, flexibility, and sustainability. Smart grids enable two-way communication and real-time control of electricity flow, facilitating the integration of renewable energy sources, demand response programs, electric vehicles, and other distributed energy resources. Here’s an in-depth exploration of smart grid infrastructure:
- Advanced Metering Infrastructure (AMI):
- Advanced metering infrastructure, including smart meters, enables automated meter reading, remote monitoring, and real-time data collection of energy consumption patterns. Smart meters provide consumers with detailed information on their electricity usage, support time-of-use pricing, and enable utilities to implement demand response programs and dynamic pricing strategies.
- Distribution Automation Systems:
- Distribution automation systems improve the reliability and efficiency of electricity distribution networks by automating fault detection, isolation, and restoration processes. Smart sensors, switches, and control devices enable utilities to identify and respond to grid disturbances quickly, minimize outage duration, and enhance grid resilience.
- Grid Monitoring and Situational Awareness:
- Grid monitoring and situational awareness systems use sensors, communication networks, and data analytics to continuously monitor grid conditions, detect abnormalities, and predict potential failures. These systems provide operators with real-time insights into grid performance, enabling proactive maintenance, load balancing, and voltage regulation to optimize grid operations.
- Demand Response and Load Management:
- Smart grid infrastructure facilitates demand response programs and load management strategies to incentivize consumers to adjust their electricity consumption patterns in response to price signals or grid conditions. Automated demand response systems, smart appliances, and energy management platforms enable utilities to reduce peak demand, avoid grid congestion, and optimize energy use.
- Energy Storage Integration:
- Energy storage systems, such as batteries and flywheels, play a vital role in smart grid infrastructure by providing grid-scale storage capacity for storing excess energy during periods of low demand and discharging it when needed to balance supply and demand. Energy storage enhances grid flexibility, reliability, and resilience by smoothing out fluctuations in renewable energy generation and improving grid stability.
- Renewable Energy Integration:
- Smart grid technologies facilitate the integration of renewable energy sources, such as solar PV, wind, and biomass, into the electricity grid by enabling real-time monitoring, forecasting, and control of renewable energy generation. Grid-friendly renewable energy integration reduces curtailment, maximizes renewable energy utilization, and supports the transition to a low-carbon energy system.
- Electric Vehicle Charging Infrastructure:
- Smart grid infrastructure supports the deployment of electric vehicle (EV) charging infrastructure by enabling bi-directional communication between EV chargers, vehicles, and the grid. Smart charging solutions optimize EV charging schedules, manage grid impact, and support vehicle-to-grid (V2G) integration, where EV batteries can provide grid services and support grid stability.
- Cybersecurity and Resilience:
- Smart grid infrastructure incorporates robust cybersecurity measures and resilience strategies to protect against cyber threats, data breaches, and grid disruptions. Encryption, authentication, intrusion detection, and incident response protocols safeguard smart grid assets, communication networks, and data exchange, ensuring the integrity, confidentiality, and availability of grid operations.
- Grid Edge Technologies:
- Grid edge technologies, such as distributed energy resources (DERs), microgrids, and smart appliances, operate at the intersection of the electricity grid and end-user facilities, providing localized generation, storage, and demand-side management capabilities. Grid edge solutions enhance grid flexibility, support decentralized energy production, and empower consumers to actively participate in energy management.
- Interoperability and Standards:
- Interoperability standards and protocols ensure seamless integration and interoperability of smart grid devices, systems, and applications from different vendors and manufacturers. Common data formats, communication protocols, and interoperability standards facilitate the exchange of information, interoperability testing, and compatibility between smart grid components, enabling plug-and-play functionality and system scalability.
Smart grid infrastructure represents a transformative approach to modernizing the electricity grid, enhancing its capabilities, and unlocking new opportunities for energy efficiency, sustainability, and grid resilience. By leveraging advanced technologies, data analytics, and real-time communication, smart grids empower utilities, consumers, and stakeholders to optimize energy use, reduce emissions, and build a more adaptive, reliable, and sustainable energy system for the future.
Decentralized Energy Systems:
Decentralized energy systems represent a paradigm shift from traditional centralized energy production and distribution models towards a more distributed, localized approach to energy generation, storage, and consumption. These systems empower communities, businesses, and individuals to produce, manage, and control their own energy resources, reducing dependence on large-scale power plants and centralized grid infrastructure. Here’s a comprehensive exploration of decentralized energy systems:
- Distributed Energy Resources (DERs):
- Decentralized energy systems leverage a diverse mix of distributed energy resources, including solar photovoltaics (PV), wind turbines, biomass generators, small-scale hydroelectric systems, combined heat and power (CHP) units, and energy storage technologies. DERs provide localized generation, storage, and demand-side management capabilities, enabling communities to meet their energy needs independently.
- Microgrid Deployment:
- Microgrids are localized energy systems that operate autonomously or in conjunction with the main electricity grid, serving specific geographic areas, campuses, military bases, industrial facilities, or remote communities. Microgrids integrate DERs, energy storage, and advanced control systems to optimize energy use, enhance grid resilience, and provide reliable power supply during grid outages or emergencies.
- Peer-to-Peer Energy Trading:
- Decentralized energy systems facilitate peer-to-peer energy trading platforms, where individuals, businesses, and organizations can buy, sell, or exchange surplus energy directly with each other. Blockchain technology, smart contracts, and digital platforms enable secure, transparent, and automated transactions, fostering energy independence, community engagement, and economic empowerment.
- Community-Owned Energy Projects:
- Community-owned energy projects, such as cooperatives, community solar gardens, and local energy cooperatives, empower residents to collectively invest in, own, or operate renewable energy infrastructure. Community ownership promotes local decision-making, economic development, and social cohesion, while supporting the transition to a more sustainable and resilient energy system.
- Demand-Side Management (DSM):
- Decentralized energy systems employ demand-side management strategies to optimize energy use, reduce peak demand, and shift consumption patterns to align with renewable energy generation. Demand response programs, time-of-use pricing, smart appliances, and energy management systems enable consumers to adjust their electricity consumption in response to price signals or grid conditions.
