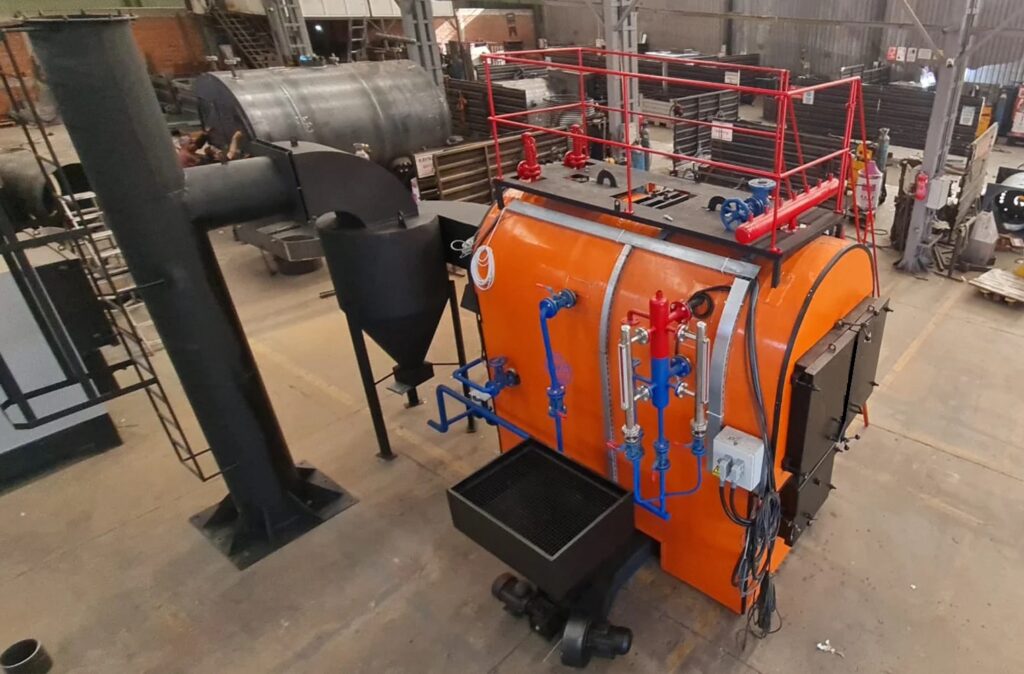
Wood Stove Electricity Generator: The steam engine is one of the most transformative inventions in human history. It has played a critical role in the development of the industrial world and is credited with fueling the first Industrial Revolution. This complex mechanism works by converting the energy stored in steam into mechanical power, which can then be used to perform various types of work such as driving machinery, locomotives, or powering factories. Understanding how the steam engine functions, its historical impact, and its modern applications is essential for appreciating its significance.
The Invention and Evolution of the Steam Engine
The concept of harnessing steam power dates back to ancient times, with early examples like Hero of Alexandria’s aeolipile—a simple device that spun when steam was emitted. However, it wasn’t until the late 17th and early 18th centuries that steam engines were developed in a practical sense. The credit for the first practical steam engine is often given to Thomas Savery, who in 1698 patented a machine to pump water out of mines using steam pressure. This was followed by Thomas Newcomen’s atmospheric engine in 1712, which improved on Savery’s design and became the first widely used steam engine for industrial purposes.
The real leap in steam technology came with James Watt’s improvements in the 1760s and 1770s. Watt’s innovations, including the separate condenser, dramatically increased the efficiency of steam engines and paved the way for their widespread adoption in factories, mines, and transportation. Watt’s engine was not only more efficient, but it could be used for a broader range of applications, making it the engine of choice for industrial enterprises during the early stages of the Industrial Revolution.
How a Steam Engine Works
At its core, a steam engine converts the energy stored in steam into mechanical power. The process begins by heating water in a boiler until it turns into steam. This steam is then directed into a cylinder where it expands, pushing a piston or turning a turbine. The movement of the piston can be connected to a flywheel or other mechanical systems to perform work, such as turning wheels or powering machinery.
There are different types of steam engines, including reciprocating engines, which use pistons, and steam turbines, which use spinning blades to convert steam power into mechanical energy. Reciprocating engines, the earliest form of steam engines, rely on the back-and-forth motion of pistons to drive a crankshaft and produce mechanical movement. These engines were widely used in locomotives, ships, and early factories.
Steam turbines, on the other hand, rely on the continuous flow of steam to spin a rotor. These engines are much more efficient than reciprocating engines and are used in modern power plants, including nuclear power stations, to generate electricity. The turbine is spun by the high-pressure steam, and as the steam passes over the blades, it causes the rotor to turn, generating mechanical power.
Applications of Steam Engines
Steam engines revolutionized many industries by providing a reliable and powerful source of mechanical energy. In transportation, steam engines were used in locomotives and ships, facilitating faster and more efficient travel over long distances. Before the development of the steam engine, transportation relied on human or animal power, or wind in the case of ships. The steam engine changed this dynamic, allowing for quicker movement of goods and people, and making global trade and communication more feasible.
In manufacturing, steam engines provided the power needed to drive machinery, leading to a surge in factory production. Textiles, mining, and iron production were just a few of the industries that saw dramatic improvements in productivity due to steam-powered machinery. Steam engines also played a vital role in agriculture by powering threshers and other equipment, increasing food production and reducing the labor required for farming.
The Role of Steam Engines in the Industrial Revolution
The steam engine is often cited as the driving force behind the Industrial Revolution. The advent of steam-powered machinery allowed for mass production in factories, which significantly boosted economic growth. Factories could now operate more efficiently, producing goods at an unprecedented scale. Steam engines were also central to the expansion of the railroads, which became the backbone of industrial economies, enabling the transport of raw materials and finished goods across vast distances.
In addition to driving economic growth, steam engines contributed to societal change. As factories grew in number and scale, urban centers expanded, and more people moved from rural areas to cities in search of work. This migration contributed to the rise of a new working class and the shift from agrarian economies to industrialized ones.
Modern Steam Engines
Though the age of the steam engine as a primary source of mechanical power has passed, it remains an important technology in certain applications. Steam turbines, for example, are still used in many power plants to generate electricity. The principles of steam power are also used in some modern propulsion systems, such as those found in nuclear-powered ships and submarines.
In the realm of heritage and tourism, steam engines still enjoy a considerable amount of attention. Steam-powered locomotives are preserved and operated by enthusiasts around the world, and many museums dedicate significant space to steam technology and its impact on industrial development.
The Decline of Steam Power
While steam engines were dominant during the 18th and 19th centuries, their use began to decline in the early 20th century. Internal combustion engines and electric motors, which are more efficient and easier to maintain, started to replace steam engines in many applications. The internal combustion engine, in particular, offered a more compact and efficient means of generating mechanical power, making it ideal for automobiles and smaller machinery. Likewise, the electrification of industries and transportation systems led to a gradual decline in the reliance on steam power.
However, the principles of steam power remain in use, particularly in power generation. Modern steam turbines are used in a wide range of applications, from fossil fuel power plants to nuclear reactors. In these systems, steam is generated by heating water using coal, natural gas, or nuclear fission, and the steam is then used to drive turbines connected to electric generators. This method of power generation is highly efficient and remains one of the primary ways electricity is produced around the world.
Environmental Impact of Steam Engines
One of the downsides of early steam engines was their environmental impact. Many steam engines relied on coal as a fuel source, which led to high levels of air pollution. The burning of coal releases large amounts of carbon dioxide and other harmful gases into the atmosphere, contributing to climate change and health problems in industrial areas. In the 19th and early 20th centuries, cities with large numbers of steam-powered factories, like London and Manchester, experienced significant smog and poor air quality as a result of coal-burning steam engines.
As environmental awareness has grown, the use of steam engines powered by fossil fuels has declined. In modern power plants, efforts have been made to reduce the environmental impact of steam turbines by using cleaner energy sources, such as natural gas, nuclear power, and renewable sources like geothermal and solar-thermal energy.
Conclusion
The steam engine was a revolutionary invention that changed the course of history. It was the driving force behind the Industrial Revolution, transforming industries, transportation, and society. While its use in everyday machinery and transportation has largely been replaced by more modern technologies, steam engines remain an important part of our energy infrastructure and a symbol of human innovation. Their legacy continues in the modern power plants that generate electricity using steam turbines, demonstrating that the principles behind this transformative invention still play a role in the world today.
