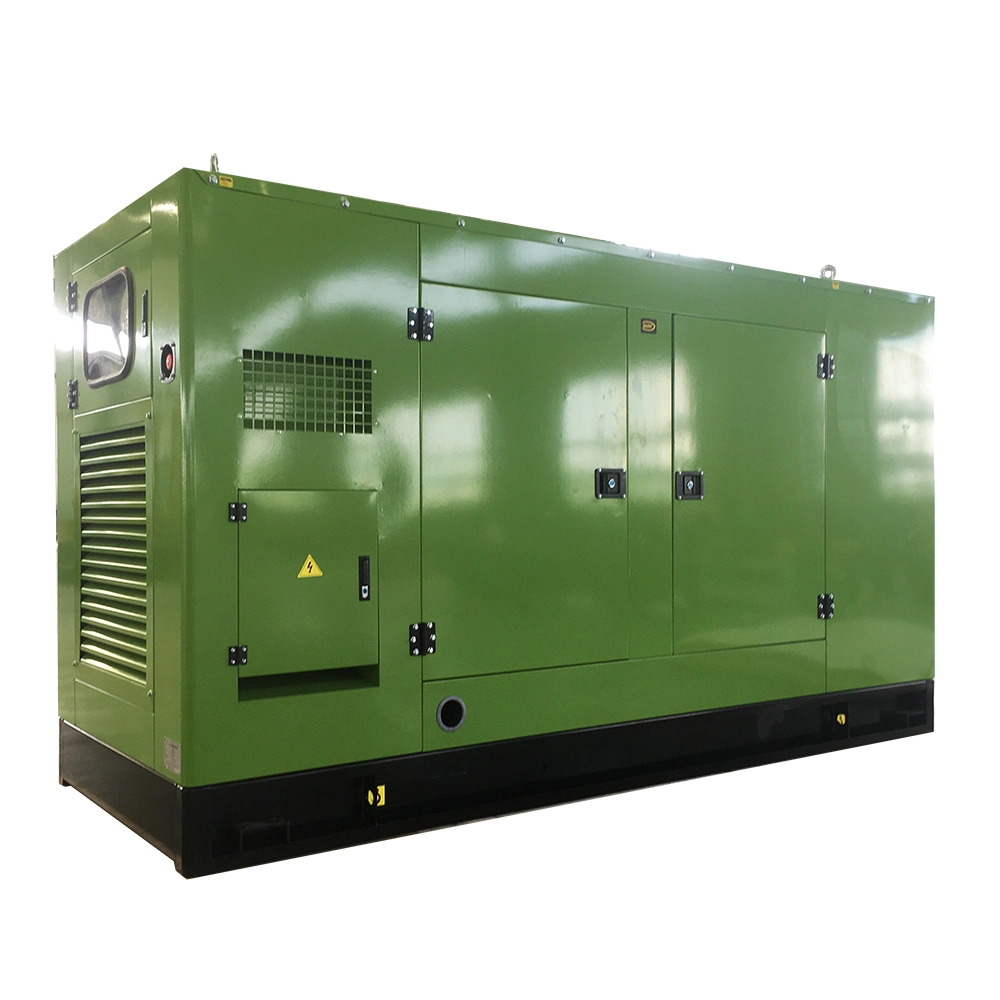
A wood gasifier electric generator is a technology that harnesses the energy released from burning wood or biomass to produce electricity. This innovative system utilizes a gasification process to convert solid wood or other organic materials into a combustible gas known as syngas. Syngas, a mixture of carbon monoxide, hydrogen, and other trace gases, can be utilized as a fuel source for powering an internal combustion engine connected to a generator.
The wood gasification process begins with the feeding of wood or biomass into the gasifier unit. This unit, typically a chamber or reactor, operates in a low-oxygen environment, preventing complete combustion and promoting the production of syngas. The thermochemical reactions involved in gasification break down the complex organic compounds in wood, releasing volatile gases that can be used as fuel.
One of the advantages of wood gasifier electric generators is their ability to use a renewable and widely available resource—wood. Wood is a sustainable and carbon-neutral fuel source, as the carbon dioxide released during combustion is offset by the carbon absorbed by the trees during their growth. This makes wood gasification an environmentally friendly option compared to traditional fossil fuels.
The produced syngas is then directed to an internal combustion engine, commonly a modified gasoline or diesel engine, which is connected to an electric generator. The engine combusts the syngas, converting the chemical energy into mechanical energy that drives the generator to produce electricity. The generated power can be used to supply electricity to homes, businesses, or off-grid locations, providing a decentralized and reliable energy source.
Wood Gasifier Electric Generator
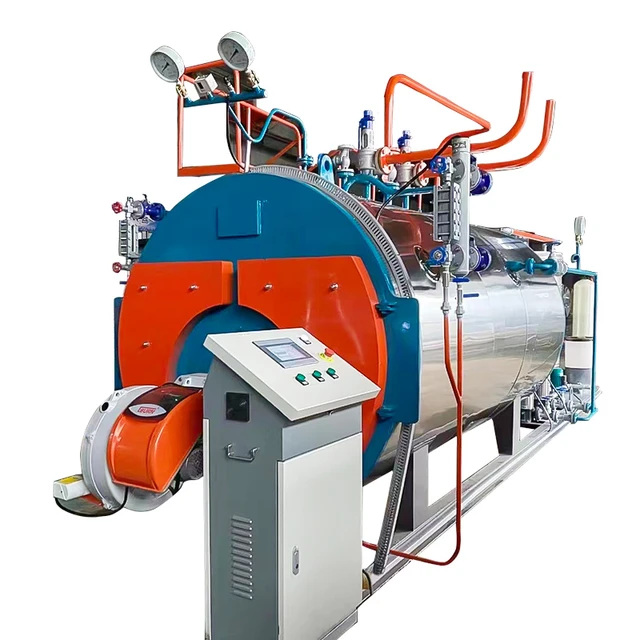
Wood gasifier electric generators find applications in various settings, including rural and remote areas where access to traditional power grids may be limited or impractical. These systems offer a viable solution for off-grid living, emergency backup power, or sustainable energy in regions with abundant biomass resources.
Despite their advantages, wood gasifier electric generators also face challenges. The gasification process can be sensitive to the type and moisture content of the biomass, requiring careful feedstock preparation. Additionally, system efficiency and power output may vary based on the design and quality of the gasifier unit and engine.
In conclusion, wood gasifier electric generators represent a sustainable and versatile solution for decentralized power generation, utilizing the energy potential of biomass to produce electricity. While facing challenges, ongoing advancements in technology and increased awareness of renewable energy options contribute to the continued development and adoption of wood gasification systems as a cleaner and more environmentally friendly energy alternative.
Wood gasification is a process that transforms solid biomass, such as wood, into a gaseous fuel known as syngas. This thermochemical conversion takes place in a gasifier, a device designed to operate in a low-oxygen environment. During gasification, wood is heated, and the lack of oxygen prevents complete combustion, leading to the production of syngas. This versatile energy source has gained attention for its potential to provide a sustainable alternative to traditional fossil fuels.
Wood gasification involves several key steps. First, the biomass, often in the form of wood chips or pellets, is fed into the gasifier. Inside the gasifier, heat initiates a series of chemical reactions, breaking down the complex organic compounds in the wood. This results in the release of volatile gases, including carbon monoxide, hydrogen, and methane, which make up the syngas.
The produced syngas can be harnessed for various applications, with one common use being as a fuel for internal combustion engines connected to generators. This process allows for the generation of electricity from wood, making it a renewable and environmentally friendly energy solution. Wood gasification is particularly appealing in regions where biomass resources are abundant, offering a sustainable alternative to conventional power sources.
Research and development in wood gasification technology continue to improve efficiency, reduce emissions, and expand the range of applicable feedstocks. As a renewable energy option, wood gasification plays a significant role in promoting a greener and more sustainable future, contributing to the global effort to mitigate climate change and reduce dependence on non-renewable energy sources.
Syngas
Syngas, short for synthesis gas, is a versatile fuel produced through the gasification of carbon-containing materials, such as biomass or coal. This gaseous mixture primarily consists of carbon monoxide (CO), hydrogen (H2), and often contains trace amounts of methane (CH4) and other gases. Syngas has gained prominence as a valuable intermediate product that can be used for various applications, including electricity generation, chemical synthesis, and as a precursor for biofuels.
The production of syngas typically involves subjecting carbon-rich feedstocks to high temperatures in a controlled environment with limited oxygen, such as a gasifier. During this thermochemical process, the biomass undergoes pyrolysis, gasification, and reforming reactions, resulting in the release of volatile components that constitute the syngas.
One of the key advantages of syngas is its flexibility as a fuel. It can be employed in internal combustion engines to generate electricity, providing an alternative to traditional fossil fuels. Additionally, syngas serves as a feedstock for the production of liquid biofuels, such as synthetic gasoline or diesel, through further processing known as Fischer-Tropsch synthesis.
Syngas is also utilized in industrial processes, including the synthesis of chemicals like ammonia and methanol. The composition of syngas can be tailored based on specific requirements, making it adaptable to a wide range of applications. Moreover, its production from renewable biomass resources contributes to a more sustainable and environmentally friendly energy landscape.
As advancements in gasification technology and syngas utilization continue, this versatile fuel holds promise for addressing energy challenges, promoting cleaner energy solutions, and reducing greenhouse gas emissions associated with traditional fossil fuels. The ongoing research and development in syngas applications underscore its potential to play a crucial role in the transition to a more sustainable energy future.
Chemical Formula of the Syngas
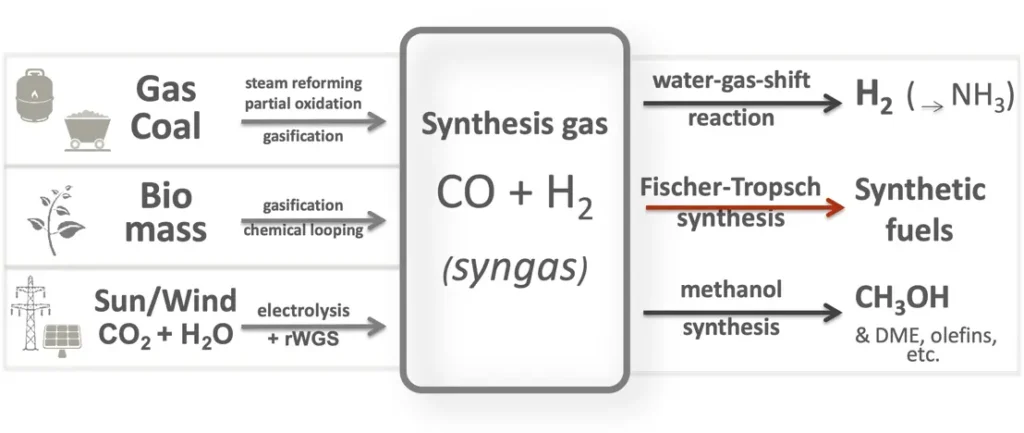
Syngas, or synthesis gas, is a mixture of gases with varying compositions depending on the specific gasification process and feedstock used. The primary components of syngas include carbon monoxide (CO), hydrogen (H2), carbon dioxide (CO2), and methane (CH4), along with trace amounts of other gases. The chemical formulation of syngas can be represented as a combination of these main components:
- Carbon Monoxide (CO):
- Chemical Formula: CO
- Hydrogen (H2):
- Chemical Formula: H2
- Carbon Dioxide (CO2):
- Chemical Formula: CO2
- Methane (CH4):
- Chemical Formula: CH4
In addition to these major components, syngas may contain trace amounts of nitrogen (N2), water vapor (H2O), sulfur compounds (H2S, COS), and other impurities depending on the gasification feedstock and process conditions.
The molar ratios of CO to H2 in syngas can vary, and the specific composition influences the syngas properties and its applications. The most common ratios are expressed as CO-rich or H2-rich syngas, depending on the intended use. For example, CO-rich syngas may be suitable for certain chemical synthesis processes, while H2-rich syngas is often desirable for fuel cell applications.
It’s important to note that the exact composition of syngas can be adjusted by optimizing the gasification process parameters, such as temperature, pressure, and the choice of feedstock. The chemical formulation provided here represents the general components found in syngas, but the actual proportions may vary based on the specific conditions of the gasification process.
Biomass Energy
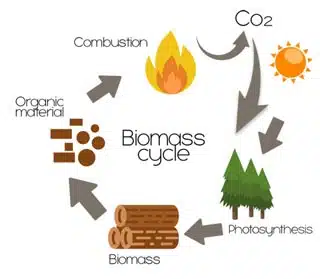
Biomass energy represents a renewable and sustainable form of energy derived from organic materials, such as plants, crop residues, wood, and agricultural byproducts. The utilization of biomass for energy production is rooted in the natural carbon cycle, as the carbon dioxide emitted during its combustion is offset by the carbon absorbed during the growth of the biomass feedstock. One prominent application of biomass energy is in wood gasifier electric generators, contributing to the diversification of the global energy mix.
The primary advantage of biomass energy lies in its potential to serve as a carbon-neutral alternative to traditional fossil fuels. As plants and crops grow, they absorb carbon dioxide from the atmosphere through photosynthesis, storing solar energy in the form of organic compounds. When these biomass materials are burned or converted into biofuels, the stored energy is released, providing a renewable source of heat, electricity, or liquid fuels.
Wood, a common biomass resource, is particularly relevant to the wood gasifier electric generator technology. In gasification systems, wood is transformed into syngas, which can then be utilized to power internal combustion engines connected to generators. This decentralized and sustainable approach to energy production has applications in off-grid settings, rural electrification, and as a backup power source during emergencies.
Biomass energy contributes to reducing dependence on finite fossil fuel resources, mitigating greenhouse gas emissions, and fostering rural development by providing economic opportunities through sustainable agriculture and forestry practices. While challenges exist, such as ensuring responsible biomass sourcing and addressing the potential impacts on land use, ongoing research and technological advancements continue to enhance the efficiency and environmental sustainability of biomass energy systems.
The integration of biomass energy into the broader energy landscape plays a crucial role in transitioning towards a cleaner, more resilient, and sustainable future, addressing the dual challenges of energy security and environmental stewardship.
Renewable Power
Renewable power refers to electricity generated from naturally replenishing resources that are virtually inexhaustible on human timescales. Unlike conventional fossil fuels, which are finite and contribute to environmental degradation, renewable power sources offer a sustainable and eco-friendly alternative. Wood gasifier electric generators exemplify the application of renewable power, harnessing the energy stored in biomass to produce electricity.
The primary characteristic of renewable power sources is their ability to regenerate or replenish themselves naturally. Common examples include solar energy, wind power, hydropower, and biomass energy. In the context of wood gasifier electric generators, the renewable aspect stems from the continuous growth of trees and plants used as biomass feedstocks. As long as these resources are managed sustainably, they can provide a perpetual source of energy.
Wood gasification as a form of renewable power offers several advantages. It reduces reliance on non-renewable fossil fuels, mitigating the environmental impact associated with their extraction, transportation, and combustion. Furthermore, renewable power contributes to energy security by diversifying the energy mix and enhancing resilience against supply disruptions.
The integration of renewable power technologies, such as wood gasifier electric generators, into the energy infrastructure aligns with global efforts to address climate change and reduce greenhouse gas emissions. As these systems become more efficient and cost-effective, they play a crucial role in transitioning towards a low-carbon and sustainable energy future.
Government incentives, technological advancements, and increasing public awareness have accelerated the adoption of renewable power sources worldwide. The ongoing development of wood gasification technology contributes to the broader goal of achieving a more resilient and environmentally responsible energy system, fostering a transition away from fossil fuel dependency.
Decentralized Energy
Decentralized energy refers to the generation of power at or near the point of use, as opposed to centralized energy systems where electricity is produced at large power plants and transmitted over long distances. Wood gasifier electric generators exemplify a decentralized approach, offering a versatile and sustainable solution for producing electricity in diverse locations, including rural areas, remote communities, and off-grid environments.
In a decentralized energy system, power is generated closer to the end-users, reducing the need for extensive transmission and distribution infrastructure. Wood gasifier electric generators are well-suited for decentralized applications, as they allow communities or individual users to generate their own electricity from locally available biomass resources, such as wood chips or agricultural residues.
The decentralization of energy production offers several advantages. It enhances energy resilience by reducing vulnerability to centralized power disruptions, ensuring a more reliable electricity supply in remote or disaster-prone areas. Additionally, decentralized energy systems contribute to energy independence, as communities can rely on local resources to meet their power needs, mitigating dependence on distant power grids.
Wood gasifier electric generators, as part of decentralized energy solutions, empower communities to take control of their energy production, fostering economic development and self-sufficiency. This approach aligns with the principles of sustainability and resilience, especially in regions where traditional grid access may be challenging or economically unfeasible.
As technology advances and the cost of decentralized energy solutions decreases, the adoption of wood gasifier electric generators and similar technologies is likely to increase. This shift towards decentralized energy not only enhances energy security but also contributes to the global transition to more sustainable and environmentally friendly energy systems.
Thermochemical Reactions
Thermochemical reactions refer to chemical processes that involve the exchange or transformation of heat energy. In the context of wood gasifier electric generators, thermochemical reactions play a pivotal role in converting solid biomass, such as wood, into a gaseous fuel known as syngas. These reactions occur within the gasifier unit and are essential for breaking down complex organic compounds in the biomass to release energy in the form of a combustible gas.
The thermochemical reactions in wood gasification consist of several stages, including pyrolysis, gasification, and combustion. Pyrolysis is the initial step where the biomass is heated in the absence of oxygen, leading to the release of volatile components and the formation of char. Gasification follows, involving the conversion of the produced char into a mixture of carbon monoxide, hydrogen, and other gases.
These thermochemical reactions are highly temperature-dependent and require careful control to optimize the gasification process. The goal is to achieve efficient conversion of biomass into syngas while minimizing undesired byproducts and ensuring complete utilization of the available energy.
Understanding and manipulating thermochemical reactions are crucial for designing and improving wood gasification technology. Researchers and engineers work to enhance reaction kinetics, optimize temperature profiles, and explore catalysts to improve the efficiency and reliability of wood gasifier electric generators. Advancements in these thermochemical processes contribute to the overall performance and viability of biomass energy systems.
Thermochemical reactions are not limited to wood gasification; they are fundamental to various energy conversion processes, including combustion engines, gas turbines, and industrial manufacturing. In the specific context of wood gasifier electric generators, a deep understanding of thermochemical reactions is essential for developing efficient and sustainable solutions that harness the energy potential of biomass resources.
Internal Combustion Engine
An internal combustion engine (ICE) is a key component in wood gasifier electric generators, responsible for converting the chemical energy of syngas into mechanical energy that drives an electric generator to produce electricity. Internal combustion engines have been widely used in automotive, industrial, and power generation applications, and their adaptation to run on syngas derived from wood gasification showcases their versatility and utility in decentralized energy systems.
The basic principle of an internal combustion engine involves the controlled combustion of a fuel-air mixture within a combustion chamber. In the case of wood gasifier electric generators, the fuel is syngas, which is a mixture of carbon monoxide, hydrogen, and other gases produced through the gasification of wood or biomass. This combustible gas is introduced into the engine’s combustion chamber, where it is ignited, leading to rapid expansion and the generation of mechanical power.
Wood gasifier electric generators often utilize modified spark ignition or compression ignition engines designed to accommodate the unique characteristics of syngas. The adaptability of internal combustion engines to run on syngas contributes to the feasibility and widespread application of wood gasification technology, especially in off-grid or remote locations where traditional power sources may be impractical.
Efficiency and reliability of internal combustion engines are critical factors in the performance of wood gasifier electric generators. Ongoing research focuses on optimizing engine design, combustion parameters, and fuel delivery systems to enhance overall system efficiency and reduce emissions. The adaptability of internal combustion engines to various fuel sources, including syngas, underscores their role in providing decentralized and sustainable power generation solutions.
As technology continues to advance, internal combustion engines in wood gasifier electric generators contribute to meeting the demand for cleaner, decentralized energy solutions, promoting energy independence and environmental sustainability.
Carbon-Neutral Fuel
Carbon-neutral fuel refers to a type of energy source or fuel that, over its entire life cycle, neither adds nor removes a significant amount of carbon dioxide (CO2) from the atmosphere. Wood gasifier electric generators contribute to carbon neutrality when utilizing wood or biomass as a fuel source. This is because the carbon released during the combustion of biomass is part of the natural carbon cycle, offset by the carbon absorbed during the growth of the biomass feedstock.
In the context of wood gasification, the carbon-neutral aspect stems from the sustainable management of forests and the responsible harvesting of wood for biomass. As trees and plants grow, they absorb carbon dioxide from the atmosphere through photosynthesis, storing the carbon in their biomass. When this biomass is used as fuel in a wood gasifier, the stored carbon is released back into the atmosphere, completing a balanced and sustainable cycle.
Compared to the combustion of fossil fuels, which releases carbon stored in ancient organic matter, using biomass as a carbon-neutral fuel helps mitigate the net increase of CO2 in the atmosphere. The carbon released during biomass combustion is part of the current carbon cycle, creating a closed loop that does not contribute to the long-term accumulation of greenhouse gases.
The concept of carbon-neutral fuel aligns with global efforts to address climate change and reduce the carbon footprint associated with energy production. Wood gasifier electric generators, by utilizing carbon-neutral biomass, provide an eco-friendly and sustainable alternative to traditional fossil fuels, contributing to a more environmentally conscious approach to power generation. As the importance of carbon neutrality grows, the integration of wood gasification technology helps pave the way for cleaner and more sustainable energy systems.
Off-Grid Power
Off-grid power refers to the generation of electricity in locations that are not connected to the traditional centralized power grid. Wood gasifier electric generators play a crucial role in providing off-grid power solutions, offering a reliable and sustainable source of electricity in areas where grid access is limited, impractical, or economically unfeasible.
Off-grid environments include remote or rural areas, isolated communities, and locations without established power infrastructure. In these settings, wood gasifier electric generators offer a decentralized and self-sufficient energy solution. By utilizing locally available biomass resources, such as wood chips or agricultural residues, communities can generate their own electricity without relying on external power grids.
The benefits of off-grid power solutions are manifold. They enhance energy resilience by reducing dependence on centralized power sources, mitigating the impact of power outages and disruptions. Off-grid power systems also contribute to improved energy security, providing a sustainable and reliable source of electricity in areas where traditional grid extensions may be impractical or cost-prohibitive.