- Off-Grid and Remote Applications:
- Decentralized energy systems are well-suited for off-grid and remote applications, such as rural electrification, island communities, and remote industrial sites, where grid connectivity is limited or unreliable. Off-grid systems combine renewable energy generation, energy storage, and efficient appliances to provide reliable power supply and improve energy access in underserved areas.
- Resilience and Energy Security:
- Decentralized energy systems enhance energy resilience and security by reducing dependence on centralized power sources and minimizing vulnerability to grid disruptions, natural disasters, and geopolitical risks. Distributed generation, localized storage, and grid-independent infrastructure improve energy reliability and ensure continuity of essential services during emergencies.
- Flexibility and Scalability:
- Decentralized energy systems offer flexibility and scalability to adapt to changing energy needs, technological advancements, and market dynamics. Modular components, standardized interfaces, and plug-and-play integration enable rapid deployment, expansion, and customization of decentralized energy infrastructure to meet diverse requirements and local conditions.
- Policy and Regulatory Support:
- Decentralized energy systems benefit from supportive policy frameworks, regulatory incentives, and market mechanisms that promote investment, innovation, and adoption of distributed energy resources. Policies such as net metering, feed-in tariffs, renewable energy targets, and energy efficiency standards create an enabling environment for decentralized energy development and deployment.
- Integration with Smart Grids:
- Decentralized energy systems complement smart grid infrastructure by enhancing grid flexibility, reliability, and efficiency through the integration of distributed energy resources, demand-side management, and advanced control systems. Interoperability standards, data exchange protocols, and grid-edge technologies facilitate seamless integration and optimization of decentralized energy assets within the broader energy ecosystem.
Decentralized energy systems represent a disruptive and transformative approach to energy production, distribution, and consumption, offering resilience, sustainability, and empowerment benefits to communities, businesses, and individuals. By embracing decentralization, local ownership, and collaborative innovation, decentralized energy systems contribute to the transition to a more resilient, equitable, and sustainable energy future.
Renewable Energy Policies:
Renewable energy policies are legislative, regulatory, and financial measures implemented by governments at the local, national, and international levels to promote the deployment, investment, and adoption of renewable energy technologies. These policies aim to accelerate the transition to a low-carbon energy system, mitigate climate change, enhance energy security, and stimulate economic growth. Here’s an in-depth exploration of renewable energy policies:
- Renewable Energy Targets:
- Renewable energy targets set specific goals and benchmarks for the share of renewable energy in the total energy mix within a given timeframe. These targets may include targets for renewable electricity generation, renewable energy capacity, or renewable energy consumption, aiming to increase the penetration of renewable energy sources and reduce reliance on fossil fuels.
- Feed-in Tariffs (FiTs):
- Feed-in tariffs are financial incentives that guarantee a fixed payment or premium price for renewable energy generated and fed into the grid. FiTs provide long-term revenue certainty and financial stability for renewable energy producers, incentivizing investment in renewable energy projects and promoting the rapid deployment of solar, wind, biomass, and other renewable technologies.
- Renewable Energy Production Incentives:
- Renewable energy production incentives provide direct financial support or tax incentives to renewable energy producers based on the amount of renewable energy generated or the environmental benefits achieved. Production incentives may take the form of production tax credits, investment tax credits, cash grants, or renewable energy certificates (RECs), encouraging renewable energy investment and deployment.
- Net Metering and Net Billing:
- Net metering and net billing policies allow renewable energy system owners to offset their electricity consumption with the electricity generated onsite and fed back into the grid. Excess energy generated is credited against future electricity bills or compensated at a predetermined rate, providing financial savings and encouraging distributed generation from rooftop solar PV systems and small wind turbines.
- Renewable Portfolio Standards (RPS):
- Renewable portfolio standards require utilities or electricity suppliers to procure a certain percentage of their electricity from renewable sources within a specified timeframe. RPS policies create demand for renewable energy, stimulate market growth, and diversify the energy portfolio, driving investment in renewable energy projects and supporting renewable energy industries.
- Green Power Purchasing Programs:
- Green power purchasing programs enable businesses, governments, and consumers to voluntarily purchase renewable energy certificates (RECs) or green power products to support renewable energy development and offset their carbon footprint. These programs provide consumers with the option to choose renewable energy sources and support renewable energy projects through their electricity purchases.
- Tax Incentives and Credits:
- Tax incentives and credits, such as investment tax credits (ITCs), production tax credits (PTCs), accelerated depreciation, and tax exemptions, reduce the cost of renewable energy investments and improve the financial viability of renewable energy projects. Tax incentives encourage private investment, stimulate economic growth, and create jobs in the renewable energy sector.
- Public Funding and Grants:
- Public funding and grants provide financial support for research, development, demonstration, and deployment of renewable energy technologies and infrastructure. Government grants, loans, and subsidies fund renewable energy projects, support technology innovation, and accelerate the commercialization of renewable energy solutions.
- Renewable Energy Auctions:
- Renewable energy auctions allocate contracts or capacity for renewable energy projects through competitive bidding processes, ensuring cost-effective deployment of renewable energy capacity and maximizing value for ratepayers. Auctions promote competition, drive down renewable energy prices, and facilitate market entry for renewable energy developers.
- Regulatory Reforms and Market Mechanisms:
- Regulatory reforms and market mechanisms, such as carbon pricing, emissions trading schemes, capacity markets, and integrated resource planning, internalize the external costs of fossil fuel-based energy generation and create a level playing field for renewable energy technologies. Market-based approaches incentivize emissions reductions, promote renewable energy investment, and foster innovation in clean energy solutions.
Renewable energy policies play a crucial role in driving the transition to a sustainable, low-carbon energy future by creating a supportive policy environment, providing financial incentives, and fostering market development and innovation. By aligning economic, environmental, and social objectives, renewable energy policies contribute to energy security, climate mitigation, and sustainable development goals, paving the way for a cleaner, greener, and more resilient energy system.
Renewable Energy Financing:
Renewable energy financing refers to the various mechanisms, instruments, and sources of funding used to support the development, deployment, and operation of renewable energy projects. Financing plays a critical role in overcoming the upfront costs, mitigating investment risks, and enabling the widespread adoption of renewable energy technologies. Here’s a detailed exploration of renewable energy financing:
- Project Finance:
- Project finance is a common financing mechanism used to fund large-scale renewable energy projects, such as utility-scale solar and wind farms. Project finance structures involve the creation of special purpose vehicles (SPVs) to ring-fence project assets and liabilities, allowing project developers to raise debt and equity financing based on the project’s cash flow and revenue streams.