Self Sustaining Power Source
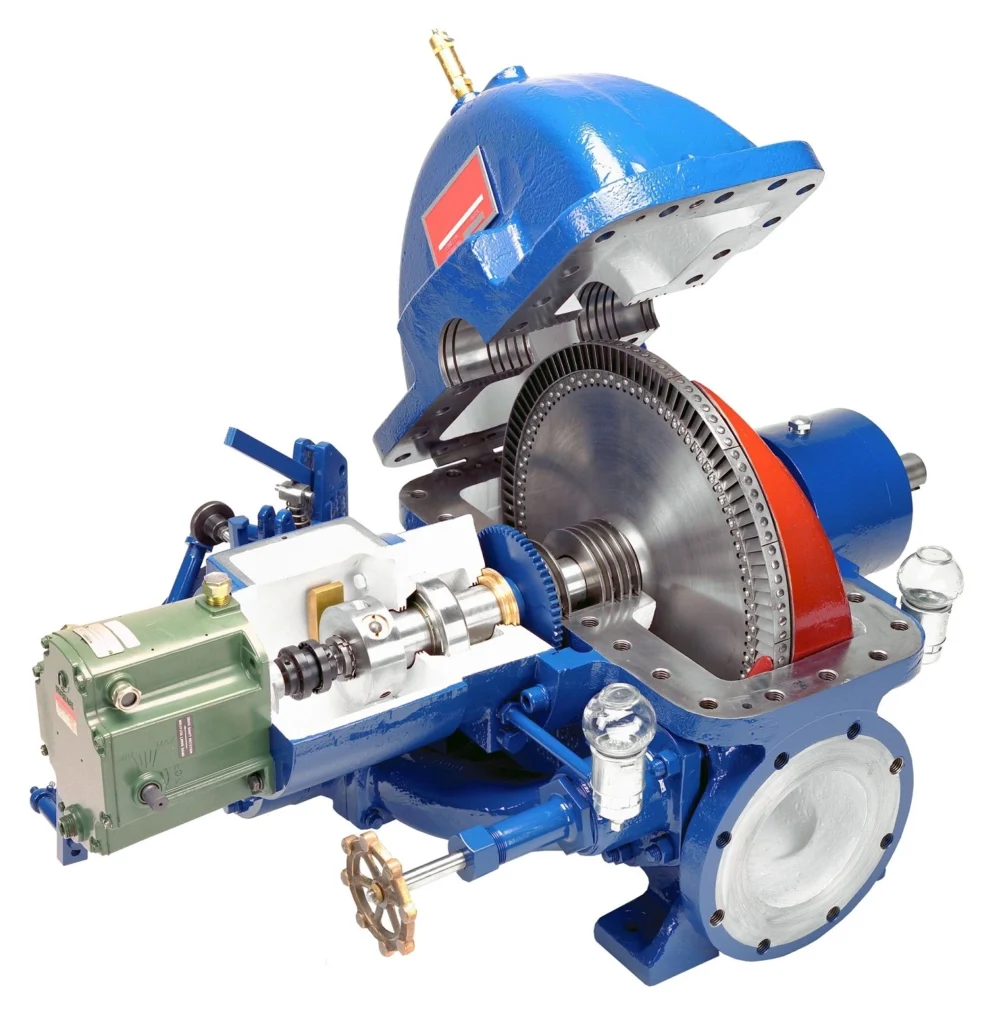
The concept of a self-sustaining power source, sometimes referred to as perpetual or autonomous power generation, is highly sought after, especially in an age where energy efficiency, sustainability, and independence from centralized power grids are becoming paramount concerns. A self-sustaining power source is essentially a system that can generate energy independently, continuously replenishing its fuel or energy supply without the need for external input or ongoing consumption of finite resources like fossil fuels. While achieving true perpetuity in power generation is largely considered impossible due to the laws of thermodynamics, various technologies and systems exist that come close by maximizing efficiency, utilizing renewable resources, or operating for extended periods with minimal input.
This section explores different types of self-sustaining power sources, their underlying principles, current technologies, and their significance in achieving energy independence, especially in off-grid settings.
Principles Behind Self-Sustaining Power Sources
The primary principle behind any self-sustaining power source is energy autonomy. Energy autonomy means that the system is designed in such a way that it can operate independently without needing continuous fuel replenishment from external sources, such as coal, natural gas, or oil. This independence can be achieved through several approaches:
- Renewable Energy: By using renewable resources such as solar, wind, or hydro, a power system can theoretically continue to operate indefinitely as long as the natural resource is available. Renewable energy systems like solar panels or wind turbines do not consume fuel but instead convert naturally available energy into electricity.
- Closed-Loop Systems: These systems aim to recycle energy or fuel within the system itself, thereby reducing the need for external inputs. Examples include cogeneration systems or biomass gasification systems, which capture and reuse byproducts of energy production to sustain the system’s operations.
- Energy Storage: Some self-sustaining systems rely on energy storage devices like batteries or capacitors to store excess energy produced during peak conditions (e.g., sunny or windy periods) and release it when production is low. The key is designing a system where energy storage and generation balance each other out.
- High-Efficiency Systems: Another key component of self-sustaining power systems is maximizing efficiency so that very little energy is lost during generation, storage, and consumption. Super-efficient designs allow systems to stretch the energy they produce, reducing their dependence on external inputs.
Key Types of Self-Sustaining Power Sources
1. Solar Power Systems with Energy Storage
Solar energy is one of the most popular forms of renewable energy used in self-sustaining systems. The main components of a solar power system are solar panels, which convert sunlight into electricity, and an energy storage system, typically batteries, that store excess power generated during the day for use at night or during cloudy periods.
A solar power system can be designed to operate off-grid, providing a household or facility with a self-sustaining power source. The battery storage system plays a crucial role in ensuring the system’s sustainability, allowing the system to operate independently of the local power grid.
With advancements in energy storage technologies, such as lithium-ion batteries, solar power systems are becoming more efficient and reliable. Solar energy is inherently sustainable since it relies on the sun, a virtually inexhaustible energy source, making solar power systems a key part of the global transition to renewable energy.
However, the limitations of solar energy—such as dependence on weather conditions and daylight—mean that without a robust energy storage solution, it cannot be entirely self-sustaining. Improvements in storage technology and hybrid systems combining solar with other renewable sources (e.g., wind or hydro) are helping to mitigate these issues.
2. Wind Power Systems
Wind turbines generate electricity by harnessing the kinetic energy of moving air. Similar to solar power, wind turbines can be part of a self-sustaining power system, particularly in regions with consistent wind patterns. A small-scale wind turbine can power individual homes or small communities, while large-scale wind farms contribute significantly to national grids.
When combined with energy storage systems, wind power can become a highly efficient self-sustaining energy source. However, wind energy is also intermittent, meaning that it is not always available when needed. Like solar energy systems, wind power systems often rely on hybrid solutions or backup generators to ensure a continuous power supply.
Another benefit of wind power is its minimal environmental footprint. Once installed, wind turbines generate electricity without producing greenhouse gases or other pollutants, making them an environmentally friendly option for self-sustaining power.
3. Hydropower Systems
Hydropower is another form of renewable energy that can be used for self-sustaining power generation. By using the kinetic energy of flowing water, hydropower systems can generate electricity continuously as long as there is a water source. Small-scale hydropower systems, also known as micro-hydropower, can be installed on streams or rivers to provide electricity to remote or off-grid communities.
One of the advantages of hydropower is its reliability. Unlike solar and wind, which are intermittent, water flows tend to be more consistent, providing a more stable source of energy. Additionally, hydropower systems can serve as a storage solution. By using reservoirs, hydropower systems can store water during periods of low demand and release it during peak demand, making them a versatile component of self-sustaining power grids.
4. Biomass Energy Systems
Biomass energy is another key player in the self-sustaining power space. Biomass refers to organic materials such as wood, agricultural residues, or dedicated energy crops that can be used as fuel to produce electricity, heat, or biofuels. Unlike solar and wind, biomass systems generate energy by burning or processing organic material, which makes them more similar to traditional power plants, but with a renewable twist.
A residential biomass generator, for instance, can convert organic waste or wood into energy, allowing a home to be powered independently of the grid. The process of biomass gasification, where organic materials are converted into syngas, is a popular method for generating both heat and electricity. Biomass systems can also be designed in a closed-loop manner where waste products from the process are recycled back into the system, further enhancing sustainability.
In areas where agricultural waste is abundant, biomass energy systems offer an excellent self-sustaining solution. They not only reduce waste but also provide a constant energy supply that is less weather-dependent than solar or wind power.
5. Geothermal Power Systems
Geothermal energy taps into the natural heat stored beneath the Earth’s surface to generate electricity or provide direct heating. In certain regions where geothermal resources are plentiful, geothermal power systems can offer a highly sustainable and reliable source of energy.
Geothermal systems are inherently self-sustaining, as the Earth continuously produces heat from radioactive decay and the residual heat from the planet’s formation. This heat can be harnessed by drilling wells and using the steam or hot water to drive turbines for electricity generation.
One of the major advantages of geothermal power is its reliability. Unlike solar or wind, geothermal systems provide a constant and stable energy supply, making them a valuable component of any self-sustaining energy strategy. However, geothermal energy is location-specific, limiting its widespread application.
Challenges and Limitations of Self-Sustaining Power Sources
While the idea of a self-sustaining power source is appealing, there are several challenges and limitations that must be considered:
- Energy Storage: For renewable systems like solar and wind to be truly self-sustaining, energy storage is critical. Battery technology is improving, but it remains expensive and can degrade over time, reducing the overall efficiency of the system.
- Intermittency: Many renewable energy sources, such as solar and wind, are intermittent, meaning they do not provide power 24/7. This can be mitigated by hybrid systems or backup generators, but it remains a challenge for achieving complete energy independence.
- Initial Costs: The upfront investment required for renewable energy systems, especially when combined with energy storage solutions, can be prohibitive for some individuals and businesses. While the long-term savings are significant, the high initial costs can be a barrier to adoption.
- Geographical Constraints: Not all regions are suitable for all types of renewable energy. For example, solar power is most effective in sunny regions, while wind power requires consistent wind patterns, and geothermal energy is only viable in certain geological locations.
The Future of Self-Sustaining Power Sources
As technology continues to advance, self-sustaining power sources are becoming more efficient and accessible. Improvements in energy storage, such as next-generation batteries and other technologies like hydrogen storage, are helping to mitigate the challenges of intermittency in renewable energy systems. Additionally, innovations in microgrids and smart grid technologies are allowing for better management of distributed energy resources, enabling more households and communities to achieve energy independence.