Wood gasifier electric generators, as off-grid power solutions, empower communities to meet their energy needs independently. This is particularly significant in remote or underserved areas, where access to reliable electricity can transform daily life, support economic activities, and enhance overall quality of life. Off-grid power solutions contribute to bridging the energy access gap and fostering sustainable development.
As technology advances and the efficiency of wood gasification systems improves, the adoption of off-grid power solutions is likely to increase, offering a practical and environmentally friendly alternative for communities seeking energy independence. The decentralized nature of wood gasifier electric generators aligns with the broader trend towards resilient and sustainable energy systems, supporting a more equitable distribution of power resources.
Sustainable Electricity
Sustainable electricity refers to the generation of power in a manner that meets current energy needs without compromising the ability of future generations to meet their own needs. Wood gasifier electric generators contribute to sustainable electricity by utilizing biomass, a renewable resource, to produce energy in an environmentally responsible and socially equitable manner.
The sustainability of electricity generated through wood gasification is rooted in the carbon-neutral nature of biomass. As trees and plants grow, they absorb carbon dioxide from the atmosphere, incorporating carbon into their biomass. When this biomass is used as fuel in a wood gasifier, the carbon is released as carbon dioxide during combustion. However, since the biomass is part of the current carbon cycle, the net carbon impact is neutral over time.
Furthermore, sustainable electricity from wood gasification helps reduce dependence on finite fossil fuels, mitigating the environmental impact associated with their extraction, transportation, and combustion. The use of biomass as a fuel source promotes responsible land management practices, afforestation, and the development of sustainable supply chains.
Wood gasifier electric generators, as a form of sustainable electricity, offer decentralized power generation that can be tailored to local needs. This is particularly advantageous in rural or off-grid areas, where access to centralized power sources may be limited. The integration of sustainable electricity solutions supports broader environmental goals, including biodiversity conservation and climate change mitigation.
As global awareness of environmental issues continues to grow, the emphasis on sustainable electricity becomes increasingly important. Wood gasifier electric generators exemplify a technology that aligns with the principles of sustainability, providing a viable and eco-friendly option for meeting energy needs while minimizing adverse impacts on the planet and its ecosystems.
Gasifier Technology
Gasifier technology forms the heart of wood gasifier electric generators, playing a pivotal role in converting solid biomass into a usable gaseous fuel, known as syngas. Gasification is a thermochemical process that occurs within the gasifier unit, where biomass undergoes transformation in a controlled environment with limited oxygen, resulting in the production of a combustible gas.
The gasifier unit typically consists of a chamber or reactor where the biomass feedstock, such as wood chips or pellets, is introduced. The key to effective gasification lies in maintaining a low-oxygen or oxygen-starved environment, preventing complete combustion and facilitating the breakdown of complex organic compounds in the biomass. This process leads to the release of volatile gases, predominantly carbon monoxide and hydrogen, which make up the syngas.
Gasifier technology is versatile and can be designed in various configurations, such as updraft, downdraft, or fluidized bed gasifiers. Each design has its advantages and challenges, influencing factors like efficiency, tar content in the syngas, and ease of operation. Advancements in gasifier technology aim to improve efficiency, minimize emissions, and expand the range of applicable biomass feedstocks.
The efficiency of gasifier technology is crucial for the overall performance of wood gasifier electric generators. Research and development focus on optimizing gasifier designs, exploring novel materials, and incorporating advanced control systems to enhance reliability and ease of use. Additionally, gasifier technology advancements contribute to addressing challenges such as feedstock variability, ensuring consistent and stable syngas production.
Gasifier technology is not limited to wood gasification; it finds application in various biomass-to-energy systems and industrial processes. As a core component of wood gasifier electric generators, advancements in gasifier technology contribute to the broader goal of utilizing biomass resources efficiently, sustainably, and economically for decentralized power generation.
Emergency Backup Generator
An emergency backup generator is a critical component of resilience planning, providing a reliable and immediate source of power during disruptions to the primary electricity supply. Wood gasifier electric generators serve as effective emergency backup generators, offering a sustainable and decentralized solution that can operate independently of the main power grid during unforeseen events such as natural disasters, grid failures, or emergencies.
The key advantage of using wood gasifier electric generators as emergency backup systems lies in their ability to utilize locally available biomass resources, such as wood, for fuel. This feature ensures a constant and renewable source of energy even when traditional power sources are compromised. In situations where continuous and reliable power is crucial for essential services, emergency backup generators powered by wood gasification technology provide a resilient and sustainable solution.
During an emergency, the gasification of wood or biomass within the generator unit produces syngas, which is then used to fuel an internal combustion engine connected to an electric generator. This process enables the immediate generation of electricity, offering a quick response to power outages and ensuring that critical operations, such as medical facilities, emergency shelters, or communication centers, can continue functioning.
The use of wood gasifier electric generators as emergency backup systems aligns with the broader trend of enhancing community resilience and disaster preparedness. The decentralized nature of these generators allows for localized power generation, reducing reliance on centralized infrastructure that may be susceptible to widespread failures.
As climate-related events and other emergencies become more frequent, the role of emergency backup generators, especially those utilizing sustainable technologies like wood gasification, becomes increasingly crucial. The adaptability, reliability, and eco-friendly nature of wood gasifier electric generators contribute to their effectiveness as a resilient energy solution in times of crisis.
Carbon Sequestration
Carbon sequestration refers to the process of capturing and storing carbon dioxide (CO2) from the atmosphere to mitigate its impact on climate change. While wood gasifier electric generators release carbon dioxide during the combustion of biomass, the use of sustainably sourced wood as a feedstock is associated with a unique carbon cycle that contributes to overall carbon neutrality and even carbon sequestration.
Trees and plants absorb carbon dioxide from the atmosphere during photosynthesis, converting it into organic compounds and storing it in their biomass. When wood is harvested for use in wood gasifier electric generators, the stored carbon is released back into the atmosphere as CO2. However, the carbon sequestration aspect comes into play when new trees are planted to replace those harvested.
In a sustainable and well-managed forestry system, the newly planted trees absorb CO2 from the atmosphere as they grow, essentially resequestering the carbon released during combustion. This creates a closed carbon cycle, making the overall process of using wood for biomass energy a form of carbon sequestration. The net effect is a reduction in the atmospheric concentration of CO2 compared to burning fossil fuels, which release carbon stored over millions of years.
The concept of carbon sequestration contributes to the appeal of wood gasifier electric generators as an environmentally friendly energy solution. By integrating sustainable forestry practices and ensuring responsible biomass sourcing, the overall impact on the carbon balance can be positive, supporting efforts to combat climate change.
As the importance of carbon sequestration gains prominence in climate change mitigation strategies, the role of wood gasification in contributing to a sustainable and closed carbon cycle becomes increasingly significant. This aligns with broader efforts to transition to low-carbon and environmentally responsible energy systems.
Syngas Utilization
Syngas utilization refers to the various ways in which the produced syngas, a mixture of carbon monoxide, hydrogen, and other gases generated through wood gasification, can be effectively and efficiently employed. In the context of wood gasifier electric generators, syngas is a valuable fuel that can be utilized in different applications to generate power, heat, or even produce biofuels.
One primary application of syngas utilization is in internal combustion engines connected to electric generators. The syngas serves as a clean and renewable fuel source, powering the engine to produce electricity. This method is particularly relevant for decentralized power generation, where wood gasifier electric generators can supply energy to off-grid locations, rural communities, or serve as emergency backup systems.
Syngas can also be employed in combined heat and power (CHP) systems, where the waste heat from electricity generation is captured and utilized for heating purposes. This enhances the overall efficiency of the system by maximizing the utilization of the energy content in the syngas.
Moreover, syngas can be a precursor for the production of biofuels through additional processing. Technologies like Fischer-Tropsch synthesis can convert syngas into liquid biofuels, such as synthetic gasoline or diesel. This diversifies the potential applications of syngas, contributing to a more comprehensive and versatile use of the biomass-derived fuel.
The versatility of syngas utilization is a key strength of wood gasifier electric generators. As technology advances, research continues to explore innovative ways to optimize syngas production and harness its potential in various energy and industrial applications. The adaptability of syngas opens up possibilities for a more sustainable and integrated approach to utilizing biomass resources for power generation and other energy-related processes.
Rural Electrification
Rural electrification involves the process of extending electricity access to rural and remote areas that are often underserved by traditional power grid infrastructure. Wood gasifier electric generators play a significant role in rural electrification initiatives, offering a decentralized and sustainable solution to bring reliable electricity to communities that may be geographically isolated or economically marginalized.
In many rural areas, the extension of conventional power grids can be challenging due to factors such as rugged terrain, low population density, and high infrastructure costs. Wood gasifier electric generators provide an alternative by utilizing locally available biomass resources, such as wood, for power generation. This allows communities to generate electricity on-site, reducing the need for extensive grid expansion.
Wood gasification technology is well-suited for rural electrification because it enables the decentralized production of electricity without relying on large-scale, centralized power plants. The generators can be tailored to the specific energy needs of a community, providing a scalable and flexible solution. Additionally, the carbon-neutral nature of biomass used in wood gasifiers aligns with sustainable development goals and environmental considerations.
By implementing wood gasifier electric generators in rural areas, communities can gain access to essential services such as lighting, heating, and powering agricultural or small-scale industrial activities. This, in turn, can contribute to economic development, improve healthcare facilities, and enhance overall living standards in rural communities.
As technology continues to advance and awareness of the benefits of decentralized energy solutions grows, wood gasifier electric generators hold promise for playing a crucial role in rural electrification efforts worldwide. They exemplify a sustainable and community-centric approach to addressing energy access challenges in underserved regions, supporting global initiatives for inclusive and equitable development.
Wood Gasification Process
Wood gasification is a thermochemical process that converts solid biomass, such as wood, into a gaseous fuel known as synthesis gas or syngas. This process involves heating the biomass in a controlled environment with a limited supply of oxygen, leading to incomplete combustion and the production of a combustible gas mixture.
The wood gasification process typically consists of several stages. Initially, the biomass feedstock, such as wood chips or pellets, is fed into a gasifier chamber. Inside the gasifier, the biomass undergoes pyrolysis, where it is heated to high temperatures in the absence of oxygen, causing it to decompose into solid char, liquid tar, and gaseous products.
As the temperature increases, the char reacts with a controlled amount of oxygen or steam, a process known as gasification. This reaction produces a mixture of carbon monoxide (CO), hydrogen (H2), methane (CH4), carbon dioxide (CO2), and other trace gases. The composition of the syngas produced can be adjusted by controlling the operating parameters of the gasifier, such as temperature, residence time, and feedstock composition.
After gasification, the raw syngas undergoes cleaning and conditioning processes to remove impurities such as tar, particulates, and sulfur compounds. This purified syngas can then be utilized for various applications, including electricity generation, heat production, and as a feedstock for the synthesis of liquid fuels and chemicals.
Wood gasification offers several advantages as a renewable energy technology. It allows for the efficient utilization of woody biomass resources, which are abundant and readily available. Additionally, the syngas produced can be used in combined heat and power (CHP) systems to provide both electricity and heat, increasing overall energy efficiency and reducing greenhouse gas emissions compared to traditional fossil fuel-based power generation methods.
Overall, wood gasification is a versatile and environmentally sustainable process for converting wood and other biomass materials into valuable energy products, contributing to the transition towards a more renewable and low-carbon energy future.
Biomass Gasification:
Biomass gasification is a thermochemical conversion process that transforms biomass feedstock into a combustible gas mixture called syngas or producer gas. This process involves heating biomass materials, such as wood chips, agricultural residues, or energy crops, in a gasifier unit with a controlled amount of oxygen or steam, under high temperatures and in the absence of complete combustion.
The biomass gasification process typically consists of several interconnected stages. Initially, the biomass feedstock is dried to reduce moisture content and then fed into the gasifier chamber. Inside the gasifier, the biomass undergoes pyrolysis, where it is heated to temperatures ranging from 700°C to 1200°C in an oxygen-limited environment. During pyrolysis, the biomass decomposes into solid char, liquid tar, and gaseous products.
As the temperature increases, the char reacts with the remaining oxygen or steam to undergo gasification reactions. This results in the production of a syngas composed primarily of carbon monoxide (CO), hydrogen (H2), methane (CH4), carbon dioxide (CO2), and other trace gases. The composition of the syngas can be controlled by adjusting operating parameters such as temperature, residence time, and the ratio of oxygen to biomass.
After gasification, the raw syngas undergoes cleaning and conditioning processes to remove impurities such as tar, particulates, and sulfur compounds. The purified syngas can then be utilized for various energy applications, including electricity generation, heat production, and as a feedstock for the synthesis of liquid fuels and chemicals.
Biomass gasification offers several advantages as a renewable energy technology. It enables the efficient conversion of diverse biomass feedstocks into a versatile energy carrier, reducing reliance on fossil fuels and mitigating greenhouse gas emissions. Additionally, biomass gasification can provide decentralized energy solutions, particularly in rural areas with abundant biomass resources, promoting energy independence and rural development.
Overall, biomass gasification is a promising technology for sustainable energy production, offering a renewable and carbon-neutral alternative to conventional fossil fuel-based energy sources. Continued research and development efforts aim to improve gasification efficiency, reduce costs, and expand the range of biomass feedstocks suitable for gasification, further enhancing its potential contribution to a sustainable energy future.
Syngas Production:
Syngas, short for synthesis gas, is a versatile fuel gas mixture primarily composed of carbon monoxide (CO) and hydrogen (H2), along with varying amounts of carbon dioxide (CO2), methane (CH4), and other trace gases. Syngas production is a key process in various industrial applications, including power generation, chemical synthesis, and fuel production.
The production of syngas can be achieved through several methods, with gasification being one of the primary techniques for biomass and coal feedstocks. In the context of biomass gasification, the syngas production process involves subjecting biomass feedstock, such as wood chips, agricultural residues, or energy crops, to high temperatures in a gasifier unit with controlled oxygen or steam supply.
During gasification, the biomass undergoes several thermochemical reactions, including pyrolysis and gasification, resulting in the conversion of solid biomass into a gaseous mixture of carbon monoxide, hydrogen, and other gases. The composition of the syngas produced can be adjusted by controlling various parameters such as temperature, residence time, and the ratio of oxygen to biomass.
Syngas production via gasification offers several advantages over conventional combustion processes. It allows for the efficient utilization of diverse biomass feedstocks, including waste materials and agricultural residues, reducing reliance on fossil fuels and contributing to carbon emission reduction goals. Additionally, syngas can be used as a feedstock for the production of synthetic fuels, such as methanol or synthetic natural gas (SNG), or for chemical synthesis processes, enabling the production of a wide range of valuable products.
The versatility of syngas makes it an attractive option for various energy and industrial applications. In power generation, syngas can be used directly in internal combustion engines, gas turbines, or fuel cells to produce electricity and heat. In chemical synthesis, syngas serves as a precursor for the production of fuels, chemicals, and other value-added products, offering opportunities for the integration of renewable resources into the production of high-value chemicals and materials.
Overall, syngas production plays a vital role in the transition towards a more sustainable and low-carbon energy future, offering a versatile and renewable alternative to conventional fossil fuels and enabling the efficient utilization of biomass resources for energy and industrial applications. Continued research and development efforts aim to further improve syngas production technologies, increase efficiency, and expand the range of feedstocks and applications, driving innovation in the field of renewable energy and resource utilization.
Wood-to-Energy:
Wood-to-energy refers to the process of converting wood biomass into usable energy forms, such as electricity, heat, or biofuels. This process involves various technologies and conversion pathways aimed at harnessing the energy stored within wood materials for practical applications.
One of the primary methods of wood-to-energy conversion is combustion, where wood biomass is burned directly to produce heat, which can then be used for space heating, water heating, or steam generation for electricity production in biomass power plants. Combustion can be carried out in various types of boilers, furnaces, or stoves, depending on the scale and application of the energy system.
Another key pathway for wood-to-energy conversion is gasification, which involves thermochemical decomposition of wood biomass into syngas—a mixture of carbon monoxide, hydrogen, and other gases—through a controlled process of heating in a gasifier unit. Syngas produced from wood gasification can be used for electricity generation in gas engines, gas turbines, or fuel cells, or further processed into biofuels and chemicals.
Pyrolysis is another thermochemical conversion process used in wood-to-energy applications, where wood biomass is heated in the absence of oxygen to produce biochar, bio-oil, and syngas. Biochar can be used as a soil amendment or carbon sequestration agent, while bio-oil can be upgraded into biofuels or used as a feedstock for chemical synthesis.
Wood-to-energy technologies offer several advantages as renewable energy sources. Wood biomass is abundant, widely available, and can be sustainably harvested from forests, agricultural residues, or urban wood waste streams. Utilizing wood for energy production can help reduce greenhouse gas emissions by displacing fossil fuel consumption and promoting carbon sequestration through sustainable forestry practices.
Furthermore, wood-to-energy systems can contribute to energy security and independence, particularly in rural areas with access to biomass resources. By diversifying energy sources and promoting decentralized energy production, wood-to-energy technologies can enhance resilience to energy supply disruptions and create economic opportunities for local communities.
Overall, wood-to-energy conversion plays a crucial role in the transition towards a more sustainable and low-carbon energy future, offering a renewable and environmentally friendly alternative to conventional fossil fuels. Continued research and development efforts aim to improve the efficiency, cost-effectiveness, and environmental performance of wood-to-energy technologies, further advancing their contribution to global energy sustainability goals.
Gasification Process:
Gasification is a thermochemical conversion process that transforms carbonaceous materials, such as biomass, coal, or waste, into a gaseous fuel mixture called syngas. This process involves subjecting the feedstock to high temperatures in a controlled environment with a limited supply of oxygen or steam, leading to partial oxidation and the production of a combustible gas.
The gasification process typically consists of several interconnected stages. Initially, the feedstock is dried to reduce moisture content and then fed into the gasifier unit. Inside the gasifier, the feedstock undergoes pyrolysis, where it is heated to high temperatures in the absence of oxygen, causing it to decompose into volatile compounds, char, and ash.
As the temperature increases, the volatile compounds react with a controlled amount of oxygen or steam to undergo gasification reactions. This results in the production of a syngas composed primarily of carbon monoxide (CO) and hydrogen (H2), along with carbon dioxide (CO2), methane (CH4), and other trace gases. The composition of the syngas can be adjusted by controlling operating parameters such as temperature, pressure, residence time, and the ratio of oxygen to feedstock.
After gasification, the raw syngas undergoes cleaning and conditioning processes to remove impurities such as tar, particulates, sulfur compounds, and moisture. This purified syngas can then be utilized for various energy applications, including electricity generation, heat production, and as a feedstock for the synthesis of liquid fuels and chemicals.
Gasification offers several advantages over conventional combustion processes. It enables the efficient utilization of a wide range of feedstocks, including biomass, coal, and waste materials, reducing reliance on fossil fuels and promoting resource efficiency. Gasification also allows for the production of a versatile energy carrier, syngas, which can be used in a variety of energy conversion technologies, such as internal combustion engines, gas turbines, fuel cells, and chemical synthesis processes.
Furthermore, gasification can facilitate the integration of renewable energy sources into existing energy systems by providing dispatchable and flexible power generation options. It also offers opportunities for carbon capture and storage (CCS) through the capture and sequestration of CO2 emissions from gasification processes, contributing to climate change mitigation efforts.
Overall, gasification is a versatile and environmentally sustainable process for converting carbonaceous materials into valuable energy products, contributing to the transition towards a more sustainable and low-carbon energy future. Continued research and development efforts aim to further improve gasification technologies, increase efficiency, and expand the range of feedstocks and applications, driving innovation in the field of renewable energy and resource utilization.
Renewable Energy:
Renewable energy refers to energy derived from naturally replenishing resources that are virtually inexhaustible on a human timescale, such as sunlight, wind, water, and biomass. Unlike fossil fuels, which are finite and contribute to environmental degradation and climate change, renewable energy sources offer sustainable alternatives for meeting energy demand while minimizing environmental impact.
One of the key characteristics of renewable energy sources is their ability to harness natural processes or phenomena to generate usable energy. For example, solar energy technologies, such as photovoltaic (PV) panels and concentrating solar power (CSP) systems, convert sunlight directly into electricity or heat. Wind turbines harness the kinetic energy of wind to generate electricity, while hydropower plants utilize the gravitational potential energy of flowing water to produce power.
Biomass energy, another form of renewable energy, involves the conversion of organic materials, such as wood, agricultural residues, and organic waste, into heat, electricity, or biofuels through processes such as combustion, gasification, or biochemical conversion. Biomass is considered renewable because it can be sustainably harvested or produced, unlike fossil fuels, which require millions of years to form.
Renewable energy technologies offer several advantages over conventional fossil fuel-based energy sources. Firstly, they reduce greenhouse gas emissions and air pollution, helping to mitigate climate change and improve air quality. By displacing fossil fuel consumption, renewable energy contributes to energy security and independence, reducing reliance on imported fuels and volatile global energy markets.
Moreover, renewable energy sources are often decentralized and widely distributed, allowing for greater resilience and flexibility in energy systems. Distributed generation from renewable sources, such as rooftop solar panels or small wind turbines, can enhance energy access and reliability, particularly in remote or off-grid areas where centralized electricity infrastructure may be lacking.
Additionally, renewable energy technologies offer economic benefits by creating jobs, stimulating local economies, and fostering innovation and technological development. As renewable energy deployment continues to grow, costs have declined significantly, making renewables increasingly competitive with conventional energy sources in many parts of the world.
Overall, renewable energy plays a crucial role in the transition towards a more sustainable and low-carbon energy future. Continued investment in research, development, and deployment of renewable energy technologies is essential to further accelerate the global energy transition and achieve ambitious climate and sustainability goals.
Biomass Gasification:
Biomass gasification is a thermochemical conversion process that transforms biomass feedstock into a combustible gas mixture known as syngas or producer gas. This process involves subjecting biomass materials, such as wood chips, agricultural residues, or energy crops, to high temperatures in a gasifier unit with a controlled supply of oxygen or steam, leading to partial oxidation and the production of a gaseous fuel.
The biomass gasification process typically consists of several interconnected stages. Initially, the biomass feedstock is dried to reduce moisture content and then fed into the gasifier chamber. Inside the gasifier, the biomass undergoes pyrolysis, where it is heated to temperatures ranging from 700°C to 1200°C in an oxygen-limited environment. During pyrolysis, the biomass decomposes into volatile compounds, char, and ash.