- Debt Financing:
- Debt financing involves borrowing money from banks, financial institutions, or bond markets to fund renewable energy projects. Debt financing options include project loans, term loans, construction loans, and project bonds, which provide capital for project development, construction, and operation. Debt financing offers lower interest rates and longer repayment terms, reducing the cost of capital for renewable energy projects.
- Equity Financing:
- Equity financing involves raising capital from investors, venture capitalists, private equity firms, and institutional investors to finance renewable energy projects. Equity investors provide funding in exchange for ownership stakes or equity shares in the project, sharing in the project’s risks and returns. Equity financing provides capital for project development, construction, and operation, supporting project viability and growth.
- Tax Equity Financing:
- Tax equity financing involves monetizing renewable energy tax incentives, such as investment tax credits (ITCs) and production tax credits (PTCs), by attracting tax-equity investors with substantial tax liabilities. Tax-equity investors provide upfront funding to renewable energy projects in exchange for the rights to claim tax benefits generated by the project, helping project developers offset project costs and improve financial returns.
- Green Bonds and Climate Bonds:
- Green bonds and climate bonds are debt instruments issued by governments, corporations, or financial institutions to raise capital for renewable energy projects and other environmentally sustainable initiatives. Green bonds are earmarked for investments in renewable energy, energy efficiency, and climate adaptation projects, providing investors with opportunities to support green initiatives while generating financial returns.
- Crowdfunding and Community Investment:
- Crowdfunding platforms and community investment schemes enable individuals, communities, and small investors to contribute financially to renewable energy projects. Crowdfunding campaigns, peer-to-peer lending platforms, and community solar programs allow participants to invest directly in renewable energy projects, share in project benefits, and support local clean energy initiatives.
- Public-Private Partnerships (PPPs):
- Public-private partnerships involve collaboration between governments, private sector entities, and development finance institutions to finance, develop, and operate renewable energy projects. PPPs leverage public sector resources, private sector expertise, and innovative financing mechanisms to overcome investment barriers, mitigate risks, and accelerate the deployment of renewable energy infrastructure.
- Multilateral and Bilateral Financing:
- Multilateral development banks (MDBs) and bilateral development agencies provide financial assistance, concessional loans, and technical support to support renewable energy projects in developing countries and emerging markets. MDBs, such as the World Bank, Asian Development Bank (ADB), and African Development Bank (AfDB), finance renewable energy initiatives to promote sustainable development, poverty reduction, and climate resilience.
- Carbon Finance and Carbon Offsetting:
- Carbon finance mechanisms, such as carbon credits, carbon offsets, and emissions trading schemes, generate revenue streams for renewable energy projects by monetizing emissions reductions and environmental benefits. Carbon finance rewards renewable energy projects for displacing fossil fuel-based energy generation and reducing greenhouse gas emissions, providing additional revenue streams and financial incentives.
- Green Investment Funds and Venture Capital:
- Green investment funds and venture capital firms specialize in financing renewable energy startups, technology innovations, and early-stage projects. These funds provide seed capital, equity investments, and strategic support to renewable energy entrepreneurs and innovators, fostering technological advancements, market competitiveness, and industry growth in the renewable energy sector.
Renewable energy financing encompasses a wide range of financial instruments, mechanisms, and stakeholders that play a crucial role in driving investment, innovation, and deployment of renewable energy technologies. By mobilizing capital, reducing financial barriers, and unlocking new investment opportunities, renewable energy financing accelerates the transition to a clean, sustainable, and resilient energy future.
Renewable Energy Incentives:
Renewable energy incentives are policy measures, financial mechanisms, and regulatory frameworks designed to promote the adoption, deployment, and utilization of renewable energy technologies. These incentives aim to stimulate investment, drive market growth, and accelerate the transition to a low-carbon energy system by providing financial support, regulatory certainty, and market incentives for renewable energy development. Here’s a comprehensive exploration of renewable energy incentives:
- Investment Tax Credits (ITCs):
- Investment tax credits (ITCs) provide tax incentives to investors, developers, and owners of renewable energy projects based on eligible capital expenditures. ITCs allow taxpayers to offset a portion of their federal or state income taxes with tax credits equal to a percentage of qualified project costs, reducing the upfront cost of renewable energy investments and improving project economics.
- Production Tax Credits (PTCs):
- Production tax credits (PTCs) offer financial incentives to renewable energy producers, such as wind, biomass, geothermal, and hydropower projects, based on the amount of renewable energy generated and sold over a specified period. PTCs provide a fixed payment per kilowatt-hour (kWh) of electricity produced, encouraging renewable energy production and supporting project viability.
- Cash Grants and Rebates:
- Cash grants and rebates provide direct financial support to renewable energy project developers and owners in the form of cash payments or refunds for eligible project expenses. Government agencies, utilities, and incentive programs offer cash grants and rebates to offset project costs, stimulate investment, and accelerate the deployment of renewable energy technologies.
- Renewable Energy Tax Exemptions:
- Renewable energy tax exemptions exempt renewable energy equipment, installations, and investments from certain taxes, levies, or duties imposed by governments or regulatory authorities. Tax exemptions reduce the financial burden on renewable energy projects, lower the cost of renewable energy deployment, and improve project economics for investors and developers.
- Accelerated Depreciation:
- Accelerated depreciation allows renewable energy project owners to recover the capital costs of renewable energy assets more rapidly through accelerated depreciation schedules. Accelerated depreciation methods, such as bonus depreciation or modified accelerated cost recovery system (MACRS), enable project owners to deduct a larger portion of the asset’s value in the early years of its useful life, providing tax savings and improving project returns.
- Renewable Energy Grants and Incentive Programs:
- Renewable energy grants and incentive programs offer financial assistance, grants, or subsidies to support renewable energy projects, research, development, and deployment. Government agencies, non-profit organizations, and industry associations administer grant programs to fund renewable energy initiatives, technology innovation, and market transformation efforts.