With the growing emphasis on sustainability and reducing carbon emissions, self-sustaining power sources will play a crucial role in the global energy transition. Off-grid living, powered by a combination of renewable energy technologies, is becoming more feasible and attractive, especially in remote or underserved areas.
Conclusion
Self-sustaining power sources offer a path toward greater energy independence, sustainability, and resilience. Whether through renewable energy systems like solar and wind or through innovative closed-loop biomass and geothermal systems, the potential for achieving autonomy from centralized grids is growing. As technological advancements continue to drive down costs and improve efficiency, the dream of living off-grid or creating self-sustaining energy solutions for homes, businesses, and communities is becoming a reality. The future of energy lies in creating systems that not only sustain themselves but also contribute to a cleaner, more sustainable world.
Residential Biomass Generator
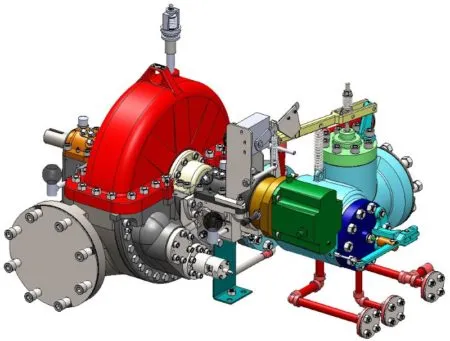
In the pursuit of sustainable and renewable energy sources, biomass generators have emerged as a viable option for residential power generation. These systems allow homeowners to convert organic materials, such as wood, agricultural waste, or dedicated energy crops, into electricity, heat, or biofuels. A residential biomass generator provides an eco-friendly alternative to conventional power sources, reducing dependence on fossil fuels and cutting down on greenhouse gas emissions.
Biomass generators work by using biological materials to create energy, whether through combustion, gasification, or anaerobic digestion. This section will explore how residential biomass generators function, their benefits, different types of systems available, and considerations for those looking to integrate this technology into their homes.
What is Biomass Energy?
Biomass energy is derived from organic materials that can be burned or processed to release stored chemical energy. This energy can then be used to generate heat or electricity. Biomass refers to a variety of biological materials, including wood, plant waste, animal manure, and even certain types of trash. These materials can either be combusted directly to produce heat, or they can undergo processes like gasification or anaerobic digestion to produce biogas, which can then be burned to generate electricity or heat.
Biomass is considered renewable because plants, trees, and other biological materials can be replenished within a relatively short period, unlike fossil fuels which take millions of years to form. Furthermore, when biomass is sourced sustainably, the carbon dioxide emitted during combustion is offset by the carbon dioxide absorbed by plants during their growth, making biomass a carbon-neutral energy source.
How Residential Biomass Generators Work
A residential biomass generator operates by converting organic materials into a usable form of energy, such as heat or electricity. The three main processes used in residential biomass systems are combustion, gasification, and anaerobic digestion:
1. Combustion
The most straightforward method of generating energy from biomass is direct combustion. In this process, organic material (such as wood pellets, logs, or agricultural waste) is burned to produce heat. This heat can be used to warm a home or drive a steam turbine to generate electricity. Combustion systems are typically the simplest type of biomass generator and are widely used in residential heating systems, such as wood stoves or pellet boilers.
For a residential biomass generator that produces electricity, the process is slightly more complex. The heat from combustion is used to generate steam, which drives a turbine connected to a generator, producing electricity. These systems are most effective when combined with cogeneration, where both electricity and heat are produced and used within the home.
2. Gasification
Gasification is a process where biomass is converted into a combustible gas (called syngas) through a reaction that occurs in a low-oxygen environment. The biomass is heated to high temperatures, and instead of burning, it releases gases like carbon monoxide, hydrogen, and methane. These gases can then be burned to generate electricity or heat.
Gasification systems are particularly efficient because they produce a clean-burning fuel with lower emissions compared to direct combustion. Small-scale residential gasifiers are available and can use a variety of feedstocks, such as wood chips, agricultural residues, or even household waste. The syngas produced can power a gas engine or generator, providing a steady source of electricity.
3. Anaerobic Digestion
Anaerobic digestion is a biological process that breaks down organic matter in the absence of oxygen, producing biogas (a mixture of methane and carbon dioxide) and digestate (a nutrient-rich byproduct). Residential anaerobic digesters are typically used to process food waste, animal manure, or other biodegradable materials. The biogas produced can be used in a modified internal combustion engine to generate electricity or heat.
Anaerobic digestion is particularly well-suited for households with access to large quantities of organic waste, such as farms or rural homes. The process is slower than combustion or gasification but is more environmentally friendly since it reduces methane emissions from organic waste that would otherwise decompose in landfills.
Advantages of Residential Biomass Generators
Residential biomass generators offer several advantages, especially for homeowners seeking energy independence and a reduction in their environmental footprint. Below are some key benefits of biomass energy systems:
1. Renewable and Carbon-Neutral
Biomass energy is considered renewable because organic materials, such as plants and wood, can be regrown and harvested sustainably. When managed properly, biomass systems are carbon-neutral, meaning the amount of carbon dioxide released during combustion is offset by the carbon dioxide absorbed by plants during their growth. This makes biomass an attractive alternative to fossil fuels for those looking to reduce their greenhouse gas emissions.
2. Energy Independence
One of the key benefits of a residential biomass generator is the potential for energy independence. Homeowners who install biomass systems can reduce or eliminate their reliance on grid electricity, especially if they live in areas with abundant organic materials. Biomass generators also provide a reliable source of power in off-grid situations, making them particularly useful in rural or remote locations where grid access may be limited or unreliable.
3. Utilization of Waste
Biomass energy systems allow homeowners to utilize organic waste that might otherwise go to landfills or be incinerated. Agricultural waste, food scraps, and wood residues can all be processed in biomass generators to produce heat or electricity. This not only reduces waste but also turns it into a valuable energy source.
4. Reduced Energy Costs
While the initial investment in a residential biomass generator can be significant, it can lead to long-term savings by reducing energy bills. For households with access to a steady supply of biomass, the ongoing fuel costs are often lower than traditional fuels like natural gas, oil, or electricity from the grid. In some cases, excess energy produced by a biomass generator can be sold back to the grid, providing an additional revenue stream.
5. Versatility of Feedstock
One of the unique advantages of biomass energy is the wide range of feedstocks that can be used. From wood chips and pellets to agricultural residues and even animal manure, biomass systems are incredibly versatile. This allows homeowners to select the most cost-effective and readily available materials for their particular location and situation.
Types of Residential Biomass Generators
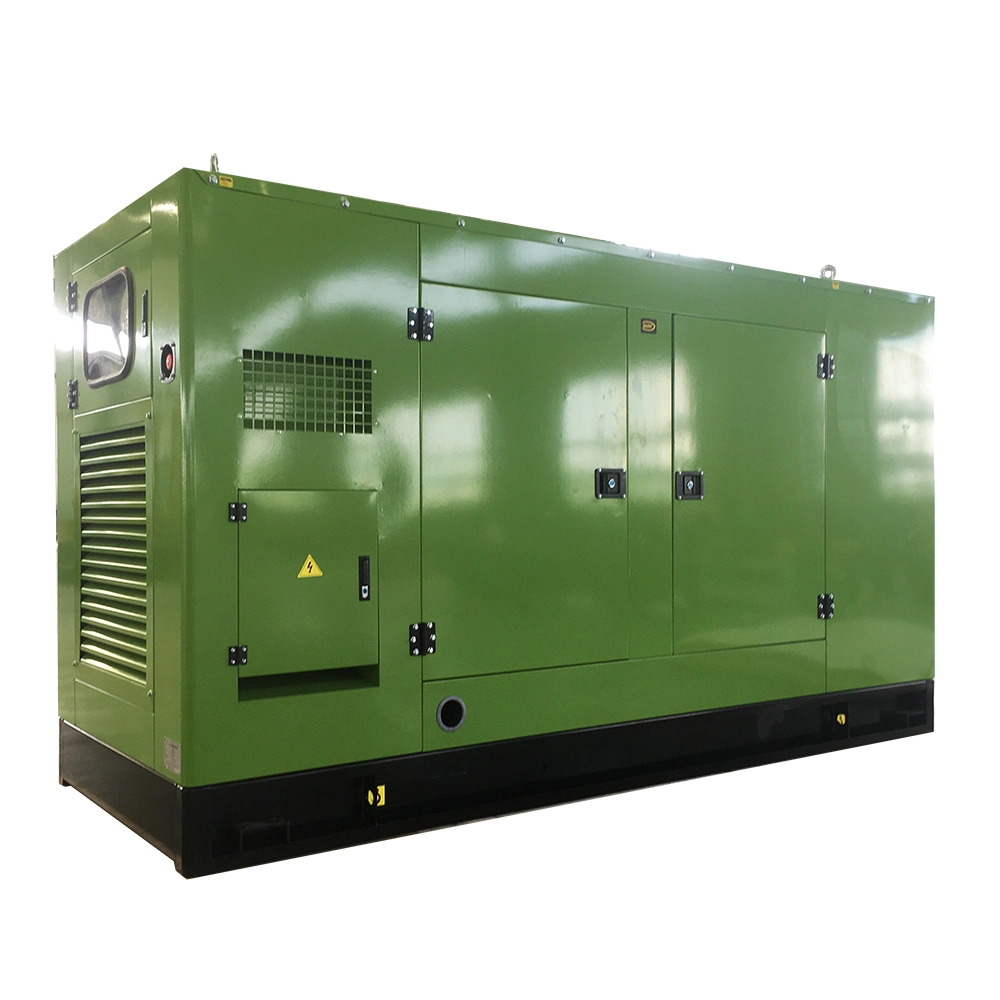
There are several types of residential biomass generators available on the market, each with its own set of advantages and disadvantages. The choice of system depends on factors such as the available feedstock, energy needs, and the level of complexity the homeowner is comfortable with.