As the temperature increases, the volatile compounds react with the remaining oxygen or steam to undergo gasification reactions. This results in the production of a syngas composed primarily of carbon monoxide (CO) and hydrogen (H2), along with carbon dioxide (CO2), methane (CH4), and other trace gases. The composition of the syngas can be controlled by adjusting operating parameters such as temperature, residence time, and the ratio of oxygen to biomass.
After gasification, the raw syngas undergoes cleaning and conditioning processes to remove impurities such as tar, particulates, sulfur compounds, and moisture. This purified syngas can then be utilized for various energy applications, including electricity generation, heat production, and as a feedstock for the synthesis of liquid fuels and chemicals.
Biomass gasification offers several advantages as a renewable energy technology. It enables the efficient utilization of diverse biomass feedstocks, including waste materials and agricultural residues, reducing reliance on fossil fuels and mitigating greenhouse gas emissions. Additionally, biomass gasification can provide decentralized energy solutions, particularly in rural areas with abundant biomass resources, promoting energy independence and rural development.
Overall, biomass gasification is a promising technology for sustainable energy production, offering a renewable and carbon-neutral alternative to conventional fossil fuels. Continued research and development efforts aim to improve gasification efficiency, reduce costs, and expand the range of biomass feedstocks suitable for gasification, further enhancing its potential contribution to a sustainable energy future.
Gasifier Technology:
Gasifier technology refers to the design, operation, and optimization of devices called gasifiers, which are used to convert carbonaceous materials into a gaseous fuel mixture known as syngas or producer gas. Gasifiers play a crucial role in various industrial applications, including power generation, chemical synthesis, and fuel production, by enabling the efficient utilization of biomass, coal, or waste feedstocks.
Gasifiers operate on the principle of thermochemical conversion, where feedstock materials are subjected to high temperatures in a controlled environment with a limited supply of oxygen or steam. This process leads to partial oxidation and the production of a combustible gas mixture composed primarily of carbon monoxide (CO) and hydrogen (H2), along with carbon dioxide (CO2), methane (CH4), and other trace gases.
Gasifier technology encompasses a wide range of designs and configurations, each tailored to specific feedstock characteristics, operating conditions, and desired syngas composition. Common types of gasifiers include fixed-bed, fluidized bed, and entrained flow gasifiers, each offering unique advantages and limitations in terms of efficiency, scalability, and feedstock flexibility.
Fixed-bed gasifiers consist of a stationary bed of solid material through which the feedstock moves as it undergoes gasification reactions. Fluidized bed gasifiers suspend the feedstock particles in a bed of inert material, such as sand or ash, using a stream of gas to fluidize the bed and enhance heat and mass transfer. Entrained flow gasifiers entrain the feedstock particles in a high-velocity stream of oxidizing gas, resulting in rapid heating and conversion to syngas.
Gasifier technology requires careful consideration of several design and operational parameters to optimize performance and syngas quality. These parameters include gasifier geometry, temperature, pressure, residence time, feedstock particle size and composition, as well as the ratio of oxygen to feedstock. Control and monitoring systems are essential for maintaining stable operation and maximizing efficiency while minimizing emissions and tar formation.
Advancements in gasifier technology continue to drive innovation in renewable energy and resource utilization, enabling the efficient conversion of diverse feedstocks into valuable energy products. Research efforts focus on improving gasifier performance, enhancing feedstock flexibility, reducing costs, and integrating gasification with other energy conversion technologies, such as combined heat and power (CHP) systems and carbon capture and storage (CCS) technologies.
Overall, gasifier technology plays a vital role in the transition towards a more sustainable and low-carbon energy future, offering versatile and efficient solutions for converting carbonaceous materials into clean and renewable energy sources. Continued research, development, and deployment of gasification technologies are essential to address global energy and environmental challenges and accelerate the adoption of renewable energy worldwide.
Sustainable Energy:
Sustainable energy refers to energy sources and practices that meet present needs without compromising the ability of future generations to meet their own needs. It encompasses renewable energy sources, energy efficiency measures, and environmentally responsible energy management practices aimed at reducing environmental impact, promoting economic development, and ensuring social equity.
One of the key pillars of sustainable energy is the utilization of renewable energy sources, such as solar, wind, hydroelectric, biomass, and geothermal energy. These sources are derived from natural processes or phenomena that are continuously replenished, making them virtually inexhaustible on human timescales. Renewable energy technologies harness these sources to generate electricity, heat, and mechanical power, offering clean, reliable, and environmentally friendly alternatives to fossil fuels.
Energy efficiency is another critical aspect of sustainable energy, focusing on minimizing energy waste and optimizing energy use across various sectors, including buildings, transportation, industry, and agriculture. Energy-efficient technologies, practices, and policies aim to reduce energy consumption, improve energy productivity, and lower greenhouse gas emissions, leading to cost savings, enhanced competitiveness, and environmental benefits.
Furthermore, sustainable energy encompasses principles of environmental stewardship, social equity, and economic viability. It seeks to minimize environmental impact by reducing pollution, conserving natural resources, and protecting ecosystems and biodiversity. Sustainable energy initiatives also aim to promote social equity by ensuring equitable access to clean and affordable energy services, particularly for underserved communities and vulnerable populations.
In addition to environmental and social considerations, sustainable energy initiatives must also be economically viable and financially sustainable. This requires balancing upfront investment costs with long-term benefits, considering factors such as energy savings, avoided environmental damages, and job creation opportunities. Sustainable energy financing mechanisms, such as subsidies, incentives, grants, and innovative financing models, play a crucial role in overcoming financial barriers and incentivizing investment in clean energy solutions.
Overall, sustainable energy represents a holistic approach to energy production, distribution, and consumption that integrates environmental, social, and economic considerations. By transitioning to sustainable energy systems, societies can reduce dependence on fossil fuels, mitigate climate change, improve energy security, create green jobs, and foster inclusive and resilient communities. Continued innovation, investment, and collaboration are essential to accelerate the global transition towards a more sustainable and low-carbon energy future.
Energy Independence:
Energy independence refers to a state in which a country or region is able to meet its energy needs without relying heavily on imported energy sources or external energy suppliers. It represents a strategic goal aimed at enhancing energy security, reducing vulnerability to supply disruptions and price fluctuations, and promoting economic stability and national sovereignty.
Achieving energy independence involves diversifying energy sources and reducing dependence on imported fossil fuels, such as oil, natural gas, and coal, which are subject to geopolitical risks, market volatility, and environmental concerns. Countries pursue various strategies to enhance energy independence, including increasing domestic production of renewable energy, expanding domestic energy infrastructure, promoting energy efficiency, and investing in energy storage technologies.
Renewable energy plays a crucial role in enhancing energy independence by providing domestically available and inexhaustible energy sources that can reduce reliance on imported fossil fuels. Solar, wind, hydroelectric, biomass, and geothermal energy resources can be harnessed to generate electricity, heat, and transportation fuels, offering clean, reliable, and locally sourced alternatives to imported energy sources.
Energy efficiency measures also contribute to energy independence by reducing overall energy consumption and dependence on external energy supplies. Improvements in energy efficiency across various sectors, including buildings, transportation, industry, and agriculture, can lower energy demand, increase energy productivity, and enhance energy security by reducing reliance on imported energy resources.
Furthermore, enhancing domestic energy infrastructure, such as transmission and distribution networks, storage facilities, and smart grid technologies, can improve energy resilience and flexibility, enabling more efficient utilization of renewable energy sources and reducing dependence on imported energy supplies.
Promoting energy independence is not only a matter of national security but also economic competitiveness and environmental sustainability. By diversifying energy sources and reducing dependence on imported fossil fuels, countries can mitigate risks associated with energy supply disruptions, enhance economic stability, create jobs, and stimulate domestic innovation and investment in clean energy technologies.
Overall, energy independence represents a strategic imperative for countries seeking to enhance energy security, promote economic development, and address global challenges such as climate change and geopolitical instability. By embracing renewable energy, energy efficiency, and domestic energy infrastructure investments, countries can chart a path towards a more resilient, sustainable, and self-reliant energy future.
Wood Fuel:
Wood fuel refers to biomass material derived from trees and woody plants that is used as a source of energy for heating, cooking, electricity generation, and other applications. Wood has been used as a fuel for thousands of years and remains an important renewable energy resource, particularly in regions with abundant forest resources and where alternative energy sources may be limited or expensive.
There are several forms of wood fuel, including firewood, wood chips, wood pellets, and wood briquettes, each with its own characteristics and applications. Firewood, for example, consists of logs or branches that are cut and split for use in fireplaces, stoves, and traditional cooking methods. Wood chips are small pieces of wood produced from chipping or shredding larger wood pieces and are commonly used in biomass boilers and cogeneration plants.
Wood pellets are compressed biomass pellets made from sawdust, wood shavings, or other wood residues. They are uniform in size and shape, making them convenient for automated feeding systems in pellet stoves, boilers, and power plants. Wood pellets have a high energy density and low moisture content, making them an efficient and clean-burning fuel source.
Wood briquettes are densified biomass fuel blocks made from compressed sawdust, wood chips, or agricultural residues. They are similar to wood pellets but are larger in size and shape, often used in larger combustion systems such as industrial boilers and cogeneration plants.
Wood fuel offers several advantages as a renewable energy source. Wood is a locally available and abundant resource in many regions, reducing transportation costs and dependence on imported fuels. Harvesting and processing wood fuel can also create jobs and stimulate local economies, particularly in rural areas with forest resources.
Furthermore, wood fuel is carbon-neutral or carbon-negative when harvested sustainably. Trees absorb carbon dioxide (CO2) from the atmosphere as they grow, and when wood is burned for fuel, the CO2 released during combustion is offset by the carbon dioxide absorbed by new trees during regrowth. This cycle of carbon sequestration and release makes wood fuel a relatively low-carbon energy source compared to fossil fuels.
However, it is essential to ensure that wood fuel is harvested sustainably to prevent deforestation, habitat loss, and other environmental impacts. Sustainable forestry practices, such as selective harvesting, reforestation, and forest management certification programs, help maintain healthy forest ecosystems and ensure the long-term availability of wood fuel resources.
Overall, wood fuel remains an important renewable energy resource with significant potential for meeting energy needs in a sustainable and environmentally responsible manner. Continued investment in research, technology development, and forest management practices can further enhance the efficiency, affordability, and environmental sustainability of wood fuel utilization.
Gasification Plant:
A gasification plant is a facility designed to convert carbonaceous feedstocks, such as coal, biomass, or waste materials, into a gaseous fuel mixture known as syngas or producer gas through the process of gasification. Gasification plants play a crucial role in various industrial applications, including power generation, chemical production, and fuel synthesis, by providing a versatile and efficient method for converting diverse feedstocks into valuable energy products.
The operation of a gasification plant involves several key components and processes. Initially, the feedstock is prepared and processed to remove moisture and size it appropriately for feeding into the gasifier unit. Inside the gasifier, the feedstock undergoes thermochemical conversion at high temperatures and in a controlled environment with a limited supply of oxygen or steam.
Gasification reactions occur in several stages, including drying, pyrolysis, oxidation, and reduction, leading to the production of a syngas composed primarily of carbon monoxide (CO) and hydrogen (H2), along with carbon dioxide (CO2), methane (CH4), and other trace gases. The composition of the syngas can be adjusted by controlling operating parameters such as temperature, pressure, residence time, and the ratio of oxygen to feedstock.
After gasification, the raw syngas undergoes cleaning and conditioning processes to remove impurities such as tar, particulates, sulfur compounds, and moisture. This purified syngas can then be utilized for various energy applications, including electricity generation, heat production, and as a feedstock for the synthesis of liquid fuels and chemicals.
Gasification plants can be designed and configured in various ways to suit specific feedstock characteristics, operational requirements, and desired syngas applications. Common types of gasifiers include fixed-bed, fluidized bed, and entrained flow gasifiers, each offering unique advantages and limitations in terms of efficiency, scalability, and feedstock flexibility.
Fixed-bed gasifiers consist of a stationary bed of solid material through which the feedstock moves as it undergoes gasification reactions. Fluidized bed gasifiers suspend the feedstock particles in a bed of inert material, using a stream of gas to fluidize the bed and enhance heat and mass transfer. Entrained flow gasifiers entrain the feedstock particles in a high-velocity stream of oxidizing gas, resulting in rapid heating and conversion to syngas.
Gasification plants require careful planning, engineering, and operation to optimize performance, efficiency, and environmental sustainability. Control and monitoring systems are essential for maintaining stable operation and maximizing syngas quality while minimizing emissions and environmental impact.
Overall, gasification plants represent a versatile and environmentally sustainable technology for converting carbonaceous feedstocks into valuable energy products, contributing to the transition towards a more sustainable and low-carbon energy future. Continued innovation, investment, and collaboration are essential to further advance gasification technology and accelerate the adoption of renewable energy worldwide.
Syngas Generator:
A syngas generator is a device or system designed to produce synthesis gas (syngas) from various carbonaceous feedstocks, such as biomass, coal, or waste materials. Syngas generators play a crucial role in a wide range of industrial applications, including power generation, chemical synthesis, and fuel production, by providing a versatile and efficient method for converting diverse feedstocks into a valuable gaseous fuel mixture.
Syngas generators utilize gasification technology to convert solid or liquid carbonaceous materials into syngas through thermochemical processes. These processes typically involve subjecting the feedstock to high temperatures in a controlled environment with a limited supply of oxygen or steam, leading to partial oxidation and the production of a combustible gas mixture.
The operation of a syngas generator involves several key components and processes. Initially, the feedstock is prepared and processed to remove moisture and size it appropriately for feeding into the gasifier unit. Inside the gasifier, the feedstock undergoes gasification reactions, including drying, pyrolysis, oxidation, and reduction, resulting in the production of syngas composed primarily of carbon monoxide (CO) and hydrogen (H2), along with carbon dioxide (CO2), methane (CH4), and other trace gases.
Syngas generators can be designed and configured in various ways to suit specific feedstock characteristics, operational requirements, and desired syngas applications. Common types of syngas generators include fixed-bed, fluidized bed, and entrained flow gasifiers, each offering unique advantages and limitations in terms of efficiency, scalability, and feedstock flexibility.
After gasification, the raw syngas undergoes cleaning and conditioning processes to remove impurities such as tar, particulates, sulfur compounds, and moisture. This purified syngas can then be utilized for various energy applications, including electricity generation, heat production, and as a feedstock for the synthesis of liquid fuels and chemicals.
Syngas generators require careful planning, engineering, and operation to optimize performance, efficiency, and environmental sustainability. Control and monitoring systems are essential for maintaining stable operation and maximizing syngas quality while minimizing emissions and environmental impact.
Overall, syngas generators represent a versatile and environmentally sustainable technology for converting carbonaceous feedstocks into valuable energy products, contributing to the transition towards a more sustainable and low-carbon energy future. Continued innovation, investment, and collaboration are essential to further advance syngas generator technology and accelerate the adoption of renewable energy worldwide.
Syngas Utilization:
Syngas utilization refers to the conversion and application of synthesis gas (syngas) produced from gasification processes in various industrial, commercial, and residential applications. Syngas is a versatile fuel mixture composed primarily of carbon monoxide (CO) and hydrogen (H2), along with carbon dioxide (CO2), methane (CH4), and other trace gases, and can be used as a substitute for natural gas or as a feedstock for chemical synthesis processes.
One of the primary applications of syngas utilization is in power generation. Syngas can be burned directly in internal combustion engines, gas turbines, or fuel cells to produce electricity and heat. Combined heat and power (CHP) systems, also known as cogeneration plants, utilize syngas to generate both electricity and useful heat, increasing overall energy efficiency and reducing greenhouse gas emissions compared to separate electricity and heat generation systems.
Syngas can also be used as a feedstock for the production of liquid fuels and chemicals through various chemical synthesis processes. Fischer-Tropsch synthesis, for example, converts syngas into liquid hydrocarbons, such as synthetic diesel or jet fuel, as well as waxes and lubricants. Methanol synthesis converts syngas into methanol, which can be used as a fuel, solvent, or feedstock for chemical synthesis.
In addition to power generation and fuel production, syngas utilization encompasses a wide range of other applications, including:
- Heat production: Syngas can be burned directly in boilers or furnaces for space heating, water heating, or industrial process heating applications.
- Hydrogen production: Syngas can be further processed through water-gas shift reactions to produce hydrogen, which is used in various industrial processes, fuel cells, and transportation applications.
- Chemical production: Syngas serves as a precursor for the production of a wide range of chemicals, including ammonia, methanol, hydrogen cyanide, and synthetic natural gas (SNG).
- Environmental remediation: Syngas can be used in thermal destruction processes to treat hazardous waste materials and contaminated soils, leading to the destruction of organic pollutants and the recovery of energy.
Syngas utilization offers several advantages as a renewable energy and resource utilization strategy. It enables the efficient conversion of diverse carbonaceous feedstocks into valuable energy products and chemicals, reducing reliance on fossil fuels and promoting resource efficiency. By utilizing syngas in various applications, societies can reduce greenhouse gas emissions, enhance energy security, and stimulate economic development while addressing environmental and social challenges.
Overall, syngas utilization plays a vital role in the transition towards a more sustainable and low-carbon energy future, offering a versatile and environmentally responsible approach to energy production, chemical synthesis, and waste management. Continued research, development, and deployment efforts are essential to further optimize syngas utilization technologies, increase efficiency, and expand the range of applications, driving innovation and progress towards global sustainability goals.
Gasification Process:
Gasification is a thermochemical conversion process that transforms carbonaceous materials, such as biomass, coal, or waste, into a gaseous fuel mixture known as syngas or producer gas. This process involves subjecting the feedstock to high temperatures in a controlled environment with a limited supply of oxygen or steam, leading to partial oxidation and the production of a combustible gas mixture.
The gasification process typically consists of several interconnected stages. Initially, the feedstock is prepared and processed to remove moisture and size it appropriately for feeding into the gasifier unit. Inside the gasifier, the feedstock undergoes thermochemical reactions, including drying, pyrolysis, oxidation, and reduction, depending on the specific conditions and composition of the feedstock.
During the drying stage, moisture present in the feedstock is removed through evaporation, typically at temperatures ranging from 100°C to 200°C. Pyrolysis, or thermal decomposition, occurs at higher temperatures (300°C to 800°C) in the absence of oxygen, leading to the breakdown of complex organic molecules into simpler compounds, such as volatile gases, char, and tar.
As the temperature increases further, the volatile gases produced during pyrolysis react with a controlled supply of oxygen or steam to undergo gasification reactions. These reactions involve the conversion of carbonaceous materials into a mixture of gases, primarily carbon monoxide (CO) and hydrogen (H2), along with carbon dioxide (CO2), methane (CH4), and other trace gases.
The composition of the syngas produced depends on several factors, including the type of feedstock, gasification conditions (temperature, pressure, residence time), and the ratio of oxygen to feedstock. Control and optimization of these parameters are essential for maximizing syngas yield, quality, and energy content while minimizing undesirable by-products, such as tar and particulates.
After gasification, the raw syngas undergoes cleaning and conditioning processes to remove impurities such as tar, particulates, sulfur compounds, and moisture. This purified syngas can then be utilized for various energy applications, including electricity generation, heat production, and as a feedstock for the synthesis of liquid fuels and chemicals.
Gasification offers several advantages over conventional combustion processes, including higher efficiency, lower emissions, and greater flexibility in feedstock utilization. It enables the efficient conversion of diverse carbonaceous materials into valuable energy products, contributing to the transition towards a more sustainable and low-carbon energy future. Continued research, development, and deployment of gasification technologies are essential to further optimize performance, reduce costs, and expand the range of applications, driving innovation and progress towards global sustainability goals.
Syngas Production:
Syngas, short for synthesis gas, is a versatile fuel gas mixture primarily composed of carbon monoxide (CO) and hydrogen (H2), along with varying amounts of carbon dioxide (CO2), methane (CH4), and other trace gases. Syngas production is a key process in various industrial applications, including power generation, chemical synthesis, and fuel production.
The production of syngas can be achieved through several methods, with gasification being one of the primary techniques for biomass and coal feedstocks. In the context of biomass gasification, the syngas production process involves subjecting biomass feedstock, such as wood chips, agricultural residues, or energy crops, to high temperatures in a gasifier unit with controlled oxygen or steam supply.
During gasification, the biomass undergoes several thermochemical reactions, including pyrolysis and gasification, resulting in the conversion of solid biomass into a gaseous mixture of carbon monoxide, hydrogen, and other gases. The composition of the syngas produced can be adjusted by controlling various parameters such as temperature, residence time, and the ratio of oxygen to biomass.
Syngas production via gasification offers several advantages over conventional combustion processes. It allows for the efficient utilization of diverse biomass feedstocks, including waste materials and agricultural residues, reducing reliance on fossil fuels and contributing to carbon emission reduction goals. Additionally, syngas can be used as a feedstock for the production of synthetic fuels, such as methanol or synthetic natural gas (SNG), or for chemical synthesis processes, enabling the production of a wide range of valuable products.
The versatility of syngas makes it an attractive option for various energy and industrial applications. In power generation, syngas can be used directly in internal combustion engines, gas turbines, or fuel cells to produce electricity and heat. In chemical synthesis, syngas serves as a precursor for the production of fuels, chemicals, and other value-added products, offering opportunities for the integration of renewable resources into the production of high-value chemicals and materials.
Overall, syngas production plays a vital role in the transition towards a more sustainable and low-carbon energy future, offering a versatile and renewable alternative to conventional fossil fuels and enabling the efficient utilization of biomass resources for energy and industrial applications. Continued research and development efforts aim to further improve syngas production technologies, increase efficiency, and expand the range of feedstocks and applications, driving innovation in the field of renewable energy and resource utilization.
Biomass Gasification Process:
Biomass gasification is a thermochemical conversion process that converts biomass feedstock into a gaseous fuel mixture known as syngas or synthesis gas. This process involves subjecting biomass materials, such as wood chips, agricultural residues, or energy crops, to high temperatures in a gasifier unit with a controlled supply of oxygen, air, or steam, leading to partial oxidation and the production of a combustible gas mixture.
The biomass gasification process typically consists of several interconnected stages:
- Feedstock Preparation: Biomass feedstock is prepared by drying and sizing to reduce moisture content and ensure uniform feeding into the gasifier unit. The feedstock may undergo additional preprocessing, such as grinding or pelletizing, to improve handling and combustion characteristics.
- Gasification Reactions: Inside the gasifier unit, the biomass undergoes thermochemical reactions at elevated temperatures, typically ranging from 700°C to 1200°C, depending on the gasifier type and operating conditions. These reactions include:
- Drying: Moisture present in the biomass is evaporated and removed.