- Net Energy Metering (NEM) Programs:
- Net energy metering (NEM) programs allow renewable energy system owners to offset their electricity consumption with onsite renewable energy generation and receive credit for excess energy exported to the grid. NEM programs enable renewable energy producers to reduce their electricity bills, receive compensation for surplus energy production, and promote distributed generation from rooftop solar PV systems.
- Renewable Energy Feed-in Tariffs (FiTs):
- Renewable energy feed-in tariffs (FiTs) guarantee a fixed payment or premium price for renewable energy generated and fed into the grid over a specified contract period. FiTs provide revenue certainty and long-term contracts for renewable energy producers, incentivizing investment in renewable energy projects and supporting the integration of renewable energy into the electricity grid.
- Renewable Energy Portfolio Standards (RPS):
- Renewable energy portfolio standards (RPS) mandate utilities or electricity suppliers to procure a certain percentage of their electricity from renewable sources, such as wind, solar, biomass, or geothermal, within a specified timeframe. RPS policies create demand for renewable energy, stimulate market growth, and diversify the energy portfolio, driving investment in renewable energy projects and supporting renewable energy industries.
- Green Power Purchasing Programs:
- Green power purchasing programs enable businesses, government agencies, and consumers to purchase renewable energy certificates (RECs) or green power products to support renewable energy development and offset their carbon footprint. Green power purchases provide financial support for renewable energy projects and promote market demand for renewable energy products and services.
Renewable energy incentives play a vital role in driving investment, stimulating market demand, and accelerating the deployment of renewable energy technologies worldwide. By providing financial support, regulatory certainty, and market incentives, renewable energy incentives facilitate the transition to a cleaner, more sustainable energy future while promoting economic growth, job creation, and environmental stewardship.
Renewable Energy Integration:
Renewable energy integration refers to the process of incorporating renewable energy sources, such as solar, wind, hydro, biomass, and geothermal, into the existing energy infrastructure to meet electricity demand, enhance grid stability, and reduce reliance on fossil fuels. Renewable energy integration involves overcoming technical, economic, regulatory, and institutional challenges to maximize the value and effectiveness of renewable energy resources within the energy system. Here’s a detailed exploration of renewable energy integration:
- Grid Interconnection and Transmission Planning:
- Renewable energy integration requires expanding and upgrading grid infrastructure to facilitate the connection of renewable energy projects to the electricity grid. Grid interconnection studies, transmission planning, and grid modernization efforts ensure reliable, efficient, and cost-effective integration of renewable energy resources, enabling electricity to be transmitted from areas of generation to areas of demand.
- Grid Stability and Power Quality:
- Renewable energy integration necessitates addressing grid stability and power quality issues associated with variable and intermittent renewable energy generation. Grid stability measures, such as frequency regulation, voltage control, and reactive power compensation, help manage fluctuations in renewable energy output and ensure the stability and reliability of the electricity grid.
- Energy Storage Solutions:
- Energy storage technologies, such as batteries, pumped hydro storage, compressed air energy storage, and thermal energy storage, play a critical role in renewable energy integration by storing excess energy during periods of high generation and releasing it during times of high demand or low renewable energy production. Energy storage solutions enhance grid flexibility, reliability, and resilience, enabling better integration of renewable energy resources.
- Demand-Side Management (DSM):
- Demand-side management (DSM) strategies optimize energy use, reduce peak demand, and align electricity consumption with renewable energy generation patterns. DSM measures, such as time-of-use pricing, demand response programs, smart grid technologies, and energy efficiency initiatives, enable consumers to adjust their electricity consumption in response to renewable energy availability, reducing the need for fossil fuel-based generation.
- Flexible Generation and Backup Power:
- Flexible generation technologies, such as natural gas-fired power plants, hydropower, and combined heat and power (CHP) systems, provide backup power and grid support services to complement variable renewable energy generation. Flexible generation assets help balance supply and demand, provide grid stability services, and ensure reliable electricity supply during periods of low renewable energy output or grid constraints.
- Smart Grid Technologies:
- Smart grid technologies, including advanced metering infrastructure (AMI), grid automation, real-time monitoring, and predictive analytics, enhance grid visibility, control, and management capabilities, facilitating the integration of renewable energy resources. Smart grid solutions enable real-time communication, grid optimization, and dynamic grid control to accommodate variable renewable energy generation and optimize grid operations.
- Hybrid Renewable Energy Systems:
- Hybrid renewable energy systems combine multiple renewable energy technologies, such as solar PV, wind turbines, and energy storage, to maximize energy generation, improve system reliability, and optimize resource utilization. Hybrid systems leverage complementary renewable energy sources and energy storage technologies to mitigate intermittency, enhance system efficiency, and increase renewable energy penetration.
- Virtual Power Plants (VPPs):
- Virtual power plants (VPPs) aggregate and manage distributed energy resources, including rooftop solar PV systems, battery storage, electric vehicles (EVs), and demand response assets, to provide grid services, optimize energy dispatch, and support renewable energy integration. VPPs enable coordinated control and optimization of distributed energy assets to balance supply and demand and enhance grid flexibility.
- Interconnection Standards and Grid Codes:
- Interconnection standards and grid codes establish technical requirements, performance criteria, and operational protocols for connecting renewable energy projects to the electricity grid. Grid codes ensure the safe, reliable, and seamless integration of renewable energy resources by defining grid connection requirements, voltage and frequency tolerance limits, and grid performance standards for renewable energy generators.
- Market Design and Regulatory Reforms:
- Market design and regulatory reforms play a crucial role in facilitating renewable energy integration by creating market mechanisms, pricing signals, and regulatory frameworks that incentivize renewable energy deployment and support grid flexibility. Market reforms, such as capacity markets, ancillary services markets, and carbon pricing, promote renewable energy investment, grid resilience, and energy transition objectives.
Renewable energy integration requires a holistic approach that encompasses grid infrastructure upgrades, energy storage deployment, demand-side management, and regulatory reforms to facilitate the seamless integration of renewable energy resources into the electricity grid. By embracing technological innovation, policy support, and collaborative stakeholder engagement, renewable energy integration enables the transition to a cleaner, more sustainable energy future while ensuring grid reliability, stability, and resilience.