1. Wood-Fired Boilers and Stoves
Wood-fired boilers and stoves are some of the most common types of residential biomass systems. They burn wood logs, pellets, or chips to produce heat, which can be used for space heating or to generate hot water. These systems are relatively simple and have been used for centuries. Modern wood-fired systems are much more efficient than traditional wood stoves, and many feature automated feeding systems and emissions controls.
Pellet stoves and boilers, in particular, have gained popularity in recent years due to their convenience. Pellets are made from compressed wood waste and are easy to store and transport. Pellet systems typically include an automated feed mechanism that allows the system to run continuously with minimal user intervention.
2. Biogas Digesters
Residential biogas digesters use anaerobic digestion to produce methane from organic waste such as food scraps, manure, or plant material. The biogas can be used to power a generator, providing electricity and heat. Biogas digesters are popular in rural areas, particularly on farms, where there is a consistent supply of organic waste. These systems are environmentally friendly, as they not only reduce waste but also generate renewable energy.
In addition to electricity and heat, biogas digesters produce digestate, which can be used as a nutrient-rich fertilizer, providing an additional benefit for households involved in agriculture or gardening.
3. Gasification Units
Gasification units are less common in residential settings but are growing in popularity due to their efficiency and low emissions. These systems convert solid biomass into syngas, which can then be used to generate electricity or heat. Residential gasifiers are typically designed to run on wood chips, pellets, or agricultural waste.
While gasification systems are more complex than combustion-based systems, they offer higher efficiency and cleaner combustion. Some models are designed for combined heat and power (CHP), meaning they can produce both electricity and heat for the home.
Challenges and Considerations
While residential biomass generators offer numerous benefits, there are also challenges and considerations that homeowners should be aware of before installing a system.
1. Initial Investment
The upfront cost of installing a biomass generator can be significant, especially when compared to conventional heating or electricity systems. This includes the cost of the generator itself, as well as installation and any necessary modifications to the home’s electrical or heating systems. However, the long-term savings on energy bills and the potential for energy independence can make biomass systems a worthwhile investment.
2. Feedstock Availability
Biomass generators require a steady supply of organic material to operate efficiently. Homeowners need to ensure they have access to enough feedstock, such as wood, agricultural residues, or organic waste, to keep the system running. In some cases, this may require regular deliveries of biomass fuel, which can be a logistical challenge in certain areas.
3. Maintenance
Biomass systems, particularly those that involve combustion or gasification, require regular maintenance to ensure optimal performance. This includes cleaning out ash, maintaining fuel feed systems, and inspecting the generator for wear and tear. Homeowners should be prepared for the ongoing maintenance requirements associated with biomass generators.
4. Emissions
While biomass is considered a renewable and carbon-neutral energy source, it can still produce emissions, particularly if the system is not properly maintained. Modern biomass systems are designed to minimize emissions, but homeowners should ensure they comply with local regulations regarding air quality and emissions standards.
Conclusion
Residential biomass generators provide an attractive option for homeowners seeking to reduce their reliance on the grid, lower their energy costs, and embrace more sustainable energy practices. By converting organic waste into heat or electricity, biomass systems offer a renewable, carbon-neutral alternative to traditional fossil fuels. While there are challenges to consider, such as the initial investment and ongoing maintenance, the long-term benefits of biomass energy make it a compelling choice for those looking to embrace renewable energy at home.
5hp Steam Engine
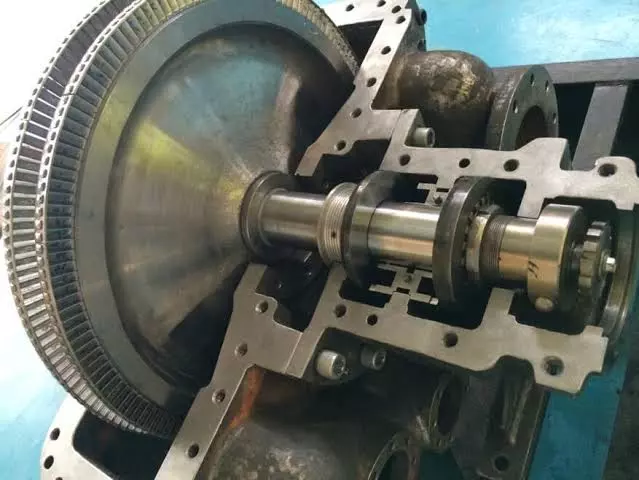
Steam engines have long played a critical role in powering the industrial revolution, and their basic principles still find relevance today, particularly in small-scale power generation applications. A 5-horsepower (hp) steam engine is a versatile piece of machinery that serves various roles, from small industrial tasks to educational purposes, as well as applications in off-grid power generation, hobbyist projects, and even historical restorations. While the size and power output of a 5hp steam engine may seem modest compared to modern combustion engines, it’s an efficient solution for specific tasks where renewable or low-cost energy sources like biomass or coal can be harnessed to generate mechanical or electrical power.
In this section, we will explore the history, design, working principles, and modern applications of a 5hp steam engine. We’ll also cover its potential as a sustainable energy solution and its relevance in the context of renewable energy and off-grid living.
Understanding Horsepower (hp)
Before diving into the specifics of a 5hp steam engine, it’s essential to understand what “horsepower” means in this context. Horsepower is a unit of power that was first developed by James Watt in the 18th century to quantify the power output of steam engines relative to the work done by horses. One horsepower is defined as the ability to perform 550 foot-pounds of work per second, or roughly the equivalent of lifting 550 pounds one foot off the ground in one second.
In the context of a steam engine, 5hp refers to the engine’s ability to generate five times that amount of work. Though it may seem like a modest amount of power, for certain applications—especially in smaller-scale operations—this is more than sufficient. A 5hp steam engine can drive a variety of mechanical systems, from pumps and mills to generators and small manufacturing machines.
History and Development of the 5hp Steam Engine
Steam engines have been integral to industrial development since the 1700s, when early models were developed to pump water out of mines. Over the centuries, steam engine technology evolved significantly, leading to the development of more efficient and compact designs. By the 19th century, smaller steam engines were being developed to power everything from industrial machinery to agricultural equipment and even small vehicles such as steam-powered cars and boats.
The 5hp steam engine emerged as a standard model for small-scale applications. In rural areas, these engines were used to drive machinery like sawmills and water pumps, enabling communities without access to electricity or gasoline to mechanize basic tasks. The advent of internal combustion engines largely supplanted steam engines in the early 20th century, but small steam engines like the 5hp model have persisted in niche applications, particularly where renewable fuels such as wood, coal, or biomass are readily available.
Working Principles of a 5hp Steam Engine
The fundamental principle behind any steam engine, including a 5hp model, is the conversion of heat energy into mechanical work. A steam engine operates by using heat to turn water into steam. The high-pressure steam expands and pushes against a piston or turbine, causing it to move. This movement is then converted into rotary or linear motion, which can be used to drive mechanical devices or generate electricity.
Key Components of a 5hp Steam Engine:
- Boiler: The boiler is where water is heated to produce steam. It’s a critical component of the steam engine, and its size and efficiency directly impact the engine’s performance. In a 5hp steam engine, the boiler is typically compact but must be robust enough to handle high pressure and temperature.
- Piston or Turbine: The high-pressure steam produced in the boiler is directed toward a piston or turbine. In piston engines, the steam enters a cylinder and pushes the piston back and forth. In steam turbines, the steam flows over blades, causing the turbine to spin.
- Flywheel: The flywheel is attached to the crankshaft and helps maintain the momentum of the engine. It stores rotational energy, ensuring smoother operation even as the power from the piston or turbine fluctuates.
- Governor: This component helps regulate the engine’s speed by controlling the steam flow into the cylinder. A well-adjusted governor ensures that the engine operates at a consistent speed, even under varying loads.
- Condenser: After the steam has done its work by moving the piston or turbine, it needs to be condensed back into water to be reused. The condenser cools the steam back into water, which can then be returned to the boiler for reheating.
Fuel Sources for a 5hp Steam Engine
Steam engines are notable for their versatility in terms of fuel sources. They can run on various types of fuel, including:
- Wood: One of the most traditional fuel sources, wood is still used in some small-scale steam engines, particularly in off-grid or rural settings.
- Coal: Coal was historically the dominant fuel for steam engines due to its high energy density and abundance during the industrial era. Though less common today, it remains an option for certain applications.
- Biomass: Biomass, such as wood chips, agricultural residues, or even waste products, can be used to fire the boiler in a 5hp steam engine, making it a more environmentally friendly option.
- Gas or Oil: Some modern steam engines have been adapted to burn natural gas or oil, which offer cleaner combustion than coal or wood.
Efficiency of a 5hp Steam Engine
Steam engines are not the most efficient means of converting energy into mechanical work, with most small-scale engines having an efficiency of around 10-15%. However, for many applications, particularly those where renewable or low-cost fuels are readily available, this is sufficient. Additionally, combining a 5hp steam engine with cogeneration (where both heat and electricity are produced) can improve overall efficiency by making use of the waste heat generated during operation.