- Pyrolysis: Biomass undergoes thermal decomposition in the absence of oxygen, producing volatile gases, char, and tar.
- Gasification: Volatile gases produced during pyrolysis react with a controlled supply of oxygen or steam to undergo gasification reactions, resulting in the production of syngas composed primarily of carbon monoxide (CO) and hydrogen (H2), along with carbon dioxide (CO2), methane (CH4), and other trace gases.
- Syngas Cleanup: The raw syngas produced from the gasifier contains impurities such as tar, particulates, sulfur compounds, and moisture. Syngas cleanup processes, such as filtration, scrubbing, and catalytic conversion, are employed to remove these impurities and condition the syngas for downstream applications.
- Syngas Utilization: The purified syngas can be utilized for various energy applications, including:
- Electricity Generation: Syngas can be burned in internal combustion engines, gas turbines, or fuel cells to produce electricity.
- Heat Production: Syngas can be used for process heat, space heating, or water heating applications.
- Fuel Synthesis: Syngas can serve as a feedstock for the production of liquid fuels, such as synthetic diesel or methanol, through chemical synthesis processes.
Biomass gasification offers several advantages as a renewable energy technology. It enables the efficient utilization of diverse biomass feedstocks, reduces greenhouse gas emissions compared to fossil fuels, and provides a flexible and dispatchable energy source for electricity generation and heat production. Continued research and development efforts aim to improve gasification efficiency, reduce costs, and expand the range of biomass feedstocks and applications, driving innovation in the field of renewable energy and resource utilization.
Gasification Feedstock:
Gasification feedstock refers to the carbonaceous materials used as raw inputs in the gasification process to produce synthesis gas (syngas) or producer gas. These materials can include a wide range of biomass, coal, or waste-derived substances that undergo thermochemical conversion in a gasifier unit to generate a gaseous fuel mixture suitable for various energy and industrial applications.
- Biomass Feedstock:
- Wood: Wood chips, sawdust, bark, and other woody residues are commonly used biomass feedstocks in gasification. Wood biomass is readily available, renewable, and can be sustainably harvested from forests or generated as a byproduct of forestry and wood processing industries.
- Agricultural Residues: Crop residues, such as corn stover, rice husks, wheat straw, and sugarcane bagasse, can be used as biomass feedstock for gasification. These residues are generated during agricultural harvesting and processing operations and provide a renewable and locally available source of biomass.
- Energy Crops: Dedicated energy crops, such as switchgrass, miscanthus, and willow, are grown specifically for use as biomass feedstock in bioenergy applications, including gasification. These crops offer high biomass yields and can be cultivated on marginal lands without competing with food crops.
- Organic Waste: Organic waste materials, such as municipal solid waste (MSW), sewage sludge, and animal manure, can be used as feedstock for gasification. Gasification of organic waste offers a sustainable waste management solution while generating renewable energy and reducing landfill disposal.
- Coal Feedstock:
- Bituminous Coal: Bituminous coal is a commonly used coal feedstock in gasification due to its relatively high carbon content and energy density. Gasification of bituminous coal can produce syngas rich in carbon monoxide and hydrogen, suitable for various energy and chemical applications.
- Sub-bituminous Coal: Sub-bituminous coal is another type of coal feedstock used in gasification processes. It contains less carbon and more moisture than bituminous coal but can still be utilized for syngas production with appropriate gasification technology and process conditions.
- Lignite: Lignite, also known as brown coal, is a low-rank coal feedstock with high moisture content and low energy density. Gasification of lignite requires specialized gasification technologies to handle its unique properties and produce syngas with desired composition and quality.
- Waste-derived Feedstock:
- Refuse-Derived Fuel (RDF): RDF is a processed solid fuel derived from municipal solid waste (MSW) through mechanical and biological treatment methods. RDF can be used as a feedstock for gasification to produce syngas while diverting waste from landfill disposal and reducing greenhouse gas emissions.
- Biomass Residues: Various waste biomass residues, such as forestry residues, agricultural residues, and food processing waste, can be used as feedstock for gasification. Gasification of biomass residues offers a sustainable waste management solution while generating renewable energy and valuable by-products.
Gasification feedstock selection depends on factors such as feedstock availability, cost, energy content, environmental impact, and desired syngas composition. Different feedstocks require specific gasification technologies and process conditions to optimize syngas production efficiency, quality, and environmental performance. Additionally, feedstock characteristics may influence gasifier design, operational parameters, and syngas utilization options, highlighting the importance of feedstock compatibility and integration in gasification systems.
Fixed-Bed Gasifier:
A fixed-bed gasifier is a type of gasification reactor where the feedstock remains stationary within the gasification chamber while undergoing thermochemical conversion to produce syngas. This gasification technology operates on the principle of counter-current flow, where the feedstock moves downward through the gasifier chamber while the oxidizing agent (such as air, oxygen, or steam) flows upward through the bed of solid material.
The operation of a fixed-bed gasifier involves several key components and processes:
- Feedstock Preparation: Biomass feedstock, such as wood chips, agricultural residues, or energy crops, is prepared and sized appropriately for feeding into the gasifier. The feedstock may undergo preprocessing steps, such as drying and size reduction, to optimize gasification performance.
- Gasification Chamber: The gasification chamber of a fixed-bed gasifier typically consists of a refractory-lined vessel with a fixed grate or hearth at the bottom to support the stationary bed of feedstock. The chamber is designed to withstand high temperatures and pressure conditions during gasification.
- Gasification Reactions: Inside the gasification chamber, the feedstock undergoes thermochemical reactions, including drying, pyrolysis, oxidation, and reduction, as it descends through the bed of solid material. These reactions are facilitated by the controlled supply of oxidizing agent and heat to the gasifier.
- Syngas Collection: As the feedstock undergoes gasification reactions, syngas is produced and rises through the bed of solid material. The syngas is collected at the top of the gasifier chamber and then directed to downstream processing units for cleanup and conditioning.
Fixed-bed gasifiers offer several advantages and limitations compared to other gasification technologies:
Advantages:
- Simple design and operation: Fixed-bed gasifiers have a relatively simple design and are easier to operate compared to other gasification technologies.
- Suitable for diverse feedstocks: Fixed-bed gasifiers can accommodate a wide range of biomass feedstocks, including wood chips, agricultural residues, and energy crops.
- High carbon conversion efficiency: Fixed-bed gasifiers can achieve high carbon conversion efficiency due to the long residence time of the feedstock in the gasification chamber.
Limitations:
- Limited scalability: Fixed-bed gasifiers are typically smaller in scale compared to fluidized bed or entrained flow gasifiers, making them less suitable for large-scale industrial applications.
- Ash handling: Fixed-bed gasifiers produce ash as a by-product of gasification, which needs to be removed periodically to maintain gasifier performance.
- Tar formation: Fixed-bed gasifiers are prone to tar formation, which can lead to operational challenges and require additional syngas cleanup steps.
Overall, fixed-bed gasifiers represent a viable option for small to medium-scale biomass gasification applications, offering simplicity, versatility, and high carbon conversion efficiency. Continued research and development efforts aim to further optimize fixed-bed gasification technology and address its limitations to expand its potential applications in renewable energy and resource utilization.
Fluidized Bed Gasifier:
A fluidized bed gasifier is a type of gasification reactor where the feedstock is suspended and fluidized by an upward flow of gas (usually air or steam) through a bed of inert material, such as sand or ash. This gasification technology operates on the principle of fluidization, where the solid particles behave like a fluid under the influence of the upward gas flow, facilitating efficient heat and mass transfer for gasification reactions.
The operation of a fluidized bed gasifier involves several key components and processes:
- Feedstock Injection: Biomass feedstock, such as wood chips, agricultural residues, or energy crops, is injected into the bottom of the fluidized bed reactor. The feedstock enters the bed of inert material and becomes suspended and fluidized by the upward flow of gas.
- Fluidization: As the gas flows upward through the bed of inert material, it imparts kinetic energy to the solid particles, causing them to move and behave like a fluid. This fluidization process creates a homogeneous mixing of the feedstock and inert material, ensuring uniform heat distribution and gas-solid contact for efficient gasification.
- Gasification Reactions: Inside the fluidized bed reactor, the feedstock undergoes thermochemical reactions, including drying, pyrolysis, oxidation, and reduction, as it interacts with the high-temperature gas environment. These reactions lead to the production of syngas, composed primarily of carbon monoxide (CO) and hydrogen (H2), along with other gases such as carbon dioxide (CO2) and methane (CH4).
- Syngas Collection: The syngas produced during gasification rises through the fluidized bed reactor and is collected at the top of the reactor vessel. The syngas is then directed to downstream processing units for cleanup and conditioning before utilization in various energy or industrial applications.
Fluidized bed gasifiers offer several advantages and limitations compared to other gasification technologies:
Advantages:
- Enhanced heat and mass transfer: Fluidized bed gasifiers provide efficient heat and mass transfer between the gas and solid phases, resulting in rapid gasification reactions and high syngas yields.
- Fuel flexibility: Fluidized bed gasifiers can accommodate a wide range of feedstocks, including biomass, coal, and waste materials, making them versatile for different applications and feedstock availability.
- Reduced tar formation: The fluidized bed environment helps to minimize tar formation during gasification, leading to cleaner syngas production and reduced downstream cleanup requirements.
Limitations:
- Complexity: Fluidized bed gasifiers are more complex in design and operation compared to fixed-bed gasifiers, requiring precise control of gas flow rates, temperatures, and residence times to maintain stable operation.
- Higher capital costs: The construction and operation of fluidized bed gasifiers may require higher capital investment compared to simpler gasification technologies, limiting their adoption in certain applications.
- Ash management: Fluidized bed gasifiers produce ash as a by-product of gasification, which needs to be managed and removed periodically to prevent bed agglomeration and maintain reactor performance.
Overall, fluidized bed gasifiers represent a promising option for biomass and waste gasification applications, offering enhanced efficiency, fuel flexibility, and reduced tar formation compared to other gasification technologies. Continued research and development efforts aim to further optimize fluidized bed gasification technology and expand its potential applications in renewable energy and resource utilization.
Entrained Flow Gasifier:
An entrained flow gasifier is a type of gasification reactor where finely ground feedstock particles are suspended and entrained in a high-velocity stream of oxidizing gas (such as oxygen or air) within a refractory-lined vessel. This gasification technology operates on the principle of rapid heating and partial oxidation of the feedstock particles as they travel through the gasifier, leading to the production of a high-quality syngas mixture.
The operation of an entrained flow gasifier involves several key components and processes:
- Feedstock Injection: Finely ground biomass feedstock, coal, or waste materials are injected into the gasifier vessel through multiple injection points, typically using pneumatic conveyance systems. The feedstock particles are entrained in a stream of oxidizing gas and transported into the gasification zone.
- Gasification Zone: Inside the gasifier vessel, the feedstock particles are rapidly heated and partially oxidized as they travel through the high-temperature gasification zone. The intense heat and turbulent gas flow promote efficient gas-solid interactions and rapid conversion of the feedstock into syngas.
- Syngas Formation: The partial oxidation of the feedstock particles results in the production of syngas, composed primarily of carbon monoxide (CO) and hydrogen (H2), along with other gases such as carbon dioxide (CO2) and methane (CH4). The syngas is generated in a highly controlled environment, allowing for precise control of syngas composition and quality.
- Syngas Cooling and Cleanup: The hot syngas produced in the gasification zone is rapidly cooled to prevent further gasification reactions and condensation of tar and other impurities. Syngas cleanup processes, such as filtration, scrubbing, and catalytic conversion, are employed to remove impurities and condition the syngas for downstream applications.
Entrained flow gasifiers offer several advantages and limitations compared to other gasification technologies:
Advantages:
- High efficiency: Entrained flow gasifiers can achieve high syngas production efficiency and high carbon conversion rates due to the intense heat and rapid gas-solid interactions in the gasification zone.
- Wide feedstock flexibility: Entrained flow gasifiers can process a wide range of feedstocks, including biomass, coal, and waste materials, making them versatile for different applications and feedstock availability.
- Precise control: Entrained flow gasifiers offer precise control of gasification parameters, such as temperature, residence time, and oxidant feed rates, allowing for tailored syngas composition and quality.
Limitations:
- High operating costs: Entrained flow gasifiers may require higher operating costs compared to other gasification technologies due to the need for high-temperature refractory materials, specialized equipment, and energy-intensive gasification processes.
- Complex design and operation: Entrained flow gasifiers are more complex in design and operation compared to fixed-bed or fluidized bed gasifiers, requiring advanced control systems and process optimization to maintain stable operation.
- Tar formation: Entrained flow gasifiers are susceptible to tar formation, particularly at lower oxygen-to-feedstock ratios, which can lead to operational challenges and require additional syngas cleanup steps.
Overall, entrained flow gasifiers represent a promising option for high-efficiency gasification of biomass, coal, and waste feedstocks, offering precise control, wide feedstock flexibility, and high syngas quality. Continued research and development efforts aim to further optimize entrained flow gasification technology and address its limitations to expand its potential applications in renewable energy and resource utilization.
Biomass
Biomass refers to organic matter derived from plants, animals, or microorganisms that can be used as a source of renewable energy. This diverse category includes a wide range of materials, such as wood, agricultural crops and residues, organic waste, algae, and dedicated energy crops. Biomass is considered renewable because it can be replenished relatively quickly through natural processes, such as photosynthesis, compared to fossil fuels, which require millions of years to form.
The use of biomass for energy dates back thousands of years, with early humans burning wood for heat and cooking. Today, biomass plays a vital role in the global energy mix, providing heat, electricity, and transportation fuels in a sustainable and environmentally friendly manner.
One of the key advantages of biomass is its carbon-neutral nature. When biomass is burned or converted into biofuels, it releases carbon dioxide (CO2) into the atmosphere. However, this carbon dioxide was originally absorbed from the atmosphere by the plants during photosynthesis. As a result, the net carbon emissions from biomass combustion are roughly equivalent to the carbon dioxide absorbed during the growth of the biomass, making it a carbon-neutral energy source when managed sustainably.
Biomass can be utilized in various forms and technologies to generate energy:
- Direct combustion: Biomass can be burned directly to produce heat or electricity in boilers, furnaces, or power plants. Wood chips, pellets, and agricultural residues are commonly used as fuels in biomass combustion systems.
- Biofuels: Biomass can be converted into liquid or gaseous biofuels through biochemical or thermochemical processes. Examples include bioethanol produced from corn or sugarcane, biodiesel from vegetable oils or animal fats, and biogas from anaerobic digestion of organic waste.
- Biopower: Biomass power plants generate electricity by burning biomass fuels to produce steam, which drives turbines connected to generators. These power plants can use a variety of biomass feedstocks, including wood, agricultural residues, and municipal solid waste.
- Biogas: Biogas is produced through the anaerobic digestion of organic materials, such as food waste, animal manure, and sewage sludge. The methane-rich biogas can be used directly as a fuel for heating, electricity generation, or transportation, or upgraded to biomethane for injection into natural gas pipelines.
- Biochar: Pyrolysis of biomass at high temperatures in the absence of oxygen produces biochar, a carbon-rich material that can be used as a soil amendment to improve soil fertility and sequester carbon.
The utilization of biomass for energy offers several environmental, social, and economic benefits. It helps reduce greenhouse gas emissions by displacing fossil fuels, promotes rural development and job creation through biomass production and processing, and enhances energy security by diversifying energy sources.
However, biomass energy also presents challenges, such as competition for land and resources with food production, biodiversity loss, air and water pollution from biomass combustion, and the need for sustainable management practices to ensure long-term environmental and social benefits.
Overall, biomass represents a valuable renewable energy resource with the potential to play a significant role in the transition to a more sustainable and low-carbon energy future. Continued research, development, and deployment of biomass technologies are essential to maximize its potential while minimizing its environmental and social impacts.
Renewable Energy:
Renewable energy refers to energy derived from naturally replenishing sources that are continually available and can be used without depleting the Earth’s resources. Unlike fossil fuels, which are finite and non-renewable, renewable energy sources are abundant and sustainable over the long term. The utilization of renewable energy is essential for reducing greenhouse gas emissions, mitigating climate change, and ensuring energy security and independence.
Key renewable energy sources include:
- Solar Energy: Solar energy is harnessed from the sun’s radiation using photovoltaic (PV) panels or solar thermal systems. PV panels convert sunlight directly into electricity, while solar thermal systems use sunlight to heat water or other fluids to generate steam for electricity generation or space heating.
- Wind Energy: Wind energy is generated by harnessing the kinetic energy of wind through wind turbines. Wind turbines convert the rotational motion of wind-driven blades into electricity through a generator, which can be used for various applications, from powering homes and businesses to supplying electricity to the grid.
- Hydropower: Hydropower utilizes the gravitational force of flowing or falling water to generate electricity. It is one of the oldest and most widely used renewable energy sources, with hydroelectric power plants harnessing the energy of rivers, dams, and tidal currents to produce electricity.
- Biomass Energy: Biomass energy is derived from organic materials, such as wood, agricultural residues, and organic waste, through processes such as combustion, anaerobic digestion, and gasification. Biomass can be used for heat, electricity, and transportation fuels, offering a versatile and renewable energy source.
- Geothermal Energy: Geothermal energy is heat extracted from the Earth’s subsurface, typically through the use of geothermal heat pumps or geothermal power plants. Geothermal power plants harness the heat from underground reservoirs of hot water or steam to generate electricity.
- Ocean Energy: Ocean energy encompasses various technologies that harness the energy of the ocean’s waves, tides, currents, and temperature gradients. Ocean energy technologies include tidal turbines, wave energy converters, ocean thermal energy conversion (OTEC) systems, and salinity gradient power systems.
Renewable energy offers numerous environmental, economic, and social benefits compared to fossil fuels. It reduces greenhouse gas emissions, air and water pollution, and dependency on imported fossil fuels, while creating jobs, stimulating economic growth, and enhancing energy security and resilience. Additionally, renewable energy sources are inherently decentralized and can be deployed at various scales, from small-scale distributed generation systems to large utility-scale power plants.
Despite these advantages, the widespread adoption of renewable energy faces challenges such as intermittency, variability, and grid integration issues, as well as upfront costs and policy and regulatory barriers. Overcoming these challenges requires continued innovation, investment, and supportive policies to accelerate the transition towards a sustainable and low-carbon energy future powered by renewable energy.
Organic Matter:
Organic matter refers to any material that originates from living organisms and contains carbon compounds. It is a fundamental component of the Earth’s biosphere and plays a crucial role in various biological, ecological, and geological processes. Organic matter encompasses a wide range of materials, including plants, animals, microorganisms, and their by-products, such as decaying plant material, animal waste, and microbial biomass.
In soils, organic matter contributes to soil fertility, structure, and nutrient cycling, influencing plant growth and ecosystem productivity. Organic matter acts as a reservoir for nutrients, water, and carbon, providing essential resources for soil organisms and plants. It improves soil structure by promoting aggregation and porosity, enhancing water infiltration, retention, and drainage, and reducing erosion and compaction.
Organic matter decomposition is a key process in the carbon cycle, where organic carbon compounds are broken down by soil microbes and converted into simpler compounds, such as carbon dioxide (CO2) and methane (CH4), through microbial respiration. This process releases energy and nutrients stored in organic matter back into the soil and atmosphere, fueling ecosystem productivity and supporting life.
In addition to soils, organic matter is found in various terrestrial and aquatic ecosystems, including forests, wetlands, grasslands, and marine environments. It serves as a source of energy and nutrients for diverse organisms, from bacteria and fungi to plants and animals, forming the basis of food webs and ecosystem functioning.
In agriculture, organic matter management is essential for maintaining soil health and fertility, enhancing crop productivity, and mitigating climate change. Practices such as crop rotation, cover cropping, composting, and reduced tillage can increase organic matter content in soils, improve soil structure and fertility, and sequester carbon from the atmosphere, contributing to climate change mitigation efforts.
Organic matter also plays a significant role in waste management and renewable energy production. Organic waste materials, such as food scraps, yard trimmings, and animal manure, can be composted or anaerobically digested to produce compost, biogas, or biofuels. These bioenergy technologies not only divert organic waste from landfills, reducing methane emissions, but also generate renewable energy and valuable soil amendments for agriculture.
Overall, organic matter is a vital component of Earth’s ecosystems, supporting biodiversity, soil fertility, and ecosystem services, while also serving as a valuable resource for agriculture, waste management, and renewable energy production. Sustainable management and conservation of organic matter are essential for maintaining ecosystem health, food security, and environmental sustainability in the face of global environmental challenges.
Carbon-Neutral:
Carbon neutrality, also known as net-zero carbon emissions, refers to achieving a balance between the amount of carbon dioxide (CO2) emitted into the atmosphere and the amount removed or offset through carbon sequestration or emission reduction measures. In a carbon-neutral state, the net carbon emissions are effectively zero, meaning that any CO2 emitted is balanced by an equivalent amount removed from the atmosphere, typically through natural or technological means.
The concept of carbon neutrality is central to efforts to mitigate climate change and reduce greenhouse gas emissions. The combustion of fossil fuels, such as coal, oil, and natural gas, releases CO2 and other greenhouse gases into the atmosphere, contributing to global warming and climate disruption. By achieving carbon neutrality, societies can minimize their impact on the climate and transition to a more sustainable and resilient future.
Carbon neutrality can be achieved through various strategies and approaches:
- Reducing Emissions: One of the primary methods for achieving carbon neutrality is to reduce greenhouse gas emissions from human activities, particularly those associated with energy production, transportation, industry, and agriculture. This can be accomplished through the adoption of energy-efficient technologies, renewable energy sources, electrification, and sustainable land use and management practices.
- Carbon Sequestration: Carbon sequestration involves capturing and storing CO2 from the atmosphere or preventing its release from sources such as power plants and industrial facilities. Natural carbon sinks, such as forests, wetlands, and soils, can sequester carbon through photosynthesis and organic matter accumulation. Additionally, technological approaches, such as carbon capture and storage (CCS) and direct air capture (DAC), can capture CO2 emissions from point sources and store them underground or utilize them for industrial processes.
- Offsetting Emissions: Carbon offsetting involves investing in projects or activities that reduce or remove carbon emissions elsewhere, thereby compensating for emissions that cannot be avoided. Examples of carbon offset projects include reforestation and afforestation initiatives, renewable energy projects, methane capture from landfills and livestock operations, and energy efficiency improvements in developing countries.