Renewable Energy Grid Parity:
Renewable energy grid parity refers to the point at which the cost of generating electricity from renewable energy sources becomes comparable to or cheaper than electricity generated from conventional fossil fuel sources, without the need for subsidies or incentives. Achieving grid parity is a significant milestone for renewable energy deployment as it enhances the competitiveness, scalability, and attractiveness of renewable energy technologies in the energy market. Here’s a comprehensive exploration of renewable energy grid parity:
- Cost Reduction Trends:
- Renewable energy grid parity has been driven by significant cost reductions in renewable energy technologies, particularly solar photovoltaics (PV) and wind power, resulting from technological advancements, economies of scale, manufacturing efficiencies, and innovation in project development and financing.
- Declining Solar PV Costs:
- The rapid decline in solar PV costs, attributed to improvements in solar cell efficiency, economies of scale in manufacturing, and reductions in installation and balance-of-system costs, has led to widespread grid parity for solar PV in many regions worldwide, enabling solar energy to compete with conventional electricity sources on a cost-competitive basis.
- Competitive Wind Power Prices:
- Wind power has achieved grid parity in numerous regions due to falling turbine costs, increased turbine efficiency, and favorable wind resource conditions. Competitive wind power prices, coupled with long-term power purchase agreements (PPAs) and government incentives, have driven the expansion of wind energy deployment and its integration into the electricity grid.
- Levelized Cost of Electricity (LCOE):
- The levelized cost of electricity (LCOE) serves as a key metric for assessing the economic competitiveness of renewable energy technologies relative to conventional fossil fuel sources. As renewable energy costs continue to decline, LCOE calculations indicate that renewable energy technologies, such as solar PV, onshore and offshore wind, biomass, and geothermal, are increasingly cost-competitive with coal, natural gas, and nuclear power plants.
- Economies of Scale and Technological Innovation:
- Economies of scale, technological innovation, and learning curves have driven down the capital costs and operational expenses of renewable energy projects, making them more economically viable and competitive in the energy market. Advances in renewable energy technology, materials science, manufacturing processes, and project development practices have accelerated the pace of cost reduction and grid parity attainment.
- Levelized Cost Reduction Strategies:
- Levelized cost reduction strategies, such as auction mechanisms, competitive procurement processes, project finance innovations, and streamlined permitting and regulatory frameworks, have contributed to cost reductions and grid parity achievement for renewable energy projects. Competitive bidding processes and market competition have driven down renewable energy prices, resulting in more affordable and accessible clean energy solutions.
- Policy Support and Market Incentives:
- Policy support, regulatory frameworks, and market incentives, including renewable energy targets, feed-in tariffs, tax incentives, renewable portfolio standards (RPS), and carbon pricing mechanisms, have played a pivotal role in accelerating renewable energy deployment and achieving grid parity. Government policies and market mechanisms create a conducive environment for renewable energy investment, innovation, and market competitiveness.
- Integrated Energy Systems:
- Integrated energy systems, combining renewable energy generation, energy storage, demand-side management, smart grid technologies, and flexible generation assets, enable the optimization of renewable energy utilization and grid integration. Integrated energy systems enhance grid stability, resilience, and reliability while maximizing the value of renewable energy resources and minimizing reliance on fossil fuels.
- Market Competitiveness and Investment Opportunities:
- Renewable energy grid parity enhances the market competitiveness of renewable energy technologies and creates attractive investment opportunities for developers, investors, utilities, and consumers. Grid parity-driven investments in renewable energy projects contribute to job creation, economic growth, energy security, and environmental sustainability, driving the transition to a clean energy future.
- Global Energy Transition:
- Renewable energy grid parity is a catalyst for the global energy transition towards a low-carbon, sustainable energy system. Achieving grid parity for renewable energy technologies accelerates the phase-out of fossil fuels, reduces greenhouse gas emissions, mitigates climate change impacts, and fosters energy independence, resilience, and prosperity for present and future generations.
Renewable energy grid parity marks a transformative shift in the energy landscape, signaling the economic viability, scalability, and mainstream adoption of renewable energy technologies as competitive alternatives to conventional fossil fuel-based electricity generation. By harnessing the power of innovation, investment, and policy support, renewable energy grid parity paves the way for a more resilient, equitable, and sustainable energy future for all.
Renewable Energy Auctions:
Renewable energy auctions are competitive procurement processes used by governments, utilities, and energy regulators to solicit bids from renewable energy developers for the supply of electricity from renewable energy projects. Auctions aim to efficiently allocate renewable energy capacity, drive down electricity prices, and accelerate the deployment of renewable energy technologies by selecting projects based on cost-effectiveness, competitiveness, and technical feasibility. Here’s an in-depth exploration of renewable energy auctions:
- Objective and Purpose:
- The primary objective of renewable energy auctions is to procure renewable electricity generation capacity at the lowest possible cost while ensuring a reliable and sustainable energy supply. Auctions facilitate the transparent and competitive selection of renewable energy projects, driving efficiency, innovation, and market competition in the renewable energy sector.
- Auction Design and Mechanisms:
- Renewable energy auctions employ various design elements and mechanisms, including sealed-bid auctions, descending-clock auctions, pay-as-bid auctions, and hybrid auction formats, to solicit bids from project developers and determine the allocation of renewable energy contracts. Auction parameters, such as capacity targets, contract terms, bidding rules, and eligibility criteria, are tailored to specific market conditions and policy objectives.
- Technology Neutrality and Eligibility:
- Renewable energy auctions may adopt technology-neutral or technology-specific approaches, allowing a wide range of renewable energy technologies, such as solar PV, wind power, biomass, hydroelectricity, and geothermal energy, to participate based on their respective technical and economic characteristics. Eligibility criteria, such as project size, technology maturity, and grid connection requirements, ensure fair competition and project viability.
- Bid Evaluation and Selection Criteria:
- Bids submitted in renewable energy auctions are evaluated based on predetermined selection criteria, including bid prices, project capacity, technical specifications, financial viability, grid integration capabilities, environmental impacts, and local content requirements. Evaluation committees or independent experts assess bid proposals to determine the most competitive and suitable projects for awarding contracts.
- Price Discovery and Clearing Price:
- Renewable energy auctions facilitate price discovery by soliciting bids from developers at different price levels and selecting projects based on the lowest bid prices or the clearing price, which represents the maximum price accepted for renewable energy contracts. Price signals generated through auctions reflect market conditions, technology costs, and policy support levels, guiding investment decisions and market expectations.