Applications of a 5hp Steam Engine
1. Off-Grid Power Generation
A 5hp steam engine can be an excellent solution for generating electricity in off-grid situations. By attaching the engine to a small generator, homeowners or remote facilities can produce enough electricity to power essential systems. When combined with a biomass boiler, this setup can create a fully self-sustaining power source that doesn’t rely on external fuel supplies like gasoline or diesel.
2. Agricultural Uses
In agricultural settings, a 5hp steam engine can be used to drive small machines such as water pumps, grain mills, or sawmills. This is particularly useful in rural areas where modern electricity infrastructure may be lacking or unreliable. The steam engine can operate using locally sourced fuels like wood or agricultural waste, providing a cost-effective solution for small-scale mechanization.
3. Historical Reenactments and Educational Projects
Enthusiasts and hobbyists often use 5hp steam engines for historical reenactments, museum displays, and educational projects. Steam engines are a perfect way to demonstrate the principles of thermodynamics, mechanical engineering, and industrial history. Their relatively simple design makes them accessible for restoration and display.
4. Hobbyist and DIY Projects
Many hobbyists and DIY enthusiasts are drawn to steam engines for their simplicity and the satisfaction of creating a fully functional power source from basic components. A 5hp steam engine can be used to power small workshops, drive tools, or even provide steam propulsion for model boats or vehicles. For these hobbyists, the appeal lies not just in the functionality but in the challenge of mastering a traditional technology.
Sustainability and Environmental Considerations
While steam engines have a reputation for being inefficient compared to modern internal combustion engines, they offer significant sustainability advantages when used with renewable fuels like wood, biomass, or waste products. The ability to run on locally sourced, renewable materials makes steam engines attractive for off-grid power generation or in rural areas where other forms of energy are scarce.
Moreover, when biomass is used as fuel, a steam engine can be part of a carbon-neutral energy cycle. The carbon dioxide released during combustion is absorbed by the plants used to produce the biomass, creating a closed-loop system. In contrast, fossil fuel-based generators release carbon that has been stored underground for millions of years, contributing to global warming.
Challenges and Limitations
While a 5hp steam engine offers many advantages for small-scale power generation, there are some challenges and limitations to consider:
- Maintenance: Steam engines require regular maintenance to operate efficiently and safely. This includes tasks like cleaning out ash from the boiler, lubricating moving parts, and ensuring that the water supply is clean and adequate.
- Fuel Storage: Depending on the fuel used, storage can be an issue. Wood, coal, or biomass requires space for storage and must be kept dry to ensure efficient combustion.
- Efficiency: As mentioned earlier, steam engines are not particularly efficient compared to modern combustion engines. However, for applications where fuel costs are low or where waste heat can be utilized, this efficiency gap can be mitigated.
- Safety: Steam engines operate under high pressure, which can pose safety risks if not properly managed. Boilers must be carefully monitored to prevent accidents like explosions or leaks. Modern safety standards have greatly reduced these risks, but they remain a consideration.
Conclusion
The 5hp steam engine may seem like a relic of the past, but it continues to offer a unique blend of versatility, sustainability, and functionality for certain applications. Whether used for off-grid power generation, small-scale industrial tasks, or as a hobbyist project, the 5hp steam engine provides an accessible and practical way to harness renewable fuels like wood or biomass. Though less efficient than modern alternatives, its simplicity, ease of use, and ability to run on renewable resources make it a viable option for those seeking to minimize their reliance on fossil fuels while maintaining mechanical or electrical power in remote or rural locations.
Exhaust Back Pressure
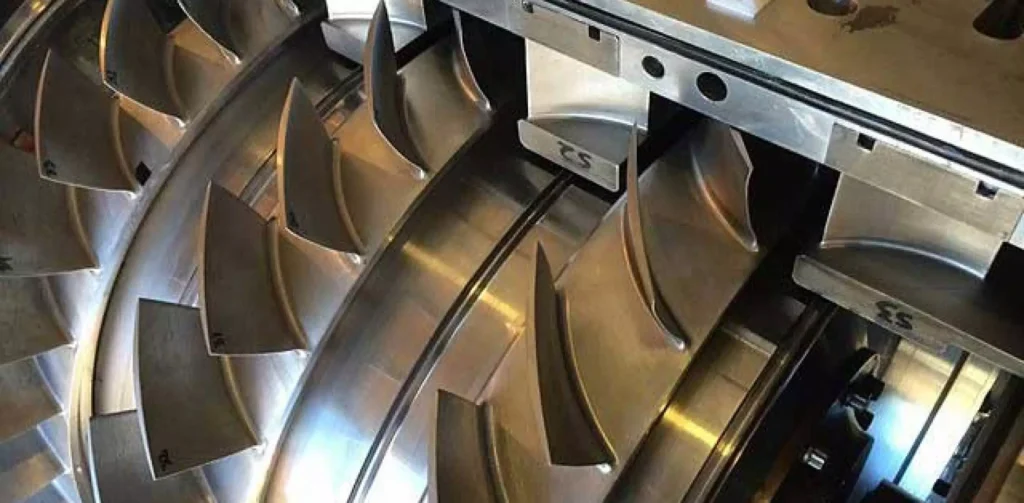
In the world of internal combustion engines and steam systems, exhaust back pressure is a critical factor that directly affects engine efficiency and overall performance. While it may not be as widely discussed as horsepower or torque, managing exhaust back pressure is essential for achieving optimal power output and fuel efficiency, as well as for preventing long-term engine damage. This principle is important across various engine types, from automobiles to steam engines, as well as for turbines in power generation.
This section delves into the concept of exhaust back pressure, its causes, how it impacts performance, and ways to minimize or manage it in both internal combustion and steam-based systems. By understanding exhaust back pressure, engineers and enthusiasts can optimize their systems for better efficiency, lower emissions, and enhanced performance.
What is Exhaust Back Pressure?
Exhaust back pressure refers to the resistance that exhaust gases encounter when they leave an engine. After combustion occurs within an engine (whether it’s a steam engine or an internal combustion engine), the exhaust gases must be expelled from the combustion chamber to allow the next cycle to begin. Ideally, this process should be smooth and free of resistance to ensure that the engine runs efficiently. However, when there is excessive resistance in the exhaust system, it can create back pressure, which restricts the flow of exhaust gases and hampers engine performance.
In internal combustion engines, this exhaust system resistance typically comes from the exhaust manifold, catalytic converters, mufflers, or other components that are designed to filter or reduce noise from exhaust gases. In steam engines or turbines, back pressure can arise from inefficiencies in the system where steam is not fully evacuated, leading to a reduction in power output.
Causes of Exhaust Back Pressure
There are various factors that contribute to exhaust back pressure, each of which can affect engine performance differently. Some of the most common causes include:
1. Obstructions in the Exhaust System
Exhaust systems are designed to direct gases away from the engine as efficiently as possible. However, obstructions like soot build-up, blockages, or damage to the exhaust pipes can significantly increase back pressure. In internal combustion engines, over time, carbon deposits from incomplete combustion can accumulate in exhaust pipes or catalytic converters, leading to higher resistance. Similarly, steam engines may experience blockages in their exhaust system if steam is not properly condensed or expelled.
2. Catalytic Converters
In modern vehicles, catalytic converters are used to reduce harmful emissions by chemically transforming pollutants in the exhaust gases. While necessary for environmental compliance, catalytic converters also introduce some level of resistance to the exhaust flow. A clogged or malfunctioning catalytic converter can drastically increase back pressure, reducing engine performance and causing potential overheating issues.
3. Mufflers
Mufflers are used to reduce the noise produced by exhaust gases as they exit an internal combustion engine. Like catalytic converters, mufflers can create back pressure by restricting the exhaust flow. Performance-oriented vehicles often use specially designed mufflers that balance noise reduction with minimal back pressure to optimize engine output.
4. Steam Condensers
In steam engines and turbines, the condenser is used to cool down and condense the steam back into water after it has passed through the engine or turbine. If the condenser is not functioning efficiently or if it is undersized, it can lead to excess steam remaining in the system, which creates back pressure. This reduces the efficiency of the engine and can lower power output.
5. Piping Design
The design and diameter of exhaust pipes in both internal combustion engines and steam systems can have a significant impact on back pressure. Small diameter pipes, sharp bends, and long exhaust systems can increase resistance and lead to higher back pressure. Proper exhaust pipe design is crucial for maintaining smooth flow and minimizing pressure build-up.
Effects of Exhaust Back Pressure on Engine Performance
Excessive exhaust back pressure can have several negative effects on engine performance, ranging from reduced power output to increased fuel consumption. Understanding these effects is crucial for diagnosing performance issues and improving engine efficiency.
1. Reduced Power Output
One of the most immediate effects of high exhaust back pressure is a reduction in engine power. In an internal combustion engine, when exhaust gases cannot escape efficiently, the engine must work harder to expel them. This reduces the amount of energy available for propulsion, effectively lowering horsepower and torque. In a steam engine or turbine, back pressure restricts the flow of steam, reducing the energy transferred to mechanical components like pistons or turbine blades, thus lowering overall power output.
2. Increased Fuel Consumption
When an engine experiences high exhaust back pressure, it has to work harder to maintain the same level of performance. This increased workload often leads to higher fuel consumption. In internal combustion engines, the air-fuel mixture may become less efficient due to the restricted exhaust flow, leading to incomplete combustion and wasted fuel. Similarly, in steam systems, excess back pressure may force the boiler to consume more fuel to maintain steam production.