- Renewable Energy Transition: Transitioning to renewable energy sources, such as solar, wind, hydropower, and biomass, can significantly reduce carbon emissions associated with fossil fuel combustion for electricity generation, heating, and transportation. By replacing fossil fuels with clean and renewable energy sources, societies can decarbonize their energy systems and move closer to carbon neutrality.
- Behavioral Changes: Individuals, businesses, and organizations can contribute to carbon neutrality through changes in behavior and lifestyle choices, such as reducing energy consumption, adopting sustainable transportation options, minimizing waste generation, and supporting carbon offset initiatives.
Achieving carbon neutrality requires coordinated efforts and collaboration across sectors and stakeholders, including governments, businesses, civil society organizations, and individuals. It involves setting ambitious emission reduction targets, implementing effective policies and regulations, investing in clean technologies and infrastructure, and fostering public awareness and engagement.
While achieving complete carbon neutrality may be challenging in the short term, incremental steps towards reducing emissions and enhancing carbon sinks can help mitigate climate change impacts and build a more sustainable and resilient future for current and future generations. Carbon neutrality represents a critical pathway towards addressing the climate crisis and achieving global climate goals, such as those outlined in the Paris Agreement.
Bioenergy:
Bioenergy refers to energy derived from organic materials, such as biomass, biofuels, and biogas, through various conversion processes. It is a versatile and renewable energy source that plays a significant role in the global energy mix, offering a sustainable alternative to fossil fuels and contributing to climate change mitigation efforts.
Bioenergy is derived from biomass, which includes a wide range of organic materials derived from plants, animals, and microorganisms. Biomass feedstocks can be categorized into several types, including:
- Woody Biomass: Derived from trees and woody plants, woody biomass includes materials such as wood chips, sawdust, bark, and forest residues. It is commonly used for heat, electricity generation, and biofuel production.
- Agricultural Residues: Agricultural residues are by-products of crop cultivation and include materials such as crop residues (e.g., straw, stalks), husks, shells, and bagasse. These residues can be used for heat, power generation, and biofuel production.
- Energy Crops: Dedicated energy crops are grown specifically for bioenergy production and include species such as switchgrass, miscanthus, willow, and poplar. These crops offer high biomass yields and can be cultivated on marginal lands without competing with food crops.
- Organic Waste: Organic waste materials, including food waste, yard trimmings, animal manure, and sewage sludge, can be converted into biogas through anaerobic digestion. Biogas is a renewable fuel composed primarily of methane and carbon dioxide, which can be used for heat, electricity generation, and transportation.
Bioenergy can be produced through various conversion processes, including:
- Combustion: Biomass can be burned directly to produce heat or electricity in boilers, furnaces, or power plants. Combustion releases energy by oxidizing biomass materials and converting them into heat, which can be used for heating buildings, generating steam, or driving turbines connected to generators.
- Biochemical Conversion: Biochemical conversion processes, such as fermentation and enzymatic hydrolysis, involve breaking down biomass feedstocks into sugars, which can then be converted into biofuels such as ethanol, biodiesel, and biobutanol. These biofuels can be used as transportation fuels or blended with conventional fuels to reduce greenhouse gas emissions.
- Thermochemical Conversion: Thermochemical conversion processes, such as pyrolysis and gasification, involve heating biomass feedstocks in the absence of oxygen to produce bio-oil, syngas, or biochar. These intermediate products can be further processed into liquid fuels, hydrogen, or synthetic gases for various energy applications.
Bioenergy offers several environmental, economic, and social benefits compared to fossil fuels. It is renewable, carbon-neutral, and helps reduce greenhouse gas emissions, air pollution, and dependence on imported fossil fuels. Additionally, bioenergy production contributes to rural development, job creation, and energy security by utilizing locally available biomass resources and promoting sustainable land use practices.
However, bioenergy production also presents challenges and considerations, including land competition with food crops, biodiversity impacts, water usage, and sustainability concerns related to biomass sourcing and land management practices. Addressing these challenges requires careful planning, sustainable practices, and regulatory frameworks to ensure that bioenergy production contributes to sustainable development goals and environmental conservation efforts.
Overall, bioenergy represents a valuable and promising renewable energy source with the potential to play a significant role in the transition to a more sustainable and low-carbon energy future. Continued research, development, and deployment of bioenergy technologies are essential to maximize its potential while minimizing its environmental and social impacts.
Sustainable Fuel:
Sustainable fuel refers to any type of fuel that is produced, processed, and utilized in a manner that minimizes environmental impact, preserves natural resources, and supports social and economic well-being over the long term. It is an essential component of efforts to transition to a more sustainable and environmentally friendly energy system, reducing greenhouse gas emissions, mitigating climate change, and promoting energy security and resilience.
Key characteristics of sustainable fuels include:
- Renewability: Sustainable fuels are derived from renewable resources that can be replenished or regenerated within a relatively short time frame. Unlike fossil fuels, which are finite and non-renewable, sustainable fuels are sourced from biomass, solar energy, wind energy, hydropower, and other renewable sources.
- Low Carbon Intensity: Sustainable fuels have lower carbon intensity compared to conventional fossil fuels, resulting in reduced greenhouse gas emissions over their lifecycle. By minimizing carbon emissions, sustainable fuels help mitigate climate change and contribute to global efforts to limit global warming to well below 2 degrees Celsius above pre-industrial levels.
- Resource Efficiency: Sustainable fuel production processes emphasize resource efficiency and optimization to minimize waste generation, energy consumption, and environmental impact. Technologies such as bioenergy, solar photovoltaics, and wind turbines strive to maximize energy conversion efficiency and minimize resource use, land footprint, and water usage.
- Environmental Compatibility: Sustainable fuels are produced and utilized in a manner that minimizes adverse environmental impacts, such as air and water pollution, habitat destruction, and biodiversity loss. Technologies such as carbon capture and storage (CCS), biochar production, and sustainable forestry practices help mitigate environmental risks and enhance ecosystem resilience.
- Social Responsibility: Sustainable fuel production and utilization prioritize social responsibility, equity, and inclusivity, ensuring that the benefits of renewable energy are shared equitably among communities, stakeholders, and future generations. This includes promoting access to clean energy, creating green jobs, supporting local economies, and empowering marginalized communities.
Examples of sustainable fuels include:
- Biofuels: Biofuels such as ethanol, biodiesel, and biogas are derived from biomass feedstocks such as corn, sugarcane, soybeans, algae, and organic waste. They offer a renewable alternative to conventional fossil fuels for transportation, heating, and electricity generation.
- Hydrogen: Hydrogen produced from renewable sources such as water electrolysis using renewable electricity or biomass gasification with carbon capture and storage (CCS) offers a clean and sustainable fuel option for various applications, including transportation, industry, and energy storage.
- Renewable Electricity: Electricity generated from renewable sources such as solar photovoltaics (PV), wind turbines, hydropower, and geothermal energy is considered a sustainable fuel for powering electric vehicles, heating buildings, and supplying clean energy to the grid.
- Synthetic Fuels: Synthetic fuels produced from renewable hydrogen and captured CO2, such as synthetic methane, synthetic gasoline, and synthetic jet fuel, offer carbon-neutral alternatives to conventional fossil fuels for transportation and industrial applications.
Achieving a sustainable fuel future requires collaboration and innovation across sectors, including government, industry, academia, and civil society. It involves investing in research, development, and deployment of renewable energy technologies, implementing supportive policies and regulations, and fostering public awareness and engagement. By transitioning to sustainable fuels, societies can reduce their environmental footprint, enhance energy security, and build a more resilient and equitable future for all.
Biomass Feedstock:
Biomass feedstock refers to the organic materials used as raw inputs for bioenergy production, including biomass-based fuels, heat, power, and bioproducts. These feedstocks are derived from a variety of renewable sources, such as plants, trees, agricultural residues, and organic waste, and play a crucial role in supporting the sustainability and viability of bioenergy systems.
Common types of biomass feedstock include:
- Woody Biomass: Woody biomass consists of woody materials derived from trees and shrubs, including branches, tops, bark, and wood chips. It is widely used for bioenergy production due to its abundance, energy density, and suitability for combustion, gasification, and pyrolysis processes. Woody biomass feedstock can be sourced from forestry residues, logging operations, urban wood waste, and dedicated energy crops such as willow and poplar.
- Agricultural Residues: Agricultural residues are by-products of crop cultivation and include materials such as straw, stalks, husks, shells, and bagasse. These residues are generated during the harvesting and processing of crops such as wheat, rice, corn, sugarcane, and soybeans. Agricultural residues are valuable biomass feedstocks for bioenergy production, offering high energy content and availability while reducing waste and environmental impact in agricultural operations.
- Energy Crops: Energy crops are dedicated biomass crops grown specifically for bioenergy production on marginal lands unsuitable for food crops. Examples of energy crops include switchgrass, miscanthus, sorghum, and giant reed. These crops are selected for their fast growth, high biomass yields, low input requirements, and adaptability to diverse climates and soil conditions. Energy crops can be cultivated as monocultures or incorporated into crop rotation systems to enhance soil fertility and productivity.
- Organic Waste: Organic waste materials, such as food waste, yard trimmings, animal manure, and sewage sludge, represent valuable biomass feedstocks for bioenergy production through anaerobic digestion and composting processes. Anaerobic digestion converts organic waste into biogas, a renewable fuel composed primarily of methane and carbon dioxide, which can be used for heat, power generation, and transportation. Composting processes convert organic waste into compost, a nutrient-rich soil amendment used in agriculture and landscaping.
- Algae: Algae are photosynthetic microorganisms that can be cultivated in open ponds or closed bioreactors to produce biomass for bioenergy production. Algae biomass is rich in lipids, proteins, and carbohydrates, which can be converted into biofuels such as biodiesel, bioethanol, and biogasoline through various conversion processes. Algae cultivation offers several advantages, including high productivity, rapid growth rates, wastewater treatment capabilities, and potential carbon capture and utilization.
Biomass feedstocks play a critical role in supporting the sustainability, availability, and cost-effectiveness of bioenergy systems. The selection of appropriate feedstocks depends on factors such as regional biomass availability, feedstock characteristics, conversion technologies, energy requirements, environmental considerations, and economic viability. Sustainable biomass feedstock management practices, including cultivation, harvesting, transportation, and storage, are essential to ensure the long-term viability and environmental integrity of bioenergy supply chains. By harnessing the diverse potential of biomass feedstocks, bioenergy can contribute to climate change mitigation, energy security, rural development, and environmental sustainability goals.
Energy Crops:
Energy crops are specialized plants cultivated specifically for the production of bioenergy, including biofuels, biogas, and biomass for heat and electricity generation. These crops are selected and grown for their high biomass yield, fast growth rates, low input requirements, and adaptability to various climatic and soil conditions. Energy crops play a crucial role in sustainable bioenergy production by providing a renewable and carbon-neutral source of energy while minimizing competition with food crops and natural habitats.
Some common types of energy crops include:
- Switchgrass (Panicum virgatum): Switchgrass is a perennial warm-season grass native to North America and widely cultivated for bioenergy production. It is known for its high biomass yield, drought tolerance, and adaptability to marginal lands. Switchgrass can be harvested for biofuel production, such as cellulosic ethanol, or used as feedstock for biomass power plants and biogas production through anaerobic digestion.
- Miscanthus (Miscanthus spp.): Miscanthus is a genus of perennial grasses native to Asia and widely grown as an energy crop in Europe and North America. It is valued for its high biomass productivity, low input requirements, and tolerance to diverse soil and climate conditions. Miscanthus can be used for biofuel production, such as ethanol and biogas, or as a feedstock for biomass-fired power plants and combined heat and power (CHP) systems.
- Willow (Salix spp.): Willow is a genus of deciduous shrubs and trees native to temperate regions of the Northern Hemisphere. It is cultivated for biomass production due to its rapid growth, high biomass yield, and ability to coppice (re-grow after harvest). Willow biomass can be used for heat and electricity generation, biofuel production, soil erosion control, phytoremediation, and wildlife habitat enhancement.
- Poplar (Populus spp.): Poplar is a genus of fast-growing deciduous trees native to the Northern Hemisphere and widely cultivated as an energy crop. It is valued for its high biomass productivity, short rotation period, and versatility for various bioenergy applications. Poplar biomass can be utilized for heat and power generation, biofuel production, pulp and paper manufacturing, and carbon sequestration in afforestation and reforestation projects.
- Sorghum (Sorghum bicolor): Sorghum is a cereal grain crop cultivated worldwide for food, feed, and bioenergy production. It is grown for its grain, which can be used for food and feed purposes, as well as its biomass, which can be converted into biofuels such as ethanol and biogas. Sweet sorghum varieties, with high sugar content in the stalks, are particularly suitable for biofuel production through fermentation and distillation processes.
Energy crops offer several advantages for bioenergy production compared to traditional food crops and fossil fuels:
- High Biomass Yield: Energy crops are selected and bred for their high biomass productivity, allowing for efficient conversion into biofuels, biogas, and biomass-based energy.
- Low Input Requirements: Many energy crops require minimal inputs such as water, fertilizer, and pesticides, reducing the environmental impact and cost of cultivation.
- Marginal Lands Utilization: Energy crops can be grown on marginal lands unsuitable for food crops, including degraded soils, abandoned farmland, and reclaimed mine sites, minimizing competition with food production and natural habitats.
- Carbon Sequestration: Energy crops contribute to carbon sequestration by removing CO2 from the atmosphere through photosynthesis and storing carbon in biomass and soil organic matter, mitigating climate change and enhancing soil fertility and resilience.
However, energy crop cultivation also presents challenges and considerations, including land use change, water usage, biodiversity impacts, soil erosion, and socio-economic implications for landowners, farmers, and local communities. Sustainable energy crop management practices, including site selection, crop rotation, soil conservation, and biodiversity conservation measures, are essential to maximize the environmental and social benefits of energy crop cultivation while minimizing potential risks and trade-offs. By harnessing the potential of energy crops in bioenergy production, societies can advance towards a more sustainable, resilient, and low-carbon energy future.
Agricultural Residues:
Agricultural residues are organic materials left over from agricultural activities, including crop cultivation, harvesting, and processing. These residues consist of various plant parts such as stalks, stems, leaves, husks, shells, and straw, which remain after the primary crop has been harvested or processed for its primary purpose (e.g., food, fiber, or seed production). Agricultural residues are abundant, widely available, and represent a valuable source of biomass for bioenergy production, soil amendment, animal bedding, and other agricultural and industrial applications.
Some common types of agricultural residues include:
- Crop Residues: Crop residues are by-products of crop cultivation and include materials such as stalks, stems, leaves, and chaff left in the field after harvesting. Examples of crop residues include corn stalks, wheat straw, rice husks, soybean stubble, and sugarcane bagasse. Crop residues are generated in large quantities worldwide and are typically left on the field as organic mulch or tilled back into the soil to improve soil fertility and structure.
- Husks and Shells: Husks and shells are outer coverings of seeds and fruits and include materials such as rice husks, corn cobs, peanut shells, and coconut husks. These agricultural residues are produced during grain and oilseed processing operations and are often considered waste or by-products. However, they contain valuable biomass and can be utilized for various purposes, including energy production, animal feed, composting, and industrial applications.
- Straw: Straw is the dried stalks of cereal crops such as wheat, barley, oats, and rye left over after grain harvest. It is a fibrous and lignocellulosic material with high cellulose and hemicellulose content, making it suitable for bioenergy production through combustion, gasification, or pelletization. Straw can also be used as animal bedding, mulch, soil amendment, and construction material in traditional and sustainable building practices.
- Bagasse: Bagasse is the fibrous residue left after sugarcane stalks are crushed to extract juice for sugar production. It is a lignocellulosic material rich in cellulose and hemicellulose and contains significant energy content. Bagasse is commonly used as a fuel in sugarcane mills to generate process heat and electricity or as a feedstock for ethanol production through fermentation and distillation processes.
- Stover: Stover refers to the leaves, stalks, and cobs of maize (corn) plants left in the field after grain harvest. Maize stover is a valuable source of biomass for bioenergy production, animal feed, soil conservation, and erosion control. It can be used as a feedstock for biogas production through anaerobic digestion, biomass-fired power generation, or cellulosic ethanol production using biochemical or thermochemical conversion processes.
Agricultural residues offer several advantages for bioenergy production compared to dedicated energy crops and woody biomass:
- Abundance: Agricultural residues are produced in large quantities worldwide as by-products of crop cultivation and processing operations, providing a readily available and abundant source of biomass for bioenergy production.
- Low-Cost Feedstock: Agricultural residues are often considered waste or by-products of agricultural activities and may be available at low or no cost to bioenergy producers, reducing feedstock procurement costs and improving the economic viability of bioenergy projects.
- Carbon Neutrality: Agricultural residues are considered carbon-neutral sources of energy because the carbon dioxide emitted during combustion or conversion was originally absorbed from the atmosphere by the plants during photosynthesis, resulting in no net increase in atmospheric CO2 levels.
However, the utilization of agricultural residues for bioenergy production also presents challenges and considerations, including:
- Logistical Challenges: Agricultural residues may be dispersed across large areas, making collection, storage, and transportation logistics challenging and costly, particularly in rural and remote regions with limited infrastructure.
- Seasonal Availability: Agricultural residues are typically available seasonally following crop harvests, resulting in intermittent feedstock availability and requiring storage and handling infrastructure to maintain feedstock quality and availability year-round.
- Soil Health and Nutrient Management: Removing agricultural residues from fields for bioenergy production can impact soil health, fertility, and nutrient cycling, requiring sustainable management practices such as residue retention, cover cropping, and nutrient replenishment to maintain soil productivity and sustainability.
Overall, agricultural residues represent a valuable and sustainable source of biomass for bioenergy production, offering environmental, economic, and social benefits while addressing agricultural waste management challenges and contributing to renewable energy goals and climate change mitigation efforts. By harnessing the potential of agricultural residues, societies can advance towards a more sustainable, resilient, and low-carbon energy future.
Forest Residues:
Forest residues are the organic materials left over from forestry operations and timber processing, including branches, tops, bark, needles, leaves, and sawdust. These residues are generated during various forestry activities such as timber harvesting, thinning, logging, and processing of wood products, and represent a significant source of biomass for bioenergy production, soil improvement, and other applications.
Forest residues are abundant, renewable, and widely available in forested regions worldwide, making them a valuable resource for sustainable bioenergy production. They consist of lignocellulosic materials rich in cellulose, hemicellulose, and lignin, which can be converted into biofuels, heat, power, and other value-added products through various conversion processes.
Common types of forest residues include:
- Logging Residues: Logging residues are generated during timber harvesting operations and include materials such as branches, tops, small-diameter trees, and stumps left in the forest after commercial tree removal. These residues are typically left on the forest floor or piled for disposal but can be collected and utilized for bioenergy production, forest restoration, and erosion control.
- Sawmill Residues: Sawmill residues are generated during the processing of roundwood logs into lumber, boards, and other wood products and include materials such as sawdust, wood chips, shavings, bark, and trimmings. These residues are produced in large quantities in sawmills and can be used for bioenergy production, pulp and paper manufacturing, composite panel production, and other industrial applications.
- Forest Thinnings: Forest thinnings are the removal of smaller, less desirable trees and understory vegetation to improve forest health, growth, and productivity. Thinnings produce residues such as small-diameter trees, branches, and brush, which can be utilized for bioenergy production, forest management, and habitat enhancement purposes.
- Wildfire Fuels: Forest residues are also generated from natural disturbances such as wildfires, windstorms, and insect outbreaks, which produce dead and fallen trees, branches, and woody debris. While wildfire fuels pose challenges for forest management and fire risk mitigation, they can also be utilized for bioenergy production, forest restoration, and wildfire hazard reduction efforts.
Forest residues offer several advantages for bioenergy production compared to other biomass feedstocks:
- Abundance: Forest residues are generated in large quantities from forestry operations and timber processing activities, providing a readily available and abundant source of biomass for bioenergy production.
- Low-Cost Feedstock: Forest residues are often considered waste or by-products of forestry operations and may be available at low or no cost to bioenergy producers, reducing feedstock procurement costs and improving the economic viability of bioenergy projects.
- Carbon Neutrality: Forest residues are considered carbon-neutral sources of energy because the carbon dioxide emitted during combustion or conversion was originally absorbed from the atmosphere by the trees during photosynthesis, resulting in no net increase in atmospheric CO2 levels.
However, the utilization of forest residues for bioenergy production also presents challenges and considerations, including:
- Environmental Impacts: Removing forest residues from ecosystems can impact soil health, nutrient cycling, wildlife habitat, and biodiversity, requiring sustainable management practices to minimize negative environmental impacts and maintain ecosystem integrity.
- Logistical Challenges: Collecting, handling, and transporting forest residues from remote forested areas to bioenergy facilities can present logistical challenges and costs, particularly in rugged terrain and sensitive ecosystems.
- Competing End Uses: Forest residues may have competing end uses such as soil erosion control, wildlife habitat enhancement, and carbon sequestration, requiring careful consideration of trade-offs and priorities in forest management and bioenergy development.
Overall, forest residues represent a valuable and sustainable source of biomass for bioenergy production, offering environmental, economic, and social benefits while supporting forest management goals and objectives. By harnessing the potential of forest residues, societies can advance towards a more sustainable, resilient, and low-carbon energy future while promoting forest health, biodiversity, and ecosystem services.
Carbon Sequestration:
Carbon sequestration is the process by which carbon dioxide (CO2) is removed from the atmosphere and stored in carbon sinks, such as forests, soils, oceans, and geological formations, for an extended period of time. It is a natural and essential component of the global carbon cycle, balancing the carbon flux between the atmosphere, biosphere, and lithosphere, and helping to regulate Earth’s climate and carbon balance.
Carbon sequestration can occur through various natural and artificial processes:
- Biological Sequestration: Biological carbon sequestration involves the uptake and storage of atmospheric CO2 by living organisms, primarily plants, through photosynthesis. Trees, shrubs, grasses, and other vegetation absorb CO2 from the atmosphere during photosynthesis, converting it into biomass (e.g., leaves, stems, roots) and storing carbon in plant tissues and soils. Forests, grasslands, wetlands, and agricultural ecosystems act as important carbon sinks, sequestering large amounts of carbon through vegetation growth and organic matter accumulation.
- Soil Sequestration: Soil carbon sequestration refers to the storage of carbon in soils through the accumulation of organic matter derived from plant residues, root exudates, and microbial biomass. Organic carbon is incorporated into soil organic matter through processes such as decomposition, humification, and microbial activity, where it can persist for decades to centuries. Soil carbon sequestration is influenced by factors such as land use, management practices, soil type, climate, and vegetation cover, with practices such as agroforestry, cover cropping, reduced tillage, and compost application enhancing soil carbon storage and fertility.