- Contractual Arrangements and Power Purchase Agreements (PPAs):
- Winning bidders in renewable energy auctions enter into long-term power purchase agreements (PPAs) or feed-in tariffs (FiTs) with utilities or off-takers to sell electricity generated from renewable energy projects at predetermined prices over the contract duration. PPAs provide revenue certainty and financial stability for project developers, enabling project financing, construction, and operation.
- Risk Mitigation and Financial Guarantees:
- Renewable energy auctions may incorporate risk mitigation measures, such as performance guarantees, financial securities, bid bonds, and project insurance, to ensure project developers’ compliance with contractual obligations and safeguard public interests. Financial guarantees mitigate project risks, enhance investor confidence, and protect against project delays or failures.
- Market Competition and Cost Reduction:
- Competitive bidding in renewable energy auctions fosters market competition among project developers, driving down renewable energy prices and reducing the levelized cost of electricity (LCOE) for renewable energy projects. Price competition incentivizes cost reduction efforts, technological innovation, and efficiency improvements across the renewable energy value chain, benefiting consumers and the environment.
- Grid Integration and System Flexibility:
- Renewable energy auctions consider grid integration requirements and system flexibility to ensure the reliable and stable integration of renewable energy projects into the electricity grid. Grid-friendly renewable energy projects with flexible dispatch capabilities, energy storage integration, and grid support services are prioritized in auctions to enhance grid reliability and resilience.
- Policy Support and Market Stability:
- Renewable energy auctions are often supported by favorable policy frameworks, renewable energy targets, carbon pricing mechanisms, and regulatory incentives to create a stable and conducive investment environment for renewable energy development. Long-term policy commitments and market stability contribute to investor confidence, project bankability, and sustainable growth of the renewable energy sector.
Renewable energy auctions have emerged as a preferred mechanism for procuring renewable electricity generation capacity, driving down costs, and expanding renewable energy deployment worldwide. By promoting transparency, competition, and efficiency in project selection and contract allocation, renewable energy auctions play a vital role in advancing the transition to a cleaner, more sustainable energy future.
Renewable Energy Certificates (RECs):
Renewable Energy Certificates (RECs), also known as Renewable Energy Credits or Green Certificates, are tradable instruments that represent the environmental attributes and benefits associated with generating electricity from renewable energy sources. RECs serve as a market-based mechanism to incentivize and track renewable energy production, facilitate renewable energy procurement, and support renewable energy goals and initiatives. Here’s an in-depth exploration of Renewable Energy Certificates:
- Environmental Attributes:
- RECs represent the environmental attributes of renewable energy generation, including the avoidance of greenhouse gas emissions, reduction of air pollutants, conservation of natural resources, and support for sustainable energy production practices. Each REC certifies that one megawatt-hour (MWh) of electricity was generated from a renewable energy source and fed into the grid.
- Generation and Certification Process:
- RECs are generated when renewable energy facilities, such as solar photovoltaic (PV) systems, wind turbines, biomass plants, hydroelectric dams, and geothermal power plants, produce electricity from renewable sources. Generation facilities are certified by regulatory authorities or independent certification bodies to ensure compliance with renewable energy standards and eligibility criteria.
- Separation of Attributes:
- RECs decouple the physical electricity generation from its environmental attributes, allowing renewable energy benefits to be separately traded, sold, or transferred independent of the underlying electricity. This separation enables electricity consumers, utilities, corporations, and governments to support renewable energy development and claim environmental benefits without physically purchasing renewable electricity.
- REC Tracking and Registry Systems:
- REC tracking and registry systems, managed by regulatory agencies, industry associations, or independent organizations, record the issuance, transfer, and retirement of RECs throughout their lifecycle. REC tracking systems provide transparency, accountability, and integrity in REC transactions, ensuring the accurate representation and verification of renewable energy attributes.
- Market Transactions and Trading:
- RECs are traded in voluntary and compliance markets, where buyers and sellers participate in REC transactions to meet renewable energy goals, compliance obligations, or sustainability targets. REC trading platforms, brokers, and exchanges facilitate the buying and selling of RECs, allowing market participants to acquire renewable energy attributes and demonstrate environmental stewardship.
- Voluntary Renewable Energy Markets:
- In voluntary markets, organizations, businesses, institutions, and individuals purchase RECs voluntarily to support renewable energy development, reduce carbon footprints, and promote sustainability initiatives. Voluntary REC buyers use RECs to offset their electricity consumption, demonstrate environmental leadership, and communicate renewable energy commitments to stakeholders.
- Compliance and Renewable Portfolio Standards (RPS):
- In compliance markets, utilities, electricity suppliers, and energy retailers purchase RECs to comply with renewable portfolio standards (RPS), renewable energy mandates, or regulatory requirements set by government agencies or legislative bodies. Compliance REC purchases ensure that a specified percentage of electricity sold comes from eligible renewable sources.
- Emission Reduction Claims and Green Marketing:
- RECs enable organizations to make credible claims about their renewable energy usage, carbon emissions reductions, and environmental impact mitigation. Businesses, brands, and institutions use RECs to substantiate green marketing initiatives, communicate sustainability achievements, and differentiate their products or services in the marketplace.
- Price Determination and Valuation:
- REC prices are influenced by supply and demand dynamics, renewable energy market conditions, policy support, renewable energy generation costs, and environmental regulations. The value of RECs reflects the societal and environmental benefits of renewable energy generation and varies based on market fundamentals and regulatory frameworks.
- Renewable Energy Impact and Additionality:
- RECs contribute to the growth of renewable energy capacity, investment in renewable energy projects, and the transition to a low-carbon energy system by providing financial incentives and market signals for renewable energy development. The purchase and retirement of RECs demonstrate commitment to renewable energy goals and support the expansion of clean energy infrastructure.
Renewable Energy Certificates play a crucial role in advancing renewable energy deployment, incentivizing renewable energy investment, and accelerating the transition to a sustainable energy future. By enabling stakeholders to quantify, trade, and monetize renewable energy attributes, RECs promote market-driven solutions, environmental stewardship, and renewable energy innovation.