3. Overheating
In both internal combustion and steam engines, excessive back pressure can cause overheating. In internal combustion engines, the buildup of exhaust gases increases the temperature in the combustion chamber, which can lead to engine knock or pre-ignition. Over time, this can damage engine components, such as valves, pistons, and gaskets. In steam engines, poor exhaust flow can cause the system to overheat if steam is not being properly vented, putting stress on the boiler and other components.
4. Increased Emissions
Exhaust back pressure can also lead to higher emissions. In internal combustion engines, restricted exhaust flow can result in incomplete combustion, which increases the amount of unburned hydrocarbons and other pollutants in the exhaust gases. In some cases, this can cause a vehicle to fail emissions tests or result in higher environmental impact. In steam systems, incomplete venting of exhaust steam can lower system efficiency, leading to increased fuel consumption and emissions.
5. Reduced Engine Longevity
Over time, excessive exhaust back pressure can cause long-term damage to an engine. Constant strain on the engine to overcome high back pressure can lead to premature wear on critical components, such as valves, piston rings, and bearings. This not only reduces engine performance but can also lead to costly repairs or the need for a complete engine rebuild.
Minimizing Exhaust Back Pressure
To optimize engine performance and prevent the negative effects of back pressure, it is essential to minimize resistance in the exhaust system. Here are some strategies for reducing exhaust back pressure:
1. Optimizing Exhaust Pipe Design
One of the most effective ways to reduce back pressure is by optimizing the design of the exhaust system. This includes ensuring that the exhaust pipes are of the appropriate diameter to allow for efficient flow of gases, and avoiding sharp bends that can increase resistance. For high-performance vehicles, aftermarket exhaust systems with larger diameter pipes are often used to reduce back pressure and improve performance.
2. High-Flow Catalytic Converters
High-flow catalytic converters are designed to reduce back pressure while still meeting emissions standards. These converters have a more open design that allows for better exhaust flow while still performing the necessary catalytic reactions to reduce pollutants. Installing a high-flow catalytic converter can improve both performance and fuel efficiency in internal combustion engines.
3. Upgrading Mufflers
Performance mufflers are designed to minimize back pressure while still reducing exhaust noise. By using sound-dampening techniques that don’t overly restrict exhaust flow, these mufflers allow for more efficient engine performance. This is particularly important for performance-oriented vehicles that need to maximize power output without exceeding noise regulations.
4. Proper Steam Condenser Sizing
For steam engines and turbines, ensuring that the condenser is appropriately sized for the engine’s output is critical to minimizing back pressure. An undersized condenser will not be able to efficiently cool and condense the steam, leading to higher exhaust back pressure. Regular maintenance of the condenser, including cleaning and inspecting for leaks, is also important for maintaining optimal performance.
5. Regular Maintenance
Routine maintenance is essential for preventing exhaust back pressure from becoming a problem. In internal combustion engines, this includes cleaning or replacing clogged catalytic converters and mufflers, as well as checking exhaust pipes for blockages or damage. In steam systems, regular maintenance of the boiler, pipes, and condenser is crucial for ensuring that exhaust steam is properly vented and that no blockages are impeding flow.
Measuring Exhaust Back Pressure
For those looking to diagnose or fine-tune engine performance, measuring exhaust back pressure can be a useful diagnostic tool. There are a few methods for measuring exhaust back pressure in both internal combustion and steam engines:
1. Pressure Gauges
Pressure gauges can be installed in the exhaust system to measure back pressure directly. This is particularly useful for diagnosing performance issues in internal combustion engines, as it allows for real-time monitoring of exhaust conditions. A pressure gauge can be installed just before the catalytic converter or muffler to measure the amount of resistance the exhaust gases are encountering.
2. Flow Meters
Flow meters are another tool that can be used to measure the flow of exhaust gases. By comparing the flow rate of exhaust gases at different points in the system, you can identify areas where back pressure is higher than it should be. This method is particularly useful in larger industrial steam systems, where exhaust flow plays a critical role in overall efficiency.
3. Exhaust Gas Analyzers
In internal combustion engines, an exhaust gas analyzer can help identify incomplete combustion and increased emissions, which may be signs of excessive back pressure. By measuring the levels of unburned hydrocarbons, carbon monoxide, and other pollutants in the exhaust gases, you can determine whether back pressure is causing performance and efficiency issues.
Conclusion
Exhaust back pressure is an often-overlooked factor that plays a significant role in engine performance, fuel efficiency, and longevity. Whether you’re dealing with an internal combustion engine or a steam-based power system, managing exhaust back pressure is critical to ensuring optimal performance and minimizing the risk of long-term damage. By understanding the causes of back pressure, its effects on performance, and the methods for minimizing it, you can improve the efficiency and reliability of your engine or turbine system. Through proper maintenance, thoughtful design, and careful monitoring, exhaust back pressure can be kept in check, allowing your system to operate at its full potential.
Advantages of Superheated Steam
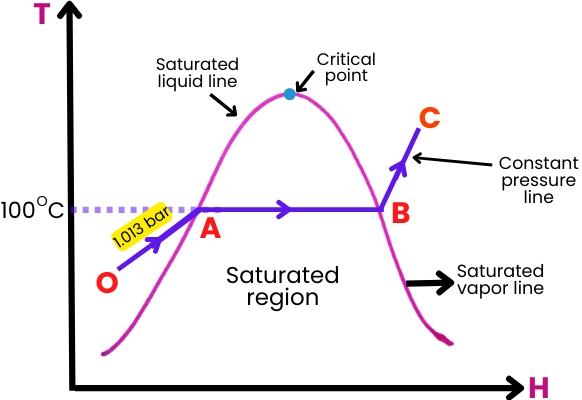
Superheated steam plays a pivotal role in a wide range of industrial, power generation, and engineering applications. It is produced when steam is heated beyond its saturation temperature, which means it no longer contains water droplets and exists entirely in a gaseous state. This transformation gives superheated steam unique properties that make it highly valuable in steam turbines, heat exchangers, and various other processes requiring high-efficiency heat transfer and energy conversion.
In this section, we will explore the advantages of superheated steam, its industrial applications, and the key reasons why it has become a crucial part of modern energy production and engineering.
What is Superheated Steam?
Superheated steam is steam that has been heated to a temperature higher than its boiling point without increasing its pressure. This process eliminates all remaining moisture, meaning the steam exists purely as a gas. For example, water boils at 100°C (212°F) at standard atmospheric pressure, and steam produced at this temperature is known as “saturated steam.” If this steam is further heated to, say, 200°C (392°F), it becomes superheated steam.
The transformation from saturated to superheated steam occurs in specialized equipment called superheaters, typically found in power plants or industrial boilers. This additional heating provides superheated steam with enhanced energy-carrying capacity, as well as other benefits that make it ideal for specific applications.
Advantages of Superheated Steam
1. Higher Thermal Efficiency
One of the primary advantages of superheated steam is its significantly higher thermal efficiency. Because it carries more energy in the form of heat, superheated steam can transfer this energy more effectively to mechanical systems, such as steam turbines. When used in power generation, the high-energy content of superheated steam allows for more efficient conversion of heat energy into mechanical work, which translates to higher electricity production.
In contrast, saturated steam contains moisture that can reduce the efficiency of turbines and other mechanical systems by causing condensation. This moisture can lead to energy losses and even damage turbine blades or other components. Superheated steam, on the other hand, eliminates these issues and ensures a more efficient and consistent transfer of energy.
2. Prevention of Condensation
One of the key challenges of using saturated steam in industrial applications is the potential for condensation as the steam cools or loses pressure. Condensation can lead to a variety of issues, such as decreased efficiency, mechanical damage, and the need for more frequent maintenance. For example, in steam turbines, the presence of moisture can cause water hammering, pitting, and erosion of the turbine blades, which can reduce the turbine’s lifespan and performance.
Superheated steam does not suffer from condensation as easily because it is further away from the saturation point, meaning it remains in a purely gaseous state for longer as it cools. This characteristic makes it ideal for applications where high-temperature steam must travel long distances or where avoiding condensation is critical to maintaining operational efficiency.
3. Higher Work Output from Steam Turbines
In power plants that use steam turbines to generate electricity, the efficiency and performance of the turbines depend on the quality of the steam used. Superheated steam is capable of delivering higher work output compared to saturated steam due to its higher energy content. This allows for greater expansion in the turbine, which leads to a more significant conversion of thermal energy into mechanical work.
Turbines designed to operate with superheated steam can also achieve higher rotational speeds, allowing them to generate more electricity with the same amount of input energy. This makes superheated steam particularly valuable in high-capacity power generation plants, such as nuclear, coal, and combined-cycle plants, where maximizing the efficiency of each turbine cycle is essential to overall performance.
4. Increased Temperature Range for Heat Transfer
In industrial applications where heat transfer is critical—such as in chemical processing, oil refining, and food manufacturing—superheated steam offers a broader temperature range compared to saturated steam. This extended range allows for more precise temperature control, especially in processes that require uniform and high-temperature heating.
For instance, in the petrochemical industry, superheated steam is used in steam cracking, a process where hydrocarbons are broken down into simpler molecules at high temperatures. Because superheated steam can reach much higher temperatures than saturated steam, it enables these reactions to occur more efficiently, reducing the time and energy required for the process.
5. Reduced Corrosion and Scaling
Steam systems often face the problem of corrosion and scaling, particularly when water droplets or moisture are present in the steam. Moisture promotes the formation of scale on metal surfaces, which reduces heat transfer efficiency and leads to higher maintenance costs. Corrosion, caused by the presence of water and oxygen in the steam, can damage pipes, boilers, and other components, leading to costly repairs or replacements.