- Oceanic Sequestration: Oceanic carbon sequestration involves the transfer of CO2 from the atmosphere to the ocean surface and its subsequent storage in deep ocean waters or marine sediments. The ocean acts as a major carbon sink, absorbing approximately one-third of anthropogenic CO2 emissions from human activities, primarily through physical and chemical processes such as gas exchange, carbonate dissolution, and biological uptake by phytoplankton and marine organisms. However, increasing CO2 levels in the atmosphere can lead to ocean acidification, impacting marine ecosystems and biogeochemical cycles.
- Geological Sequestration: Geological carbon sequestration, also known as carbon capture and storage (CCS), involves capturing CO2 emissions from industrial sources such as power plants, cement kilns, and refineries and injecting them deep underground into geological formations, such as depleted oil and gas reservoirs, saline aquifers, and coal seams. The injected CO2 is stored in porous rock formations and trapped by impermeable caprocks, preventing its release into the atmosphere. CCS technologies offer potential opportunities for mitigating greenhouse gas emissions from large point sources and enhancing carbon storage capacity in geological reservoirs.
Carbon sequestration plays a critical role in mitigating climate change and reducing atmospheric CO2 concentrations by removing carbon from the atmosphere and offsetting anthropogenic emissions. Enhanced carbon sequestration in forests, soils, and oceans can help mitigate the impacts of climate change, including rising temperatures, altered precipitation patterns, sea-level rise, and extreme weather events. However, achieving significant and sustained carbon sequestration requires comprehensive strategies and actions to address deforestation, land degradation, fossil fuel combustion, and other drivers of carbon emissions, while promoting sustainable land management, reforestation, afforestation, and conservation efforts. By harnessing the potential of carbon sequestration, societies can advance towards a more sustainable and resilient future while mitigating the impacts of climate change on ecosystems, economies, and human well-being.
Biochar:
Biochar is a carbon-rich solid material produced from the pyrolysis (thermal decomposition) of biomass in the absence of oxygen (anaerobic conditions). It is a type of charcoal that is rich in carbon and stable over long periods, making it a valuable soil amendment, carbon sequestration tool, and renewable energy source. Biochar has been used for centuries as a soil enhancer in traditional agricultural practices, and its potential for climate change mitigation and sustainable land management is increasingly recognized.
The production of biochar involves heating biomass feedstocks, such as wood chips, crop residues, agricultural waste, or organic matter, at temperatures typically ranging from 350°C to 700°C in a low-oxygen environment (pyrolysis). During pyrolysis, volatile organic compounds and gases are released from the biomass, leaving behind a carbon-rich solid residue, known as biochar. The production process can be optimized to produce biochar with specific properties, such as pore structure, surface area, and chemical composition, tailored to different applications.
Biochar offers several potential benefits and applications:
- Soil Amendment: Biochar is highly porous and has a large surface area, which can improve soil structure, water retention, nutrient retention, and microbial activity. When applied to soil, biochar acts as a long-term carbon sink, sequestering carbon and reducing greenhouse gas emissions. It can also enhance soil fertility, crop productivity, and resilience to drought, erosion, and nutrient leaching, making it a valuable soil amendment for agriculture, forestry, and land restoration.
- Carbon Sequestration: Biochar is a stable form of organic carbon that can persist in soil for hundreds to thousands of years, effectively sequestering carbon and mitigating climate change. By converting biomass into biochar through pyrolysis, carbon that would otherwise be released into the atmosphere as CO2 during decomposition or combustion is stored in a stable form in soil, contributing to carbon sequestration and reducing net greenhouse gas emissions.
- Waste Management: Biochar production can help mitigate the environmental impacts of biomass waste streams, such as agricultural residues, forestry residues, and organic waste. By converting these waste materials into biochar, valuable nutrients and organic matter can be retained and recycled in soil, reducing the need for chemical fertilizers, landfill disposal, and greenhouse gas emissions from biomass decomposition.
- Renewable Energy: Biochar production generates bioenergy in the form of syngas (a mixture of hydrogen, carbon monoxide, and methane) and bio-oil, which can be used as renewable fuels for heat and power generation or as feedstocks for biofuel production. The by-products of biochar production can be utilized to offset fossil fuel consumption and reduce dependence on non-renewable energy sources, contributing to sustainable energy production and climate change mitigation.
- Water Filtration: Biochar’s porous structure and high surface area make it an effective adsorbent for removing contaminants from water, including heavy metals, organic pollutants, and nutrients. Biochar can be used in water filtration systems, wastewater treatment plants, and agricultural drainage systems to improve water quality, reduce pollution, and protect aquatic ecosystems.
Overall, biochar represents a versatile and sustainable solution for addressing multiple environmental challenges, including soil degradation, climate change, waste management, and water pollution. By harnessing the potential of biochar production and utilization, societies can promote sustainable land management practices, enhance soil fertility and resilience, and contribute to global efforts to mitigate climate change and preserve natural resources for future generations.
Biomass Gasification:
Biomass gasification is a thermochemical process that converts biomass feedstocks into synthesis gas (syngas), a mixture of hydrogen, carbon monoxide, methane, and other gases, in the presence of a controlled amount of oxygen and/or steam. It is a versatile and efficient technology for converting solid biomass, such as wood chips, agricultural residues, and energy crops, into a clean and renewable fuel for heat, power, and biofuel production.
The biomass gasification process typically consists of several steps:
- Feedstock Preparation: Biomass feedstocks are first prepared by shredding, chipping, grinding, or drying to reduce particle size, moisture content, and variability. This preparation step ensures uniform feeding and efficient conversion in the gasifier.
- Gasification: Biomass feedstocks are then fed into a gasifier, where they are subjected to high temperatures (typically between 700°C and 1,200°C) and limited oxygen supply (substoichiometric conditions) to undergo thermochemical reactions. The biomass undergoes several simultaneous processes, including pyrolysis (thermal decomposition), oxidation, and reduction, resulting in the production of syngas.
- Syngas Cleanup: The raw syngas produced from the gasifier contains impurities such as tars, particulates, sulfur compounds, and trace contaminants, which must be removed to meet quality specifications for downstream utilization. Syngas cleanup technologies, such as filtration, scrubbing, catalytic conversion, and tar cracking, are employed to remove impurities and improve syngas composition and quality.
- Syngas Conditioning: The cleaned syngas may undergo further conditioning and upgrading to adjust its composition and energy content for specific end uses. This may include adjusting the ratio of hydrogen to carbon monoxide (the H2:CO ratio), removing trace impurities, adjusting gas moisture content, and optimizing syngas heating value for combustion, gasification, or synthesis applications.
- Syngas Utilization: The conditioned syngas can be utilized for various energy applications, including:
- Heat Generation: Syngas can be combusted directly in boilers, furnaces, or gas turbines to produce heat for industrial processes, district heating, or residential heating applications.
- Power Generation: Syngas can be used as a fuel for internal combustion engines, gas turbines, or fuel cells to generate electricity. Combined heat and power (CHP) systems can utilize both the heat and power produced from syngas for maximum efficiency.
- Biofuel Production: Syngas can be converted into liquid fuels such as methanol, ethanol, Fischer-Tropsch diesel, or synthetic natural gas (SNG) through catalytic processes such as Fischer-Tropsch synthesis or methanol synthesis. These biofuels can be used as transportation fuels or blended with conventional fuels to reduce greenhouse gas emissions.
Biomass gasification offers several advantages for bioenergy production compared to conventional combustion technologies:
- Higher Energy Efficiency: Gasification can achieve higher energy conversion efficiencies compared to direct combustion by utilizing waste heat for cogeneration or capturing and utilizing syngas by-products such as hydrogen and carbon monoxide for fuel synthesis.
- Fuel Flexibility: Gasification can utilize a wide range of biomass feedstocks, including wood, agricultural residues, energy crops, and organic waste, allowing for flexibility in feedstock selection based on availability, cost, and sustainability considerations.
- Lower Emissions: Gasification produces syngas with lower emissions of sulfur dioxide, nitrogen oxides, particulate matter, and greenhouse gases compared to conventional combustion, contributing to air quality improvement and climate change mitigation efforts.
However, biomass gasification also presents challenges and considerations, including high capital costs, complex process integration, feedstock variability, syngas cleanup requirements, and technology scale-up issues. Addressing these challenges requires ongoing research, development, and deployment of gasification technologies, as well as supportive policies, incentives, and regulatory frameworks to promote biomass utilization and renewable energy deployment. By harnessing the potential of biomass gasification, societies can advance towards a more sustainable, low-carbon energy future while promoting rural development, economic growth, and environmental stewardship.
Pyrolysis:
Pyrolysis is a thermochemical decomposition process that converts biomass feedstocks into biochar, bio-oil, and syngas in the absence of oxygen (anaerobic conditions). It is a versatile and efficient technology for converting solid biomass, such as wood, agricultural residues, and energy crops, into valuable products for energy, fuels, and chemicals production, while also sequestering carbon and reducing greenhouse gas emissions.
The pyrolysis process typically consists of several stages:
- Feedstock Preparation: Biomass feedstocks are first prepared by shredding, chipping, grinding, or drying to reduce particle size, moisture content, and variability. This preparation step ensures uniform heating and efficient conversion in the pyrolysis reactor.
- Pyrolysis Reactor: The prepared biomass feedstocks are then fed into a pyrolysis reactor, where they are heated to high temperatures (typically between 300°C and 800°C) in the absence of oxygen (anaerobic conditions). The biomass undergoes thermal decomposition reactions, breaking down into volatile organic compounds (bio-oil), non-condensable gases (syngas), and solid carbonaceous residue (biochar).
- Product Collection: The products of pyrolysis are collected and separated for further processing and utilization:
- Biochar: The solid carbonaceous residue, known as biochar, is a stable form of organic carbon that can be used as a soil amendment, carbon sequestration tool, or renewable energy source. Biochar has a high surface area, porosity, and nutrient retention capacity, making it valuable for improving soil fertility, water retention, and crop productivity in agricultural, forestry, and land restoration applications.
- Bio-oil: The liquid fraction of pyrolysis, known as bio-oil or pyrolysis oil, is a complex mixture of organic compounds, including oxygenates, hydrocarbons, and phenolics. Bio-oil can be upgraded and refined into biofuels such as biodiesel, renewable diesel, or gasoline through processes such as hydrodeoxygenation, esterification, or hydrotreating. Bio-oil can also be used as a feedstock for chemical synthesis, heat and power generation, or integrated into refinery processes for petroleum co-processing.
- Syngas: The gaseous fraction of pyrolysis, known as syngas or synthesis gas, is a mixture of hydrogen, carbon monoxide, methane, and other gases. Syngas can be used as a fuel for heat and power generation, chemical synthesis, or conversion into biofuels such as hydrogen, methane, or synthetic natural gas (SNG) through processes such as steam reforming, Fischer-Tropsch synthesis, or methanation.
Pyrolysis offers several advantages for biomass conversion compared to other thermochemical processes:
- Biochar Production: Pyrolysis produces biochar as a valuable co-product, which can improve soil fertility, carbon sequestration, and agricultural sustainability, while also reducing greenhouse gas emissions and mitigating climate change.
- Liquid Biofuel Production: Pyrolysis generates bio-oil as a versatile feedstock for liquid biofuel production, offering potential alternatives to petroleum-based fuels and reducing dependence on fossil fuels.
- Syngas Utilization: Pyrolysis produces syngas as a versatile fuel for heat, power, and chemical production, offering flexibility in energy applications and value-added product synthesis.
However, pyrolysis also presents challenges and considerations, including feedstock variability, product quality and stability, process scalability, energy efficiency, and economic viability. Addressing these challenges requires ongoing research, development, and deployment of pyrolysis technologies, as well as supportive policies, incentives, and market mechanisms to promote biomass utilization and renewable energy deployment. By harnessing the potential of pyrolysis, societies can advance towards a more sustainable, low-carbon energy future while promoting rural development, economic growth, and environmental stewardship.
Anaerobic Digestion:
Anaerobic digestion is a biological process that converts organic materials, such as agricultural residues, food waste, animal manure, and sewage sludge, into biogas and digestate in the absence of oxygen (anaerobic conditions). It is a sustainable and environmentally friendly technology for waste management, renewable energy production, and nutrient recycling, offering multiple benefits for agriculture, wastewater treatment, and resource recovery.
The anaerobic digestion process typically consists of several stages:
- Feedstock Preparation: Organic feedstocks are first prepared by shredding, grinding, or mixing to reduce particle size, increase surface area, and enhance biodegradability. This preparation step facilitates the breakdown of complex organic compounds into simpler compounds by anaerobic microorganisms.
- Digestion Tank: The prepared organic feedstocks are then fed into a sealed digestion tank or reactor, where they are mixed and maintained under anaerobic conditions to facilitate microbial decomposition. Anaerobic microorganisms, primarily bacteria and archaea, break down organic matter through a series of biochemical reactions, producing biogas as a by-product.
- Biogas Production: During anaerobic digestion, organic matter is converted into biogas, a mixture of methane (CH4), carbon dioxide (CO2), and trace gases such as hydrogen sulfide (H2S) and ammonia (NH3). Methane is the primary component of biogas and can be used as a renewable fuel for heat and power generation, transportation, and industrial applications.
- Digestate Production: The residual material remaining after anaerobic digestion, known as digestate, is rich in nutrients such as nitrogen, phosphorus, potassium, and organic matter. Digestate can be separated into solid and liquid fractions and used as a nutrient-rich fertilizer or soil amendment in agriculture, horticulture, and landscaping, helping to improve soil fertility and crop productivity.
Anaerobic digestion offers several advantages for organic waste management and renewable energy production:
- Waste Reduction: Anaerobic digestion reduces the volume and biodegradability of organic waste materials, diverting them from landfills and reducing greenhouse gas emissions from decomposition. It helps to minimize odors, pathogens, and environmental pollution associated with organic waste disposal while recovering valuable energy and nutrients from waste streams.
- Biogas Production: Anaerobic digestion produces biogas as a renewable and versatile energy source, which can be used for heat and power generation, combined heat and power (CHP) systems, biogas upgrading to biomethane for injection into natural gas pipelines, or transportation fuels such as compressed natural gas (CNG) or liquefied natural gas (LNG).
- Nutrient Recycling: Anaerobic digestion recovers nutrients such as nitrogen, phosphorus, and potassium from organic waste streams, converting them into a valuable fertilizer (digestate) for agricultural use. Digestate provides organic matter, micronutrients, and soil conditioning properties, promoting soil health, fertility, and sustainable crop production practices.
- Greenhouse Gas Mitigation: Anaerobic digestion reduces methane emissions from organic waste decomposition in landfills by capturing and utilizing biogas for energy production. Methane, a potent greenhouse gas, is converted into carbon dioxide during combustion or utilization, reducing its impact on global warming and climate change.
However, anaerobic digestion also presents challenges and considerations, including:
- Feedstock Contamination: Anaerobic digestion performance can be affected by feedstock contamination, such as inert materials, plastics, heavy metals, and toxic compounds, which may inhibit microbial activity, cause process upsets, and reduce biogas yield and quality.
- Process Optimization: Anaerobic digestion processes require careful monitoring and optimization of operational parameters such as temperature, pH, retention time, mixing, and substrate loading rates to maximize biogas production, digestate quality, and process stability.
- Economic Viability: Anaerobic digestion projects require upfront investment in infrastructure, equipment, and operational costs, which may be challenging to recover through biogas and digestate sales, energy revenues, or carbon credits, particularly in regions with low energy prices or limited market opportunities.
Addressing these challenges requires technological innovation, process optimization, supportive policies, financial incentives, and stakeholder engagement to promote anaerobic digestion as a sustainable waste management and renewable energy solution. By harnessing the potential of anaerobic digestion, societies can advance towards a circular economy, resource efficiency, and climate resilience while mitigating environmental impacts and promoting sustainable development.
Syngas:
Syngas, short for synthesis gas, is a mixture of hydrogen (H2), carbon monoxide (CO), and sometimes carbon dioxide (CO2) and methane (CH4). It is produced through various thermochemical processes such as gasification, pyrolysis, and steam reforming of hydrocarbons or biomass in the presence of controlled amounts of oxygen, steam, or both.
Syngas serves as a versatile intermediate for the production of a wide range of fuels, chemicals, and energy products. Its composition and properties can vary depending on the feedstock used, process conditions, and gasification technology employed. The most common components of syngas and their roles are:
- Hydrogen (H2): Hydrogen is a valuable fuel and chemical feedstock used in various industrial processes, fuel cells, and hydrogenation reactions. It has a high energy content per unit mass and can be used directly as a clean fuel for heat and power generation or as a feedstock for ammonia synthesis, methanol production, hydrogenation of organic compounds, and hydrogen fuel cells.
- Carbon Monoxide (CO): Carbon monoxide is a versatile building block for chemical synthesis and catalytic reactions. It is used in processes such as Fischer-Tropsch synthesis for producing synthetic fuels and chemicals, methanol synthesis, hydroformylation, and carbonylation reactions. CO can also be combusted directly in gas turbines or internal combustion engines for power generation or used as a reducing agent in metallurgical processes.
- Carbon Dioxide (CO2): Carbon dioxide is a greenhouse gas emitted from various industrial processes, combustion of fossil fuels, and biomass utilization. In syngas production, CO2 can be present as a by-product or introduced as a reactant for adjusting syngas composition or promoting certain chemical reactions. CO2 can be captured and sequestered to reduce greenhouse gas emissions or utilized in processes such as methanol synthesis, carbonation reactions, and algae cultivation for biofuels production.
- Methane (CH4): Methane is a major component of natural gas and biogas and serves as a valuable fuel for heat and power generation, residential and commercial heating, industrial processes, and transportation. It can be produced from syngas through methanation reactions or introduced as a contaminant from biomass or coal gasification processes. Methane can also be upgraded to higher-value products such as hydrogen or synthetic natural gas (SNG) through steam reforming or gasification processes.
Syngas can be produced from a variety of feedstocks, including natural gas, coal, biomass, municipal solid waste, and industrial by-products. It offers several advantages as a renewable and versatile energy source:
- Fuel Flexibility: Syngas can be produced from a wide range of feedstocks, providing flexibility in feedstock selection based on availability, cost, and sustainability considerations. Biomass-derived syngas offers carbon neutrality and environmental benefits compared to fossil-based syngas.
- Energy Efficiency: Syngas production processes, such as gasification and pyrolysis, can achieve high energy conversion efficiencies compared to conventional combustion technologies. Combined heat and power (CHP) systems can utilize syngas for simultaneous heat and power generation, improving overall energy efficiency and resource utilization.
- Chemical Synthesis: Syngas serves as a versatile feedstock for chemical synthesis, enabling the production of a wide range of fuels, chemicals, and materials through catalytic processes such as Fischer-Tropsch synthesis, methanol synthesis, ammonia synthesis, and hydroformylation. These chemicals can be used as transportation fuels, industrial feedstocks, plastics, pharmaceuticals, and specialty chemicals.
However, syngas utilization also presents challenges and considerations, including:
- Gas Cleanup: Syngas produced from biomass or coal gasification processes may contain impurities such as tars, particulates, sulfur compounds, and trace contaminants, which must be removed to meet quality specifications for downstream utilization. Syngas cleanup technologies, such as filtration, scrubbing, catalytic conversion, and tar cracking, are employed to remove impurities and improve syngas composition and quality.
- Technology Integration: Syngas utilization requires integration with downstream processes such as gas cleanup, conditioning, upgrading, and utilization in power generation, chemical synthesis, or fuel production applications. Process integration and optimization are critical for maximizing syngas yield, quality, and value-added product synthesis.
- Economic Viability: Syngas utilization projects require upfront investment in infrastructure, equipment, and operational costs, which may be challenging to recover through product sales, energy revenues, or carbon credits, particularly in regions with low energy prices or limited market opportunities. Economic incentives, supportive policies, and market mechanisms are needed to promote syngas utilization and investment in renewable energy and low-carbon technologies.
Overall, syngas represents a valuable and versatile energy source for addressing energy security, environmental sustainability, and climate change mitigation challenges. By harnessing the potential of syngas production and utilization, societies can advance towards a more sustainable, low-carbon energy future while promoting economic development, innovation, and energy resilience.
Gasification Plant:
A gasification plant is an industrial facility that converts carbonaceous feedstocks, such as coal, biomass, petroleum coke, or municipal solid waste, into synthesis gas (syngas) through the process of gasification. Gasification plants play a critical role in the production of clean and renewable energy, fuels, chemicals, and materials by converting solid or liquid feedstocks into a versatile gaseous fuel suitable for various applications.
The operation of a gasification plant typically involves the following key steps:
- Feedstock Handling and Preparation: Carbonaceous feedstocks are received, stored, and prepared for gasification at the plant site. Depending on the feedstock type and characteristics, preparation may include shredding, grinding, drying, screening, and size reduction to optimize feedstock properties and facilitate gasification.
- Gasification Reactor: The prepared feedstock is fed into a gasification reactor, where it undergoes thermochemical conversion at elevated temperatures (typically between 700°C and 1,500°C) and controlled oxygen supply (substoichiometric conditions). Gasification reactors may operate under various configurations, such as fixed-bed, fluidized bed, entrained flow, or plasma arc, depending on the feedstock properties, process requirements, and desired product outputs.
- Syngas Production: In the gasification reactor, the feedstock undergoes several simultaneous thermochemical reactions, including pyrolysis (thermal decomposition), oxidation, reduction, and reforming, resulting in the production of syngas. Syngas is composed primarily of hydrogen (H2), carbon monoxide (CO), carbon dioxide (CO2), methane (CH4), and trace contaminants such as hydrogen sulfide (H2S), ammonia (NH3), and tar compounds.
- Syngas Cleanup and Conditioning: The raw syngas produced from the gasification reactor contains impurities such as tars, particulates, sulfur compounds, and trace contaminants, which must be removed to meet quality specifications for downstream utilization. Syngas cleanup and conditioning technologies, such as filtration, scrubbing, catalytic conversion, and tar cracking, are employed to remove impurities and improve syngas composition, purity, and energy content.
- Syngas Utilization: The cleaned and conditioned syngas can be utilized for various energy, fuels, chemicals, and materials production applications, including:
- Heat and Power Generation: Syngas can be combusted directly in boilers, gas turbines, or internal combustion engines to produce heat and power for industrial processes, district heating, or electricity generation.