Renewable Energy Financing:
Renewable energy financing encompasses the diverse array of financial mechanisms, instruments, and strategies used to fund the development, construction, and operation of renewable energy projects. Financing renewable energy projects involves mobilizing capital, managing financial risks, and structuring investments to support the deployment of solar, wind, hydro, biomass, geothermal, and other renewable energy technologies. Here’s an in-depth exploration of renewable energy financing:
- Project Development Capital:
- Renewable energy project developers require capital to finance the development phase, including site identification, feasibility studies, permitting, environmental assessments, and interconnection studies. Project development capital covers upfront costs associated with project planning, design, and regulatory compliance before construction commences.
- Construction Financing:
- Construction financing provides the necessary funds to build renewable energy infrastructure, including solar PV arrays, wind farms, biomass facilities, and hydroelectric dams. Construction loans, project finance structures, and equity investments are used to cover equipment procurement, engineering, procurement, and construction (EPC) contracts, labor, and installation costs.
- Debt Financing:
- Debt financing plays a crucial role in funding renewable energy projects, with project developers leveraging loans, bonds, and other debt instruments to raise capital for project development and construction. Debt financing options include commercial loans, project finance debt, green bonds, and structured finance products tailored to renewable energy assets.
- Equity Investment:
- Equity investment involves raising capital from investors, venture capitalists, private equity firms, and institutional funds to finance renewable energy projects and acquire ownership stakes in project assets. Equity investors provide capital in exchange for ownership shares, dividends, or capital appreciation, sharing in the risks and returns of renewable energy investments.
- Tax Equity Financing:
- Tax equity financing utilizes federal, state, and local tax incentives, such as investment tax credits (ITCs), production tax credits (PTCs), and accelerated depreciation allowances, to attract investment capital from tax equity investors. Tax equity investors monetize renewable energy tax benefits by investing in renewable energy projects and claiming tax credits or deductions over time.
- Power Purchase Agreements (PPAs):
- Power purchase agreements (PPAs) enable renewable energy project developers to secure long-term revenue streams by selling electricity generated from renewable sources to off-takers, such as utilities, corporations, municipalities, and government agencies, under fixed-price or indexed-price contracts. PPAs provide revenue certainty and mitigate market risks for project financiers.
- Asset-Backed Securities (ABS):
- Asset-backed securities (ABS) are financial instruments backed by the cash flows generated from renewable energy assets, such as solar installations, wind turbines, and biomass facilities. ABS issuances securitize renewable energy project revenues, allowing investors to purchase bonds or securities tied to the performance of renewable energy projects.
- Green Bonds and Sustainable Finance:
- Green bonds and sustainable finance instruments raise capital for renewable energy projects and environmentally sustainable initiatives by issuing bonds, loans, or financial products with specific environmental or social impact objectives. Green bonds attract socially responsible investors and institutional funds seeking to support renewable energy development and climate mitigation efforts.
- Project Finance Structures:
- Project finance structures, such as limited recourse financing, non-recourse financing, and special purpose vehicles (SPVs), allocate project risks and liabilities among project stakeholders, lenders, and investors. Project finance arrangements provide lenders with security interests in project assets and cash flows while shielding sponsors from project-related liabilities.
- Renewable Energy Investment Funds:
- Renewable energy investment funds pool capital from institutional investors, pension funds, sovereign wealth funds, and accredited investors to invest in diversified portfolios of renewable energy projects, including solar, wind, hydro, and biomass assets. Renewable energy funds provide access to professionally managed investment opportunities and portfolio diversification in the renewable energy sector.
Renewable energy financing mechanisms play a critical role in overcoming financial barriers, attracting investment capital, and accelerating the deployment of renewable energy projects worldwide. By leveraging innovative financing structures, tax incentives, and market mechanisms, renewable energy financing supports the transition to a clean, sustainable, and resilient energy future.
Renewable Energy Tax Incentives:
Renewable energy tax incentives are government policies and financial mechanisms designed to stimulate investment in renewable energy projects, promote renewable energy deployment, and accelerate the transition to a low-carbon energy system. These incentives provide tax credits, deductions, exemptions, and other financial benefits to renewable energy developers, investors, and end-users to offset project costs, improve project economics, and encourage renewable energy adoption. Here’s an in-depth exploration of renewable energy tax incentives:
- Investment Tax Credit (ITC):
- The Investment Tax Credit (ITC) provides a federal tax credit to eligible renewable energy projects, including solar, wind, geothermal, biomass, and fuel cell systems, based on a percentage of eligible project costs. The ITC allows project developers and investors to claim a tax credit equal to a specified percentage (e.g., 26% for solar and 60% for fuel cells) of qualified project expenditures, reducing federal tax liabilities dollar-for-dollar.
- Production Tax Credit (PTC):
- The Production Tax Credit (PTC) offers a federal tax credit for electricity generated from qualified renewable energy sources, such as wind, biomass, geothermal, hydroelectric, and marine energy systems, over a specified period (typically 10 years). The PTC provides a per-kilowatt-hour (kWh) tax credit for electricity produced and sold by eligible renewable energy facilities, incentivizing renewable energy production and investment.
Renewable Electricity Production Incentives:
- Renewable electricity production incentives, also known as feed-in tariffs (FiTs) or renewable energy payments, provide financial incentives to renewable energy generators for every unit of electricity produced and fed into the grid. These incentives, implemented at the state or local level, guarantee fixed or premium prices for renewable electricity generation, offering revenue certainty and long-term support for renewable energy projects.
- Accelerated Depreciation Allowance:
- Accelerated depreciation allowances allow renewable energy project owners to depreciate the capital costs of renewable energy assets over a shorter timeframe, accelerating tax deductions and reducing taxable income. Accelerated depreciation methods, such as the Modified Accelerated Cost Recovery System (MACRS), enable faster recovery of investment costs for renewable energy projects, enhancing project economics and cash flow.
- Energy Investment Tax Credit (EITC):
- The Energy Investment Tax Credit (EITC) provides tax credits for investments in energy-efficient equipment and renewable energy technologies, such as energy storage systems, combined heat and power (CHP) systems, and energy conservation measures. The EITC incentivizes energy efficiency upgrades and renewable energy investments in residential, commercial, industrial, and institutional buildings.