Superheated steam, being entirely free of moisture, minimizes these risks. The absence of water droplets means that there is less chance for scale formation and corrosion in steam pipes, boilers, and turbines. This contributes to longer equipment life, reduced downtime for maintenance, and overall lower operating costs for industrial plants.
6. Improved Flow Characteristics
Superheated steam has better flow characteristics compared to saturated steam because it is less dense and more gaseous. This allows it to move more freely through pipelines and other components without causing pressure drops or flow restrictions. The improved flow characteristics make superheated steam easier to transport over long distances without losing significant energy or pressure.
In applications where steam needs to be distributed across large areas or through complex piping networks, superheated steam provides better overall performance and ensures that the steam arrives at its destination with minimal energy loss.
7. Enhanced Performance in Industrial Drying Processes
Superheated steam is widely used in drying processes because of its ability to transfer large amounts of heat without introducing additional moisture. This is particularly important in industries such as textiles, paper manufacturing, and food processing, where moisture content needs to be tightly controlled.
In these industries, superheated steam helps achieve faster and more efficient drying compared to traditional hot air or saturated steam. By rapidly evaporating water from materials without condensing on the surface, superheated steam ensures uniform and high-quality drying, reducing the risk of product defects.
8. Higher Power Plant Efficiency
Power plants that use superheated steam, especially those operating at high pressures, are significantly more efficient than those relying on saturated steam. In these plants, superheated steam enables turbines to operate at higher thermal efficiencies by allowing for a larger temperature difference between the steam entering and exiting the turbine.
This greater temperature difference leads to a more efficient conversion of heat into mechanical work, resulting in higher overall efficiency for the power plant. Plants using superheated steam are also able to achieve higher output with lower fuel consumption, making them more cost-effective and environmentally friendly in the long run.
9. Lower Specific Steam Consumption
Specific steam consumption refers to the amount of steam required to produce a unit of work or energy. Because superheated steam carries more energy than saturated steam, less steam is needed to achieve the same amount of work. This means that systems using superheated steam can operate with lower steam consumption, leading to reduced fuel usage and operating costs.
In power generation, this translates to more electricity being produced per unit of steam, enhancing the overall efficiency of the plant and reducing the environmental impact associated with fuel consumption.
10. Better Control in Steam Injection Applications
Superheated steam is often used in steam injection applications, such as enhanced oil recovery (EOR) in the petroleum industry. In these applications, steam is injected into oil reservoirs to reduce the viscosity of heavy oil, allowing it to flow more freely and be extracted more efficiently.
Superheated steam offers better control in these applications because its higher temperature allows for more precise heating of the oil reservoir. This results in improved oil recovery rates and more efficient extraction processes.
Applications of Superheated Steam
Superheated steam is used in a variety of industrial, energy, and manufacturing applications, where its unique properties offer advantages over saturated steam:
1. Power Generation
In power plants, superheated steam is used to drive steam turbines, which convert thermal energy into mechanical work for electricity generation. Superheated steam allows turbines to operate at higher efficiencies, improving the overall performance of the power plant.
2. Industrial Manufacturing
Superheated steam is used in industrial processes that require high-temperature heat transfer, such as chemical reactions, drying, and sterilization. Its ability to remain in a gaseous state at high temperatures makes it ideal for applications where condensation would be detrimental to the process.
3. Petroleum Refining
In the petrochemical industry, superheated steam is used for processes like steam cracking and distillation, where high temperatures are required to break down complex hydrocarbons into simpler molecules.
4. Food Processing
Superheated steam is used in food processing to sterilize equipment, cook products, and perform other tasks where high-temperature steam is needed without introducing moisture to the food.
5. Enhanced Oil Recovery
In enhanced oil recovery (EOR), superheated steam is injected into oil reservoirs to heat the oil and reduce its viscosity, making it easier to extract. Superheated steam’s high temperature and energy content make it an effective tool for improving oil recovery rates.
Conclusion
Superheated steam offers several advantages over saturated steam, particularly in applications that require high efficiency, precise temperature control, and minimal condensation. Its ability to carry more energy, remain in a purely gaseous state, and prevent corrosion makes it a valuable resource in power generation, industrial processes, and various other engineering applications. By harnessing the unique properties of superheated steam, industries can achieve higher productivity, lower operating costs, and improved sustainability.
Steam Turbine Rotor Forgings
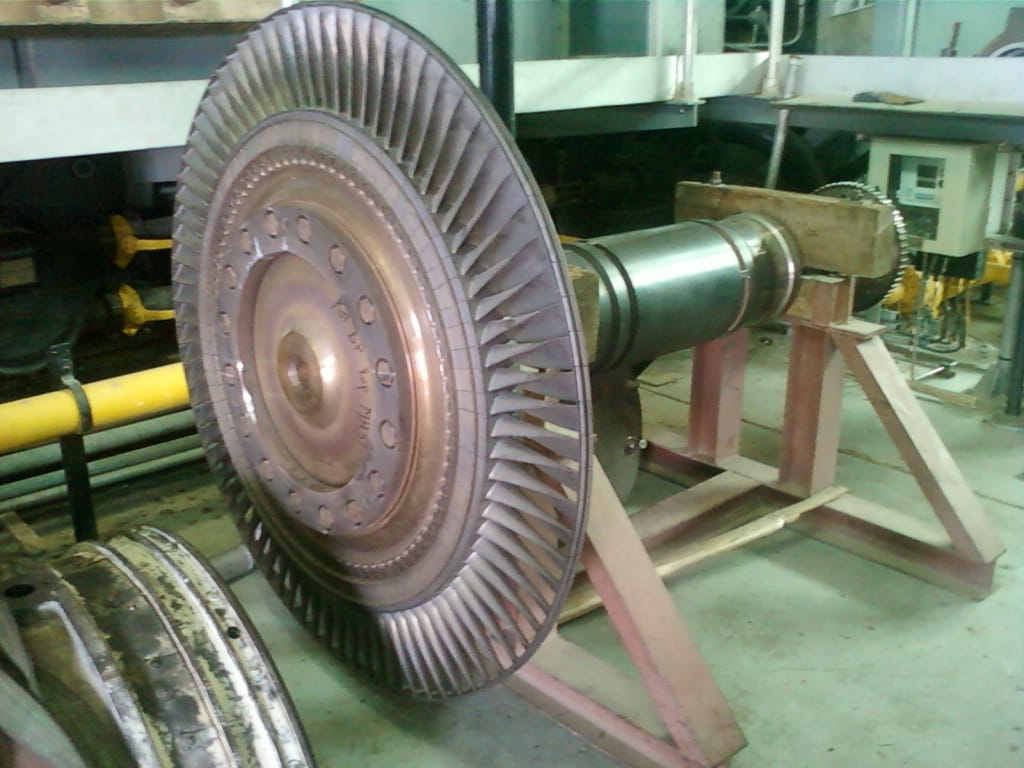
Steam turbines play a critical role in power generation, driving many of the world’s electricity plants by converting the thermal energy from steam into mechanical energy. At the heart of this process lies the steam turbine rotor—a vital component that transforms high-pressure steam into rotational energy. The rotor is the central shaft to which turbine blades are attached, and it must withstand extreme conditions of heat, pressure, and mechanical stress. To ensure that it can meet these demands, rotors are often made using forging techniques, which provide the necessary strength and durability.
In this section, we will explore the importance of rotor forgings in steam turbines, the materials used, the manufacturing processes, and the advantages of using forged components in such a critical application. Understanding steam turbine rotor forgings is essential for those involved in power generation, metallurgy, and heavy manufacturing industries.
What is a Steam Turbine Rotor?
A steam turbine rotor is the rotating component in a steam turbine system that converts the kinetic energy of steam into mechanical energy. It consists of a central shaft, onto which multiple rows of turbine blades are attached. As superheated or high-pressure steam flows over the blades, it causes the rotor to spin at high speeds, which in turn drives an electrical generator or mechanical system.
Rotors must operate under extremely demanding conditions, often spinning at thousands of revolutions per minute (RPM) while enduring high temperatures and pressures. The rotor’s performance directly impacts the turbine’s efficiency, reliability, and overall lifespan. Given these factors, the materials and manufacturing methods used to produce the rotor are of paramount importance.
Importance of Forgings in Steam Turbine Rotors
The process of forging involves shaping metal under high pressure and temperature to produce parts with exceptional mechanical properties. Forged components are denser and stronger than those produced by casting or machining, as the forging process refines the grain structure of the metal, eliminating porosity and reducing the likelihood of defects.
In the case of steam turbine rotors, forging is preferred over other manufacturing methods for several reasons:
1. Enhanced Strength and Durability
Steam turbine rotors must withstand massive centrifugal forces as they spin at high speeds. The forging process enhances the mechanical strength of the material, making it more resistant to deformation and failure. Forged rotors have a uniform grain structure that aligns with the shape of the component, providing better resistance to fatigue, stress, and mechanical wear.
2. Resistance to Thermal Fatigue
Steam turbines operate under high thermal loads due to the continuous exposure to steam at elevated temperatures. Over time, these thermal cycles can cause fatigue in the material, leading to cracks and eventual failure. Forged materials, particularly those made from advanced alloys, have a higher resistance to thermal fatigue compared to cast or machined parts, making them ideal for use in such demanding environments.
3. Improved Mechanical Properties
The forging process imparts superior mechanical properties to the rotor, such as increased tensile strength, toughness, and impact resistance. These properties are essential for withstanding the high rotational speeds and stresses experienced by the rotor during operation.