- Biofuel Production: Syngas can be converted into liquid fuels such as methanol, ethanol, Fischer-Tropsch diesel, or synthetic natural gas (SNG) through catalytic processes such as Fischer-Tropsch synthesis, methanol synthesis, or hydrocarbon reforming. These biofuels can be used as transportation fuels or blended with conventional fuels to reduce greenhouse gas emissions.
- Chemical Synthesis: Syngas serves as a versatile feedstock for chemical synthesis, enabling the production of a wide range of chemicals, including ammonia, hydrogen, methanol, dimethyl ether, olefins, and synthetic fuels. These chemicals are used in various industrial sectors, including agriculture, petrochemicals, pharmaceuticals, and materials manufacturing.
Gasification plants offer several advantages for energy and chemical production compared to conventional combustion technologies:
- Fuel Flexibility: Gasification plants can utilize a wide range of feedstocks, including coal, biomass, petroleum coke, and municipal solid waste, providing flexibility in feedstock selection based on availability, cost, and sustainability considerations.
- Efficiency: Gasification processes can achieve higher energy conversion efficiencies compared to conventional combustion technologies by utilizing waste heat for cogeneration or capturing and utilizing syngas by-products such as hydrogen and carbon monoxide for fuel synthesis.
- Emissions Reduction: Gasification plants produce syngas with lower emissions of sulfur dioxide, nitrogen oxides, particulate matter, and greenhouse gases compared to conventional combustion technologies, contributing to air quality improvement and climate change mitigation efforts.
However, gasification plants also present challenges and considerations, including high capital costs, complex process integration, feedstock variability, syngas cleanup requirements, and technology scale-up issues. Addressing these challenges requires ongoing research, development, and deployment of gasification technologies, as well as supportive policies, incentives, and regulatory frameworks to promote biomass utilization and renewable energy deployment.
Overall, gasification plants play a critical role in advancing towards a more sustainable, low-carbon energy future by converting carbonaceous feedstocks into clean and renewable energy, fuels, chemicals, and materials while reducing environmental impacts and promoting economic development and energy security.
Biomass Cogeneration:
Biomass cogeneration, also known as biomass combined heat and power (CHP), is a sustainable energy generation process that simultaneously produces heat and electricity from biomass feedstocks such as wood, agricultural residues, energy crops, and organic waste. It is a highly efficient and environmentally friendly technology that utilizes the energy content of biomass to generate both thermal and electrical energy, maximizing resource utilization and minimizing greenhouse gas emissions.
The biomass cogeneration process typically involves the following key components:
- Biomass Fuel Handling: Biomass feedstocks are received, stored, and prepared for combustion or gasification at the cogeneration plant site. Feedstock handling systems may include shredders, grinders, conveyors, and storage silos to optimize feedstock properties and facilitate biomass combustion or gasification.
- Combustion or Gasification System: Biomass feedstocks are combusted in a boiler or gasified in a gasifier to generate thermal energy in the form of steam or hot gases. Combustion systems burn biomass in the presence of oxygen to produce heat, while gasification systems convert biomass into syngas (a mixture of hydrogen, carbon monoxide, and methane) in the absence of oxygen.
- Heat Recovery: The thermal energy produced from biomass combustion or gasification is used to generate steam, hot water, or thermal oil, depending on the process requirements and system configuration. Heat exchangers, boilers, and heat recovery steam generators (HRSGs) recover heat from flue gases or syngas and transfer it to a heat transfer fluid for further utilization.
- Steam Turbine or Engine: The high-pressure steam or hot gas produced from biomass combustion or gasification drives a steam turbine, reciprocating engine, or other prime mover to generate electricity. The mechanical energy of the turbine or engine is converted into electrical energy through a generator, which produces electricity for on-site use or export to the grid.
- Combined Heat and Power (CHP) Generation: Biomass cogeneration systems produce both heat and electricity simultaneously, allowing for efficient utilization of energy resources and maximizing overall energy efficiency. The heat generated from biomass combustion or gasification is used for industrial processes, space heating, district heating, or hot water production, while the electricity generated is used for on-site power supply or exported to the grid.
- Energy Distribution and Utilization: The heat and electricity produced from biomass cogeneration are distributed and utilized for various applications, including industrial processes, residential heating, commercial buildings, agricultural operations, and district heating networks. Cogeneration systems can provide reliable and decentralized energy supply, improve energy security, and reduce dependence on fossil fuels.
Biomass cogeneration offers several advantages over conventional energy generation technologies:
- High Efficiency: Biomass cogeneration systems can achieve high overall energy conversion efficiencies (typically 70-90%) by utilizing waste heat for cogeneration, compared to separate generation of heat and electricity. This results in significant energy savings and greenhouse gas emissions reductions.
- Renewable Energy: Biomass is a renewable and sustainable energy source derived from organic materials that can be replenished over time through natural processes such as photosynthesis. Biomass cogeneration helps reduce dependence on fossil fuels and mitigate climate change by displacing greenhouse gas emissions from fossil-based energy sources.
- Resource Utilization: Biomass cogeneration utilizes the energy content of biomass feedstocks efficiently by generating both heat and electricity, maximizing resource utilization and minimizing waste. Biomass residues and by-products from agricultural, forestry, and industrial processes can be utilized as fuel, reducing waste disposal and environmental pollution.
- Local Economic Development: Biomass cogeneration projects can stimulate local economic development by creating jobs, supporting rural economies, and providing opportunities for biomass suppliers, equipment manufacturers, and service providers. Cogeneration plants can also contribute to energy independence and resilience by diversifying energy sources and reducing reliance on imported fuels.
However, biomass cogeneration also presents challenges and considerations, including biomass availability and logistics, feedstock quality and variability, technology integration and optimization, environmental permitting and regulatory compliance, and economic viability. Addressing these challenges requires comprehensive planning, stakeholder engagement, technological innovation, supportive policies, and financial incentives to promote biomass utilization and renewable energy deployment.
Overall, biomass cogeneration plays a vital role in advancing towards a more sustainable, low-carbon energy future by harnessing the potential of biomass resources to generate clean and renewable heat and power, while supporting economic development, energy security, and environmental stewardship.
Biomass Pyrolysis:
Biomass pyrolysis is a thermochemical conversion process that decomposes biomass feedstocks into biochar, bio-oil, and syngas in the absence of oxygen (anaerobic conditions). It is a versatile and efficient technology for converting solid biomass, such as wood, agricultural residues, and energy crops, into valuable products for energy, fuels, chemicals, and materials production, while also sequestering carbon and reducing greenhouse gas emissions.
The biomass pyrolysis process involves several stages:
- Feedstock Preparation: Biomass feedstocks are first prepared by shredding, chipping, grinding, or drying to reduce particle size, moisture content, and variability. This preparation step ensures uniform heating and efficient conversion in the pyrolysis reactor.
- Pyrolysis Reactor: The prepared biomass feedstocks are then fed into a pyrolysis reactor, where they are heated to high temperatures (typically between 300°C and 800°C) in the absence of oxygen. The biomass undergoes thermal decomposition reactions, breaking down into volatile organic compounds (bio-oil), non-condensable gases (syngas), and solid carbonaceous residue (biochar).
- Product Collection: The products of pyrolysis are collected and separated for further processing and utilization:
- Biochar: The solid carbonaceous residue, known as biochar, is a stable form of organic carbon that can be used as a soil amendment, carbon sequestration tool, or renewable energy source. Biochar has a high surface area, porosity, and nutrient retention capacity, making it valuable for improving soil fertility, water retention, and crop productivity in agricultural, forestry, and land restoration applications.
- Bio-oil: The liquid fraction of pyrolysis, known as bio-oil or pyrolysis oil, is a complex mixture of organic compounds, including oxygenates, hydrocarbons, and phenolics. Bio-oil can be upgraded and refined into biofuels such as biodiesel, renewable diesel, or gasoline through processes such as hydrodeoxygenation, esterification, or hydrotreating. Bio-oil can also be used as a feedstock for chemical synthesis, heat and power generation, or integrated into refinery processes for petroleum co-processing.
- Syngas: The gaseous fraction of pyrolysis, known as syngas or synthesis gas, is a mixture of hydrogen, carbon monoxide, methane, and other gases. Syngas can be used as a fuel for heat and power generation, chemical synthesis, or conversion into biofuels such as hydrogen, methane, or synthetic natural gas (SNG) through processes such as steam reforming, Fischer-Tropsch synthesis, or methanation.
Biomass pyrolysis offers several advantages for biomass conversion and utilization:
- Biochar Production: Pyrolysis produces biochar as a valuable co-product, which can improve soil fertility, carbon sequestration, and agricultural sustainability, while also reducing greenhouse gas emissions and mitigating climate change.
- Liquid Biofuel Production: Pyrolysis generates bio-oil as a versatile feedstock for liquid biofuel production, offering potential alternatives to petroleum-based fuels and reducing dependence on fossil fuels.
- Syngas Utilization: Pyrolysis produces syngas as a versatile fuel for heat, power, and chemical production, offering flexibility in energy applications and value-added product synthesis.
However, biomass pyrolysis also presents challenges and considerations, including feedstock variability, product quality and stability, process scalability, energy efficiency, and economic viability. Addressing these challenges requires ongoing research, development, and deployment of pyrolysis technologies, as well as supportive policies, incentives, and market mechanisms to promote biomass utilization and renewable energy deployment.
Overall, biomass pyrolysis plays a critical role in advancing towards a more sustainable, low-carbon energy future by converting biomass resources into valuable products for energy, fuels, chemicals, and materials production, while also promoting environmental stewardship, economic development, and energy security.
Renewable Energy:
Renewable energy refers to energy derived from naturally replenishing resources that are virtually inexhaustible over human time scales, such as sunlight, wind, water, biomass, and geothermal heat. Unlike fossil fuels, which are finite and non-renewable, renewable energy sources are abundant, clean, and environmentally sustainable, making them key components of efforts to mitigate climate change, enhance energy security, and promote sustainable development.
The major sources of renewable energy include:
- Solar Energy: Solar energy is derived from the radiation of sunlight, which can be captured and converted into electricity using photovoltaic (PV) solar panels or concentrated solar power (CSP) systems. Solar energy is abundant, widely distributed, and inexhaustible, offering significant potential for decentralized electricity generation, off-grid applications, and renewable energy integration into buildings, infrastructure, and transportation.
- Wind Energy: Wind energy is generated by harnessing the kinetic energy of wind through wind turbines, which convert wind power into electricity. Wind energy is clean, abundant, and cost-effective, with onshore and offshore wind farms providing a growing share of global electricity generation. Wind energy contributes to energy diversification, grid stability, and carbon emissions reduction, displacing fossil fuels in power generation and promoting energy transition.
- Hydropower: Hydropower is generated by capturing the energy of flowing water through dams, turbines, and hydroelectric power plants. Hydropower is one of the oldest and most widely used renewable energy sources, providing reliable and dispatchable electricity generation, energy storage, flood control, and water management services. Large-scale hydroelectric projects and small-scale run-of-river installations contribute to renewable energy deployment and sustainable water resources management.
- Biomass Energy: Biomass energy is derived from organic materials such as wood, agricultural residues, energy crops, and organic waste, which can be burned, gasified, or fermented to produce heat, electricity, biofuels, and bioproducts. Biomass energy is versatile, flexible, and carbon-neutral when sustainably managed, offering opportunities for decentralized energy production, waste valorization, and rural development.
- Geothermal Energy: Geothermal energy is produced by harnessing the heat stored beneath the Earth’s surface in hot water reservoirs, steam fields, and geothermal wells. Geothermal power plants use geothermal heat to generate electricity through steam turbines, while direct-use applications utilize geothermal heat for district heating, greenhouse heating, industrial processes, and spa resorts. Geothermal energy is reliable, baseload-compatible, and environmentally benign, providing sustainable heating and cooling solutions in geologically active regions.
- Ocean Energy: Ocean energy encompasses various technologies for capturing energy from the ocean’s waves, tides, currents, and thermal gradients. Ocean energy resources, including wave energy, tidal energy, ocean current energy, and ocean thermal energy conversion (OTEC), offer significant potential for clean, renewable electricity generation, particularly in coastal regions and island nations. Ocean energy technologies are still in the early stages of commercial development but hold promise for contributing to renewable energy portfolios and climate change mitigation strategies.
Renewable energy offers numerous benefits compared to fossil fuels, including:
- Climate Change Mitigation: Renewable energy sources produce little to no greenhouse gas emissions during electricity generation, reducing carbon dioxide and other pollutants that contribute to climate change and air pollution. Transitioning to renewable energy is essential for achieving global climate targets and limiting global warming to well below 2°C.
- Energy Security: Renewable energy sources are domestically available and diversified, reducing dependence on imported fossil fuels and enhancing energy security and resilience. Investing in renewable energy technologies reduces exposure to volatile fuel prices, geopolitical risks, and supply disruptions, promoting energy independence and sovereignty.
- Environmental Sustainability: Renewable energy development minimizes habitat destruction, land degradation, water pollution, and ecosystem disruption associated with fossil fuel extraction, transportation, and combustion. Renewable energy projects can be designed and managed to minimize environmental impacts, preserve biodiversity, and enhance ecosystem services, supporting sustainable development goals.
- Economic Growth: Renewable energy deployment stimulates economic growth, job creation, and investment opportunities in manufacturing, construction, installation, operation, and maintenance of renewable energy infrastructure. Renewable energy industries generate local employment, tax revenues, and economic development benefits, particularly in rural and underserved communities.
Despite these benefits, renewable energy deployment faces challenges and barriers, including intermittency and variability of renewable resources, grid integration and energy storage requirements, technology costs and performance, policy and regulatory barriers, financing constraints, and public acceptance and awareness. Addressing these challenges requires coordinated efforts from governments, industry stakeholders, academia, and civil society to develop and deploy renewable energy technologies, improve energy efficiency, and accelerate the transition to a sustainable, low-carbon energy future.
Overall, renewable energy plays a pivotal role in advancing towards a more sustainable, resilient, and equitable energy system, supporting global efforts to address climate change, promote economic development, and ensure energy access for all. By harnessing the power of renewable energy, societies can build a cleaner, healthier, and more prosperous future for generations to come.
Gasification
Gasification is a thermochemical process that converts carbonaceous materials, such as biomass, coal, or waste, into syngas (synthesis gas) in the presence of controlled amounts of oxygen and/or steam. This process occurs at elevated temperatures (typically between 700°C and 1,500°C) and in the absence of complete combustion, resulting in the partial oxidation of the feedstock and the production of a mixture of carbon monoxide (CO), hydrogen (H2), carbon dioxide (CO2), methane (CH4), and other trace gases.
Gasification technology offers several advantages over conventional combustion processes, including higher energy efficiency, lower emissions, and greater flexibility in feedstock utilization. By controlling the gasification conditions, such as temperature, pressure, residence time, and gas composition, it is possible to tailor the syngas composition to meet specific requirements for various applications, including heat and power generation, chemical synthesis, and transportation fuels production.
The gasification process typically involves several key steps:
- Feedstock Preparation: Carbonaceous feedstocks, such as biomass, coal, or waste, are prepared by shredding, drying, and sizing to optimize their physical and chemical properties. This preparation step enhances the reactivity and conversion efficiency of the feedstock during gasification.
- Gasification Reactor: The prepared feedstock is fed into a gasification reactor, where it undergoes thermochemical conversion at high temperatures and controlled oxygen supply. Gasification reactors can operate under various configurations, including fixed-bed, fluidized bed, entrained flow, or plasma arc reactors, depending on the feedstock properties, process requirements, and desired syngas composition.
- Chemical Reactions: In the gasification reactor, the feedstock reacts with oxygen and/or steam to produce syngas through several simultaneous thermochemical reactions, including pyrolysis, oxidation, reduction, and reforming. These reactions break down the complex organic compounds in the feedstock into simpler molecules, releasing energy and forming syngas as the primary product.
- Syngas Cleanup: The raw syngas produced from the gasification reactor contains impurities such as tars, particulates, sulfur compounds, and trace contaminants, which must be removed to meet quality specifications for downstream utilization. Syngas cleanup technologies, such as filtration, scrubbing, catalytic conversion, and tar cracking, are employed to remove impurities and improve syngas composition, purity, and energy content.
- Syngas Utilization: The cleaned and conditioned syngas can be utilized for various energy, fuels, chemicals, and materials production applications, including:
- Heat and Power Generation: Syngas can be combusted directly in boilers, gas turbines, or internal combustion engines to produce heat and power for industrial processes, district heating, or electricity generation.
- Chemical Synthesis: Syngas serves as a versatile feedstock for chemical synthesis, enabling the production of a wide range of chemicals, including ammonia, methanol, hydrogen, and synthetic fuels. These chemicals are used in various industrial sectors, including agriculture, petrochemicals, pharmaceuticals, and materials manufacturing.
- Biofuels Production: Syngas can be converted into liquid biofuels such as ethanol, biodiesel, or Fischer-Tropsch diesel through catalytic processes such as Fischer-Tropsch synthesis or alcohol synthesis. These biofuels can be used as transportation fuels or blended with conventional fuels to reduce greenhouse gas emissions and promote energy independence.
Gasification technology offers several benefits, including:
- Energy Efficiency: Gasification processes can achieve high energy conversion efficiencies compared to conventional combustion technologies by utilizing waste heat for cogeneration or capturing and utilizing syngas by-products for fuel synthesis.
- Fuel Flexibility: Gasification plants can utilize a wide range of feedstocks, including biomass, coal, petroleum coke, and municipal solid waste, providing flexibility in feedstock selection based on availability, cost, and sustainability considerations.
- Emissions Reduction: Gasification plants produce syngas with lower emissions of sulfur dioxide, nitrogen oxides, particulate matter, and greenhouse gases compared to conventional combustion technologies, contributing to air quality improvement and climate change mitigation efforts.
However, gasification technology also presents challenges and considerations, including feedstock variability, syngas cleanup requirements, technology integration, operational complexity, and economic viability. Addressing these challenges requires ongoing research, development, and deployment of gasification technologies, as well as supportive policies, incentives, and market mechanisms to promote renewable energy deployment and low-carbon technologies.
Thermochemical Conversion:
Thermochemical conversion is a process that involves the transformation of biomass or other carbonaceous materials into energy products, such as heat, electricity, fuels, and chemicals, through the application of heat and chemical reactions. Unlike biochemical conversion, which relies on biological processes such as fermentation or digestion, thermochemical conversion uses heat and chemical catalysts to break down organic matter and produce useful energy carriers.
The thermochemical conversion of biomass typically occurs through several distinct processes, each characterized by specific reaction pathways and operating conditions:
- Pyrolysis: Pyrolysis is a thermal decomposition process that occurs in the absence of oxygen (anaerobic conditions) at temperatures typically ranging from 300°C to 800°C. During pyrolysis, biomass feedstocks are heated rapidly, causing the release of volatile organic compounds, tar, and char. The primary products of pyrolysis are biochar (solid carbonaceous residue), bio-oil (liquid fraction), and syngas (gaseous fraction). Pyrolysis is used to produce biochar for soil amendment, bio-oil for biofuels production, and syngas for heat and power generation.
- Gasification: Gasification is a thermochemical process that converts biomass or other carbonaceous materials into syngas (synthesis gas) through partial oxidation in the presence of controlled amounts of oxygen and/or steam. Gasification occurs at elevated temperatures (typically between 700°C and 1,500°C) and in the absence of complete combustion, resulting in the production of a mixture of carbon monoxide (CO), hydrogen (H2), carbon dioxide (CO2), methane (CH4), and other trace gases. Syngas produced from gasification can be used for heat and power generation, chemical synthesis, or conversion into biofuels such as hydrogen, methane, or synthetic natural gas (SNG).
- Combustion: Combustion is a chemical reaction between a fuel and an oxidizing agent (typically oxygen) that produces heat, light, and combustion products, such as carbon dioxide (CO2), water vapor (H2O), and trace pollutants. Biomass combustion involves the burning of biomass feedstocks in the presence of oxygen to release energy in the form of heat, which can be used for space heating, water heating, steam generation, or electricity production. Combustion technologies include stoker boilers, fluidized bed boilers, and biomass power plants, which combust biomass feedstocks to produce heat and power.
- Torrefaction: Torrefaction is a mild pyrolysis process that involves heating biomass feedstocks in the absence of oxygen at temperatures typically between 200°C and 300°C. During torrefaction, biomass undergoes partial decomposition and removal of volatile organic compounds, resulting in the production of a dry, energy-dense solid called torrefied biomass or bio-coal. Torrefied biomass has improved handling properties, higher energy density, and reduced moisture content compared to raw biomass, making it suitable for combustion, gasification, or pelletization for energy production.
Thermochemical conversion technologies offer several advantages for biomass utilization and energy production:
- Resource Efficiency: Thermochemical conversion processes can utilize a wide range of biomass feedstocks, including woody biomass, agricultural residues, energy crops, and organic waste, maximizing resource utilization and reducing waste disposal.
- Energy Recovery: Thermochemical conversion technologies recover energy from biomass feedstocks in the form of heat, electricity, fuels, and chemicals, displacing fossil fuels and reducing greenhouse gas emissions associated with energy production.
- Product Flexibility: Thermochemical conversion processes can produce a variety of energy products, including biochar, bio-oil, syngas, torrefied biomass, and biofuels, which can be tailored to meet specific market demands and applications.
- Carbon Sequestration: Thermochemical conversion processes such as pyrolysis and torrefaction can produce biochar, a stable form of organic carbon that can be sequestered in soils to enhance soil fertility, carbon storage, and climate change mitigation.
However, thermochemical conversion technologies also present challenges and considerations, including feedstock variability, process efficiency, product quality, technology scale-up, and economic viability. Addressing these challenges requires ongoing research, development, and deployment of thermochemical conversion technologies, as well as supportive policies, incentives, and market mechanisms to promote biomass utilization and renewable energy deployment.
Overall, thermochemical conversion plays a crucial role in advancing towards a more sustainable, low-carbon energy future by converting biomass resources into valuable energy products, while also promoting environmental stewardship, economic development, and energy security.
Biomass Feedstock:
Biomass feedstock refers to organic materials derived from plants, animals, or microorganisms that are used as raw materials for bioenergy production, biofuel manufacturing, and other bioproducts. Biomass feedstocks are renewable, carbon-neutral, and widely available resources that can be sustainably managed and harvested to produce energy and other valuable products while mitigating environmental impacts and promoting rural development.