- Renewable Energy Bonds (REBs):
- Renewable Energy Bonds (REBs), including Clean Renewable Energy Bonds (CREBs) and Qualified Energy Conservation Bonds (QECBs), offer tax-advantaged financing options for renewable energy projects and energy efficiency initiatives. REBs provide low-cost or zero-interest loans, grants, or subsidies to eligible borrowers, such as municipalities, tribal governments, public agencies, and nonprofit organizations, to finance renewable energy projects and green infrastructure improvements.
- Tax-Exempt Financing:
- Tax-exempt financing allows certain types of renewable energy projects, such as qualified small-scale renewable energy systems and community-owned renewable energy projects, to access tax-exempt bonds or financing arrangements. Tax-exempt financing lowers borrowing costs, reduces financing expenses, and makes renewable energy investments more attractive to investors and project developers.
- Renewable Energy Grants and Rebates:
- Renewable energy grants and rebates provide direct financial assistance to renewable energy project developers, homeowners, businesses, and agricultural producers to offset the upfront costs of renewable energy installations. Government agencies, utilities, and nonprofit organizations offer grants, rebates, and financial incentives for solar PV systems, wind turbines, geothermal heat pumps, and other renewable energy technologies.
- Carbon Capture Tax Credits:
- Carbon capture tax credits incentivize the deployment of carbon capture, utilization, and storage (CCUS) technologies to reduce greenhouse gas emissions from industrial facilities, power plants, and other stationary sources. Carbon capture tax credits provide financial incentives for capturing and sequestering carbon dioxide (CO2) emissions, contributing to climate change mitigation efforts and promoting clean energy innovation.
- Renewable Energy Research and Development (R&D) Tax Credits:
- Renewable energy research and development (R&D) tax credits support investment in renewable energy technology innovation, research, and commercialization activities. R&D tax credits provide tax incentives to companies, universities, and research institutions engaged in renewable energy research, development, and demonstration projects, fostering technological advancements and industry growth.
Renewable energy tax incentives play a critical role in driving investment, stimulating economic growth, and advancing renewable energy adoption. By reducing financial barriers, improving project economics, and incentivizing clean energy investments, these tax policies contribute to the transition to a sustainable, low-carbon energy future.
EMS Power Machines
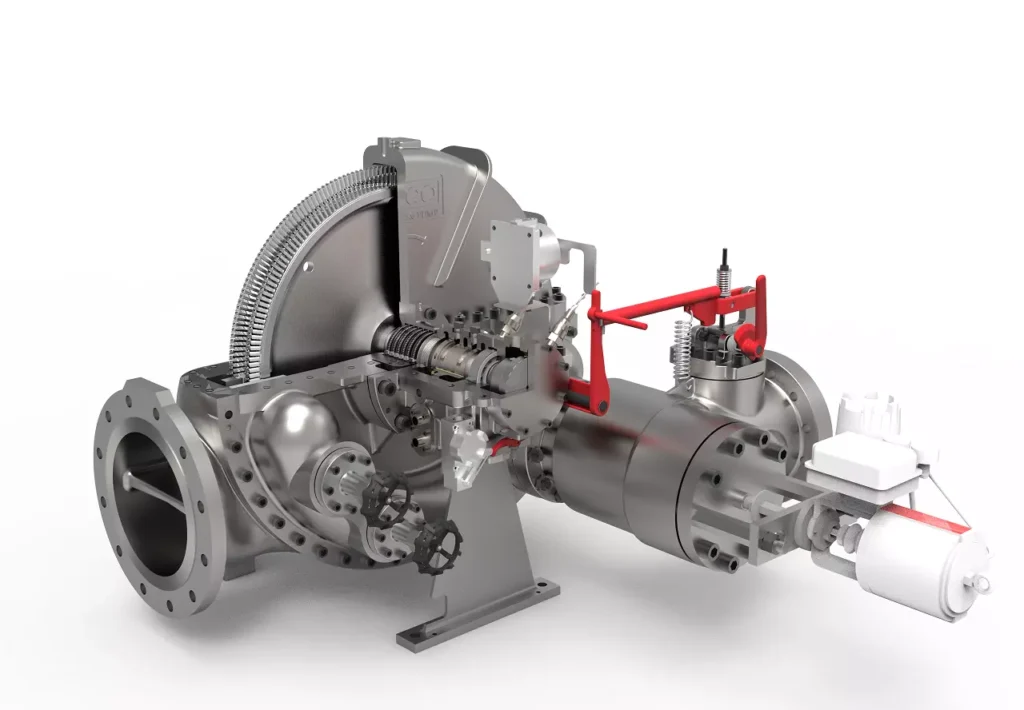
We design, manufacture and assembly Power Machines such as – diesel generators, electric motors, vibration motors, pumps, steam engines and steam turbines
EMS Power Machines is a global power engineering company, one of the five world leaders in the industry in terms of installed equipment. The companies included in the company have been operating in the energy market for more than 60 years.
EMS Power Machines manufactures steam turbines, gas turbines, hydroelectric turbines, generators, and other power equipment for thermal, nuclear, and hydroelectric power plants, as well as for various industries, transport, and marine energy.
EMS Power Machines is a major player in the global power industry, and its equipment is used in power plants all over the world. The company has a strong track record of innovation, and it is constantly developing new and improved technologies.
Here are some examples of Power Machines’ products and services:
- Steam turbines for thermal and nuclear power plants
- Gas turbines for combined cycle power plants and industrial applications
- Hydroelectric turbines for hydroelectric power plants
- Generators for all types of power plants
- Boilers for thermal power plants
- Condensers for thermal power plants
- Reheaters for thermal power plants
- Air preheaters for thermal power plants
- Feedwater pumps for thermal power plants
- Control systems for power plants
- Maintenance and repair services for power plants
EMS Power Machines is committed to providing its customers with high-quality products and services. The company has a strong reputation for reliability and innovation. Power Machines is a leading provider of power equipment and services, and it plays a vital role in the global power industry.
EMS Power Machines, which began in 1961 as a small factory of electric motors, has become a leading global supplier of electronic products for different segments. The search for excellence has resulted in the diversification of the business, adding to the electric motors products which provide from power generation to more efficient means of use.