4. Reduced Risk of Defects
One of the major advantages of forging is the reduction of internal defects, such as voids, inclusions, or porosity, which can weaken the rotor and increase the risk of failure. The high-pressure forging process eliminates these imperfections, ensuring that the rotor is of the highest quality and capable of performing under extreme conditions.
Materials Used in Steam Turbine Rotor Forgings
The choice of materials for steam turbine rotors is critical to ensuring their performance, longevity, and safety. The materials used must have a combination of high strength, toughness, and resistance to heat and corrosion. Some of the most commonly used materials for steam turbine rotor forgings include:
1. Low-Alloy Steels
Low-alloy steels are commonly used in steam turbine rotors due to their high strength, good toughness, and relatively low cost. These steels typically contain small amounts of alloying elements, such as chromium, molybdenum, and nickel, which enhance their mechanical properties and resistance to high temperatures. Low-alloy steels are particularly suitable for applications where the operating temperatures are moderate.
2. High-Alloy Steels
For turbines operating at higher temperatures or pressures, high-alloy steels are often used. These materials contain a higher percentage of alloying elements, such as chromium, vanadium, and tungsten, which provide increased heat resistance, strength, and toughness. High-alloy steels are particularly useful in high-efficiency steam turbines, where performance demands are greater.
3. Stainless Steels
In some cases, stainless steels are used for steam turbine rotors, particularly in environments where corrosion resistance is a critical factor. Stainless steels are highly resistant to oxidation and corrosion, making them ideal for use in turbines exposed to aggressive steam environments, such as those found in certain chemical or petrochemical industries.
4. Nickel-Based Superalloys
In the most demanding steam turbine applications, particularly in high-temperature, high-pressure environments such as those found in nuclear or gas-fired power plants, nickel-based superalloys are used for rotor forgings. These materials offer exceptional resistance to heat, corrosion, and mechanical stress, making them suitable for use in turbines operating at the upper limits of thermal efficiency.
Nickel-based superalloys, such as Inconel or Hastelloy, are capable of maintaining their mechanical properties even at temperatures exceeding 600°C (1112°F), making them ideal for use in advanced steam turbines.
The Forging Process for Steam Turbine Rotors
The forging of steam turbine rotors is a highly specialized process that requires precision, expertise, and advanced equipment. The goal is to create a rotor with uniform properties, free from internal defects, and capable of withstanding the extreme conditions in which it will operate.
The forging process generally involves the following steps:
1. Material Selection and Preparation
The first step in rotor forging is selecting the appropriate material based on the specific requirements of the turbine. The raw material, usually in the form of a large ingot, is carefully inspected to ensure it meets the necessary standards for chemical composition, cleanliness, and quality.
2. Heating
The ingot is heated to a temperature suitable for forging, typically between 1100°C and 1300°C (2012°F to 2372°F), depending on the material. This high temperature makes the material malleable and easier to shape without causing cracks or defects.
3. Forging
Once the material reaches the desired temperature, it is placed under a forging press, which applies immense pressure to shape the material into the form of a rotor. The forging process typically involves multiple stages, including rough forging, finishing forging, and trimming, to achieve the final shape.
During this process, the grain structure of the material is refined, and any internal defects, such as porosity, are eliminated. The forging press can exert pressures of up to several thousand tons, ensuring that the material is fully consolidated and that the rotor has the required mechanical properties.
4. Heat Treatment
After the forging process is complete, the rotor undergoes a series of heat treatments to improve its mechanical properties. This may include processes such as quenching, tempering, or annealing, depending on the material used and the final application. Heat treatment enhances the rotor’s strength, toughness, and resistance to thermal fatigue.
5. Machining and Finishing
Once the rotor has been forged and heat-treated, it undergoes precision machining to achieve the final dimensions and surface finish. This process involves removing any excess material and ensuring that the rotor meets the strict tolerances required for its operation in a steam turbine.
6. Quality Control and Inspection
After machining, the rotor is subjected to rigorous quality control tests to ensure that it meets all necessary specifications. This may include non-destructive testing (NDT) methods, such as ultrasonic testing, magnetic particle testing, or radiographic testing, to check for internal defects, cracks, or other flaws that could compromise the rotor’s performance.
Advantages of Forged Rotors in Steam Turbines
The use of forged rotors in steam turbines offers several key advantages over other manufacturing methods, such as casting or fabrication. These advantages include:
1. Superior Mechanical Strength
Forged rotors have a denser and more uniform grain structure than cast or machined parts, providing superior mechanical strength. This makes them more resistant to the high rotational forces and stresses experienced during turbine operation.
2. Enhanced Durability
The forging process produces components that are more resistant to wear, fatigue, and thermal stress. This means that forged rotors can operate for longer periods without the need for maintenance or replacement, reducing downtime and overall operating costs.
3. Improved Reliability
Forged rotors are less prone to defects such as porosity, inclusions, or cracks, which can compromise the performance of the turbine. The high-quality forging process ensures that the rotor is capable of withstanding the extreme conditions in which it operates, providing better overall reliability.
4. Increased Efficiency
Steam turbines equipped with forged rotors can achieve higher efficiencies due to the rotor’s ability to maintain its mechanical properties under extreme conditions. This translates to improved energy conversion and higher electricity output in power generation applications.
5. Longer Service Life
Forged rotors tend to have a longer service life than their cast or machined counterparts, as they are better able to withstand the rigors of turbine operation. This reduces the need for costly replacements and ensures that the turbine can operate at peak performance for extended periods.
EMS Power Machines
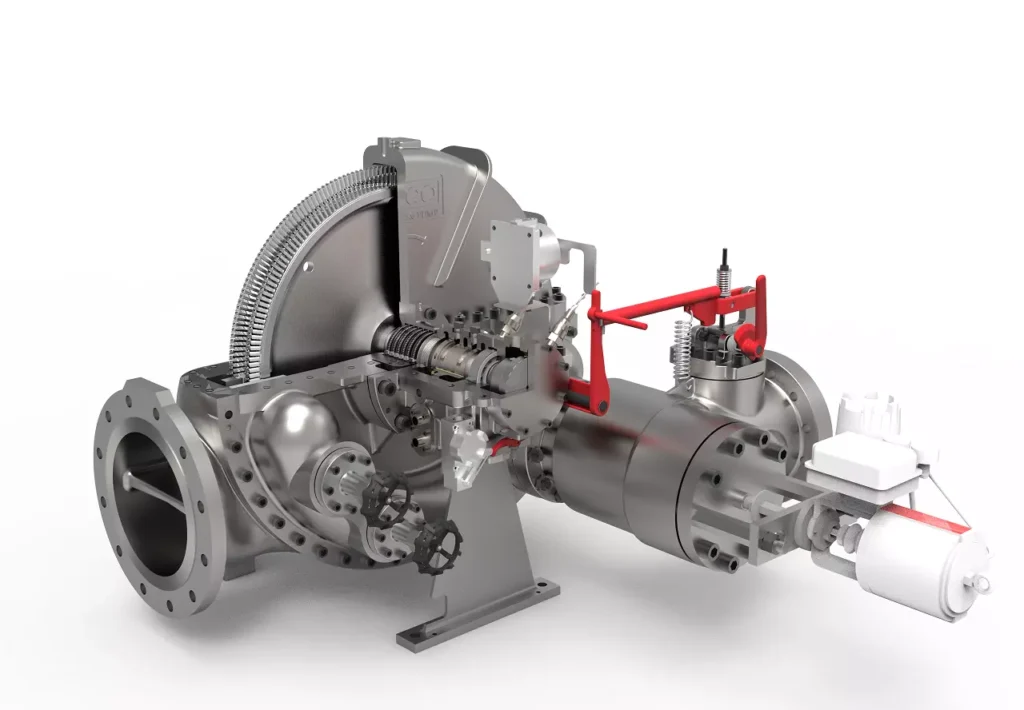
We design, manufacture and assembly Power Machines such as – diesel generators, electric motors, vibration motors, pumps, steam engines and steam turbines
EMS Power Machines is a global power engineering company, one of the five world leaders in the industry in terms of installed equipment. The companies included in the company have been operating in the energy market for more than 60 years.
EMS Power Machines manufactures steam turbines, gas turbines, hydroelectric turbines, generators, and other power equipment for thermal, nuclear, and hydroelectric power plants, as well as for various industries, transport, and marine energy.
EMS Power Machines is a major player in the global power industry, and its equipment is used in power plants all over the world. The company has a strong track record of innovation, and it is constantly developing new and improved technologies.
Here are some examples of Power Machines’ products and services:
- Steam turbines for thermal and nuclear power plants
- Gas turbines for combined cycle power plants and industrial applications
- Hydroelectric turbines for hydroelectric power plants
- Generators for all types of power plants
- Boilers for thermal power plants
- Condensers for thermal power plants
- Reheaters for thermal power plants
- Air preheaters for thermal power plants
- Feedwater pumps for thermal power plants
- Control systems for power plants
- Maintenance and repair services for power plants
EMS Power Machines is committed to providing its customers with high-quality products and services. The company has a strong reputation for reliability and innovation. Power Machines is a leading provider of power equipment and services, and it plays a vital role in the global power industry.
EMS Power Machines, which began in 1961 as a small factory of electric motors, has become a leading global supplier of electronic products for different segments. The search for excellence has resulted in the diversification of the business, adding to the electric motors products which provide from power generation to more efficient means of use.