There are various types of biomass feedstocks, each with its unique characteristics, properties, and applications:
- Woody Biomass: Woody biomass includes trees, shrubs, branches, and woody residues from forestry, logging, and land clearing operations. Woody biomass feedstocks can be harvested from forests, agroforestry systems, or dedicated energy plantations and used for heat and power generation, wood pellets production, pulp and paper manufacturing, and biofuel production.
- Agricultural Residues: Agricultural residues are by-products of crop cultivation, harvesting, and processing operations, including straw, stalks, husks, cobs, shells, and bagasse. Agricultural residues are abundant, locally available feedstocks that can be used for biomass combustion, biogas production, biochar production, or ethanol production, providing additional revenue streams for farmers and reducing agricultural waste.
- Energy Crops: Energy crops are dedicated crops grown specifically for bioenergy production, such as switchgrass, miscanthus, willow, poplar, and sugarcane. Energy crops are fast-growing, high-yielding feedstocks that can be cultivated on marginal lands, degraded soils, or underutilized agricultural land, reducing competition with food crops and enhancing biodiversity and ecosystem services.
- Organic Waste: Organic waste includes biodegradable materials from municipal, industrial, and agricultural sources, such as food waste, yard waste, animal manure, and sewage sludge. Organic waste feedstocks can be collected, processed, and converted into biogas through anaerobic digestion, producing renewable natural gas (RNG) for heat, power, or transportation fuel applications, and reducing methane emissions from landfills and wastewater treatment plants.
- Algae: Algae are photosynthetic microorganisms that can convert sunlight, carbon dioxide, and nutrients into biomass through photosynthesis. Algae feedstocks can be cultivated in ponds, tanks, or photobioreactors and harvested for biofuel production, including biodiesel, bioethanol, biogas, or biohydrogen, offering potential alternatives to land-based biomass feedstocks and reducing pressure on arable land and freshwater resources.
Biomass feedstocks offer several advantages for bioenergy production and sustainable development:
- Renewability: Biomass feedstocks are renewable resources derived from solar energy and carbon dioxide through photosynthesis, replenished over time through natural processes and sustainable management practices.
- Carbon Neutrality: Biomass feedstocks are considered carbon-neutral sources of energy because the carbon dioxide emitted during combustion or decomposition is offset by the carbon dioxide absorbed during plant growth, resulting in no net increase in atmospheric carbon dioxide levels.
- Resource Diversity: Biomass feedstocks are diverse and abundant resources that can be sourced from a variety of sources, including forestry, agriculture, waste streams, and algae cultivation, providing flexibility in feedstock selection and supply chain resilience.
- Rural Development: Biomass feedstock production and utilization can stimulate rural economies, create jobs, and provide additional income opportunities for farmers, foresters, and rural communities, contributing to economic development, poverty reduction, and social equity.
However, biomass feedstock utilization also presents challenges and considerations, including feedstock availability and logistics, supply chain management, land use competition, water consumption, nutrient cycling, biodiversity impacts, and socio-economic implications. Addressing these challenges requires integrated approaches that balance environmental, social, and economic objectives and promote sustainable biomass production, utilization, and management practices.
Overall, biomass feedstocks play a critical role in advancing towards a more sustainable, low-carbon energy future by providing renewable resources for bioenergy production, biofuel manufacturing, and other bioproducts, while also promoting environmental stewardship, economic development, and energy security.
Pyrolysis:
Pyrolysis is a thermochemical decomposition process that converts organic materials, such as biomass, into biochar, bio-oil, and syngas in the absence of oxygen (anaerobic conditions). This process involves heating the biomass feedstock at elevated temperatures (typically between 300°C and 800°C) in a controlled environment, leading to the breakdown of complex organic compounds into simpler molecules and the release of volatile gases.
The pyrolysis process can be divided into several stages:
- Drying Stage: Initially, the biomass feedstock undergoes drying to remove moisture content, typically through low-temperature heating. This stage prepares the biomass for subsequent pyrolysis reactions by reducing the energy required for heating and improving the efficiency of the process.
- Pyrolysis Stage: As the temperature increases, the biomass undergoes thermal decomposition, leading to the release of volatile organic compounds, water vapor, and char. The pyrolysis reactions involve several simultaneous processes, including depolymerization, dehydration, decarboxylation, and devolatilization, which break down the biomass into biochar, bio-oil, and syngas.
- Biochar: Biochar is the solid carbonaceous residue produced from pyrolysis, consisting of carbon-rich material with high surface area, porosity, and stability. Biochar is used as a soil amendment to improve soil fertility, water retention, and nutrient cycling, enhance crop productivity, and sequester carbon in agricultural soils, mitigating climate change and enhancing ecosystem resilience.
- Bio-oil: Bio-oil, also known as pyrolysis oil or bio-crude, is the liquid fraction of pyrolysis consisting of a complex mixture of oxygenated hydrocarbons, phenolics, and other organic compounds. Bio-oil can be upgraded and refined into biofuels such as biodiesel, renewable diesel, or gasoline through processes such as hydrotreating, esterification, or catalytic cracking, offering alternatives to petroleum-based fuels and reducing greenhouse gas emissions.
- Syngas: Syngas, also known as synthesis gas or producer gas, is the gaseous fraction of pyrolysis consisting primarily of carbon monoxide (CO), hydrogen (H2), carbon dioxide (CO2), methane (CH4), and other trace gases. Syngas can be used as a fuel for heat and power generation, chemical synthesis, or conversion into biofuels such as hydrogen, methane, or synthetic natural gas (SNG) through processes such as steam reforming, Fischer-Tropsch synthesis, or methanation.
- Cooling and Condensation Stage: After pyrolysis, the biochar, bio-oil, and syngas products are cooled and condensed to separate them into their respective phases. Biochar is typically recovered as a solid residue, while bio-oil is collected as a liquid product, and syngas is captured as a gaseous stream.
Pyrolysis offers several advantages for biomass utilization and energy production:
- Resource Utilization: Pyrolysis enables the conversion of biomass feedstocks, including agricultural residues, forestry residues, energy crops, and organic waste, into valuable products, such as biochar, bio-oil, and syngas, maximizing resource utilization and reducing waste disposal.
- Energy Recovery: Pyrolysis processes recover energy from biomass feedstocks in the form of biochar, bio-oil, and syngas, which can be utilized for heat and power generation, biofuels production, or chemical synthesis, displacing fossil fuels and reducing greenhouse gas emissions.
- Carbon Sequestration: Pyrolysis produces biochar as a stable form of organic carbon that can be applied to soils to enhance soil carbon storage, improve soil fertility, and mitigate climate change by sequestering carbon in agricultural ecosystems.
- Environmental Benefits: Pyrolysis processes reduce air pollution and greenhouse gas emissions compared to conventional combustion technologies by minimizing incomplete combustion and volatile organic compound emissions, promoting cleaner and more sustainable energy production.
However, pyrolysis technology also presents challenges and considerations, including feedstock variability, process scalability, product quality and stability, energy efficiency, and economic viability. Addressing these challenges requires ongoing research, development, and deployment of pyrolysis technologies, as well as supportive policies, incentives, and market mechanisms to promote biomass utilization and renewable energy deployment.
Overall, pyrolysis plays a critical role in advancing towards a more sustainable, low-carbon energy future by converting biomass resources into valuable biochar, bio-oil, and syngas products, while also promoting environmental stewardship, economic development, and energy security.
Steam Reforming:
Steam reforming, also known as steam methane reforming (SMR), is a chemical process used to produce hydrogen gas (H2) from hydrocarbon feedstocks, typically natural gas or methane (CH4), in the presence of steam (H2O) and a catalyst. This highly endothermic reaction is the most common method for industrial hydrogen production, accounting for the majority of global hydrogen production.
The steam reforming process can be summarized by the following reaction:
CH4 + H2O ⟶ CO + 3H2
In this reaction, methane reacts with steam to produce carbon monoxide (CO) and hydrogen gas (H2). The reaction is typically carried out at elevated temperatures (around 700°C to 900°C) and moderate pressures (around 20 to 30 bar) in the presence of a catalyst, usually nickel (Ni) or platinum (Pt) supported on a high-surface-area substrate such as alumina (Al2O3).
The steam reforming process occurs in several sequential steps:
- Steam-Methane Reaction: Methane (CH4) and steam (H2O) are fed into a reformer reactor containing a catalyst bed. In the presence of the catalyst, methane reacts with steam to produce carbon monoxide (CO) and hydrogen gas (H2) through a series of endothermic reactions.
- Water-Gas Shift Reaction: The water-gas shift reaction (WGSR) occurs simultaneously with steam reforming, where carbon monoxide (CO) reacts with steam (H2O) to produce additional hydrogen gas (H2) and carbon dioxide (CO2):CO + H2O ⟶ CO2 + H2This reaction helps to shift the equilibrium towards the production of hydrogen gas and reduces the concentration of carbon monoxide in the syngas stream.
- Heat Management: The steam reforming reaction is highly endothermic, requiring a significant amount of heat input to drive the reaction forward. Heat is typically supplied to the reformer reactor through external heating sources, such as natural gas combustion or electric heaters, to maintain the desired temperature range for reaction kinetics.
- Product Separation: The resulting syngas mixture, consisting primarily of hydrogen gas (H2), carbon monoxide (CO), carbon dioxide (CO2), steam (H2O), and trace impurities, is cooled and passed through a series of separation units to remove impurities and by-products. These separation units may include water-gas shift reactors, pressure swing adsorption (PSA) units, or membrane separation systems.
Steam reforming offers several advantages for hydrogen production:
- High Efficiency: Steam reforming is a highly efficient process for hydrogen production, with typical efficiencies exceeding 80% in commercial-scale facilities.
- Abundant Feedstock: Natural gas, the primary feedstock for steam reforming, is abundant and widely available, making steam reforming a cost-effective and scalable technology for large-scale hydrogen production.
- Flexible Operation: Steam reforming can be operated flexibly to accommodate fluctuations in demand and supply, allowing for on-demand hydrogen production to meet varying market requirements.
- Product Purity: Steam reforming produces high-purity hydrogen gas suitable for a wide range of industrial applications, including ammonia synthesis, petroleum refining, chemical manufacturing, and fuel cells.
However, steam reforming also presents some challenges and considerations:
- Carbon Emissions: Steam reforming produces carbon dioxide (CO2) as a by-product, contributing to greenhouse gas emissions and climate change. To address this issue, carbon capture and storage (CCS) technologies can be employed to capture and sequester CO2 emissions from steam reforming facilities.
- Energy Intensity: Steam reforming is an energy-intensive process, requiring large amounts of heat energy for reaction heating and steam generation. Improving energy efficiency and heat integration can help reduce energy consumption and operating costs.
- Feedstock Availability: Steam reforming relies on the availability of natural gas or methane feedstocks, which may be subject to price fluctuations, supply constraints, and geopolitical risks.
Overall, steam reforming is a key technology for industrial hydrogen production, providing a reliable and cost-effective pathway to meet growing demand for clean and sustainable hydrogen energy. As the world transitions towards a low-carbon economy, steam reforming, along with advancements in carbon capture and utilization, renewable hydrogen production, and electrolysis technologies, will play a crucial role in realizing a hydrogen-based energy future.
Fischer-Tropsch Synthesis:
Fischer-Tropsch (FT) synthesis is a catalytic chemical process used to convert synthesis gas (syngas) into liquid hydrocarbons and other valuable products. It was developed in the 1920s by Franz Fischer and Hans Tropsch and has since become a key technology for producing liquid fuels, chemicals, and waxes from coal, natural gas, biomass, or other carbonaceous feedstocks.
The Fischer-Tropsch process involves a series of complex reactions that occur over heterogeneous catalysts under controlled temperature, pressure, and composition conditions. The general reaction can be represented as follows:
nCO + (2n+1)H2 ⟶ CnH(2n+2) + nH2O
In this reaction, carbon monoxide (CO) and hydrogen gas (H2) react to form higher hydrocarbons (CnH(2n+2)) and water (H2O). The synthesis gas feedstock typically consists of a mixture of CO and H2 in a stoichiometric ratio of 1:2, although variations in composition and ratio can be accommodated depending on the desired product slate.
The Fischer-Tropsch synthesis process can be divided into several steps:
- Adsorption: The reactant gases, CO and H2, are adsorbed onto the surface of the catalyst particles, where they undergo activation and dissociation into reactive intermediates, such as surface-bound CO and H atoms.
- Surface Reactions: The activated CO and H atoms undergo a series of surface reactions, including hydrogenation, carbon chain growth, and chain termination, to form a range of hydrocarbon products, including alkanes, alkenes, and oxygenated compounds.
- Desorption: The hydrocarbon products desorb from the catalyst surface and are removed from the reactor as liquid or gaseous products. Water (H2O) is also formed as a by-product of the reaction and is typically removed from the product stream through condensation and separation.
- Product Upgrading: The liquid hydrocarbon products obtained from Fischer-Tropsch synthesis may undergo additional upgrading and refining processes, such as distillation, hydrogenation, hydrocracking, and isomerization, to produce finished fuels, lubricants, or specialty chemicals with the desired properties and specifications.
Fischer-Tropsch synthesis offers several advantages for liquid fuels and chemicals production:
- Fuel Flexibility: Fischer-Tropsch synthesis can utilize a wide range of feedstocks, including coal, natural gas, biomass, or syngas derived from renewable sources, providing flexibility in feedstock selection and availability.
- Product Versatility: Fischer-Tropsch synthesis can produce a variety of hydrocarbon products, including diesel, gasoline, jet fuel, waxes, lubricants, and chemicals, which can be tailored to meet specific market demands and applications.
- Cleaner Fuels: Fischer-Tropsch synthetic fuels have lower sulfur, nitrogen, and aromatic content compared to conventional petroleum-derived fuels, resulting in lower emissions of particulate matter, sulfur oxides, and nitrogen oxides, and reduced environmental impact.
- Energy Security: Fischer-Tropsch synthesis enables the production of liquid fuels and chemicals from domestic or locally available feedstocks, reducing dependence on imported oil and enhancing energy security and resilience.
However, Fischer-Tropsch synthesis also presents challenges and considerations:
- Catalyst Performance: Fischer-Tropsch catalysts must exhibit high activity, selectivity, and stability over extended periods of operation to maximize product yield and minimize by-product formation. Catalyst deactivation, fouling, and poisoning can occur due to factors such as catalyst aging, coke deposition, and impurity poisoning.
- Process Optimization: Fischer-Tropsch synthesis requires careful control and optimization of reaction conditions, including temperature, pressure, gas composition, and catalyst properties, to achieve the desired product distribution, quality, and yield. Process optimization involves balancing competing reactions, managing heat and mass transfer, and minimizing energy consumption and operating costs.
- Economic Viability: Fischer-Tropsch synthesis is a capital-intensive process with high upfront investment costs, requiring large-scale production facilities to achieve economies of scale and competitive production costs. The economic viability of Fischer-Tropsch projects depends on factors such as feedstock availability, product market demand, regulatory requirements, and financial incentives.
Overall, Fischer-Tropsch synthesis is a versatile and important technology for converting synthesis gas into valuable liquid fuels and chemicals, contributing to energy security, economic development, and environmental sustainability. As global demand for clean and sustainable energy continues to grow, Fischer-Tropsch synthesis will play a significant role in diversifying energy sources, reducing greenhouse gas emissions, and advancing towards a more sustainable, low-carbon energy future.
Anaerobic Digestion:
Anaerobic digestion is a biological process that converts organic materials, such as agricultural residues, animal manure, food waste, and wastewater sludge, into biogas and digestate in the absence of oxygen (anaerobic conditions). It is a natural decomposition process carried out by microorganisms known as methanogens, which break down complex organic compounds into simpler molecules through a series of biochemical reactions.
The anaerobic digestion process can be divided into several stages:
- Hydrolysis: In the first stage of anaerobic digestion, complex organic polymers such as carbohydrates, proteins, and lipids are hydrolyzed by hydrolytic bacteria into soluble organic compounds such as sugars, amino acids, and fatty acids. This step involves the breakdown of large molecules into smaller molecules that can be easily metabolized by the microorganisms.
- Acidogenesis: In the acidogenesis stage, acidogenic bacteria ferment the soluble organic compounds produced during hydrolysis into volatile fatty acids (VFAs) such as acetic acid, propionic acid, and butyric acid, as well as alcohols, ammonia, and carbon dioxide. This step results in the production of organic acids and other intermediates that serve as substrates for methane production in the subsequent stages.
- Acetogenesis: In the acetogenesis stage, acetogenic bacteria further metabolize the volatile fatty acids and alcohols produced during acidogenesis into acetic acid (CH3COOH) and hydrogen gas (H2) through acetogenesis reactions. This step involves the conversion of organic acids and alcohols into acetate and hydrogen, which are key precursors for methane production in the methanogenesis stage.
- Methanogenesis: In the methanogenesis stage, methanogenic archaea utilize the acetate, hydrogen, and carbon dioxide produced during acetogenesis to produce methane (CH4) and carbon dioxide (CO2) through methanogenesis reactions. This step involves the conversion of acetate and hydrogen into methane and carbon dioxide by specific groups of methanogenic microorganisms, resulting in the production of biogas as the primary end product of anaerobic digestion.
The biogas produced from anaerobic digestion typically consists of methane (50-70%), carbon dioxide (30-50%), and trace amounts of other gases such as hydrogen sulfide (H2S), ammonia (NH3), and water vapor (H2O). Biogas can be utilized as a renewable energy source for heat and power generation, cogeneration, or upgraded to biomethane for injection into natural gas pipelines or use as a transportation fuel.
In addition to biogas, anaerobic digestion also produces digestate, a nutrient-rich organic fertilizer that can be used as soil amendment to improve soil fertility, water retention, and crop productivity. Digestate contains valuable nutrients such as nitrogen, phosphorus, potassium, and organic matter, which can enhance soil health, reduce the need for chemical fertilizers, and promote sustainable agriculture practices.
Anaerobic digestion offers several advantages for organic waste management and renewable energy production:
- Waste Valorization: Anaerobic digestion provides a sustainable and environmentally friendly solution for treating organic waste streams, including agricultural residues, food waste, and wastewater sludge, reducing greenhouse gas emissions, odors, and pathogens associated with traditional waste disposal methods such as landfilling or incineration.
- Renewable Energy Production: Anaerobic digestion produces biogas as a renewable energy source that can be used for heat and power generation, replacing fossil fuels and reducing dependence on non-renewable energy sources. Biogas can also be upgraded to biomethane for injection into natural gas pipelines or used as a transportation fuel, further displacing fossil fuel consumption and reducing greenhouse gas emissions.
- Nutrient Recycling: Anaerobic digestion converts organic nutrients from waste streams into digestate, a valuable organic fertilizer that can be recycled back to agricultural soils to improve soil fertility, nutrient cycling, and crop yields, closing the nutrient loop and promoting circular economy principles.
- Climate Change Mitigation: Anaerobic digestion reduces greenhouse gas emissions by capturing and utilizing methane produced during organic waste decomposition, mitigating climate change and contributing to global efforts to reduce carbon emissions and achieve sustainability goals.
However, anaerobic digestion also presents challenges and considerations:
- Feedstock Composition: The efficiency of anaerobic digestion depends on the composition and characteristics of the feedstock, including its organic content, moisture content, nutrient composition, and inhibitory substances such as heavy metals, toxins, or inhibitors, which can affect microbial activity and biogas production.
- Process Stability: Anaerobic digestion is sensitive to changes in operating conditions, such as temperature, pH, hydraulic retention time (HRT), and organic loading rate (OLR), which can impact process stability, biogas yield, and digestate quality. Maintaining optimal process conditions and monitoring system performance are essential for maximizing biogas production and nutrient recovery.
- Digestate Management: The application of digestate as a soil amendment requires careful consideration of nutrient management practices, land application rates, crop nutrient requirements, and environmental regulations to prevent nutrient runoff, soil erosion, or groundwater contamination, and ensure sustainable agricultural practices.
- Economic Viability: The economic viability of anaerobic digestion projects depends on factors such as feedstock availability, biogas yield, energy prices, digestate value, capital investment costs, operational expenses, and government incentives or subsidies, which can affect project feasibility, profitability, and long-term sustainability.
Overall, anaerobic digestion is a promising technology for organic waste management, renewable energy production, and nutrient recycling, offering environmental, economic, and social benefits for sustainable development and resource management. As the world transitions towards a low-carbon, circular economy, anaerobic digestion will play an increasingly important role in addressing global challenges such as climate change, resource scarcity, and waste management, while promoting energy security, environmental stewardship, and socio-economic development.
Renewable Natural Gas (RNG):
Renewable Natural Gas (RNG), also known as biomethane or biogas, is a renewable energy resource derived from organic materials such as agricultural waste, food waste, wastewater, landfill gas, or dedicated energy crops through anaerobic digestion, landfill gas recovery, or thermochemical conversion processes. RNG is chemically identical to conventional natural gas (fossil-derived methane) and can be used interchangeably for the same applications, including heat and power generation, transportation fuel, and industrial processes.
The production of RNG typically involves the following steps:
- Feedstock Collection: Organic feedstocks, such as agricultural residues, food waste, livestock manure, or wastewater sludge, are collected from sources such as farms, food processing facilities, municipalities, or landfills. These feedstocks contain biodegradable organic matter that can be converted into biogas through anaerobic digestion or thermochemical conversion processes.
- Conversion Process: The organic feedstocks undergo anaerobic digestion, landfill gas recovery, or thermochemical conversion processes to produce biogas, a mixture of methane (CH4), carbon dioxide (CO2), and trace gases such as hydrogen sulfide (H2S), ammonia (NH3), and water vapor (H2O). Biogas can also be upgraded to biomethane, a purified form of methane with high methane content (typically over 90%), through gas purification and upgrading technologies such as pressure swing adsorption (PSA), water scrubbing, or membrane separation.
- Gas Purification: Biogas is purified to remove impurities such as carbon dioxide, hydrogen sulfide, moisture, and trace contaminants, which can affect the quality, performance, and safety of the RNG product. Gas purification technologies such as adsorption, absorption, membrane separation, or chemical scrubbing are used to separate methane from other gases and produce high-purity RNG suitable for various applications.
- Injection or Distribution: The purified RNG product, known as biomethane or RNG, is injected into natural gas pipelines for distribution to end-users such as residential, commercial, or industrial customers, or used as a transportation fuel for compressed natural gas (CNG) or liquefied natural gas (LNG) vehicles. RNG can also be utilized onsite for heat and power generation, cogeneration, or combined heat and power (CHP) applications, displacing fossil fuels and reducing greenhouse gas emissions.
- End-Use Applications: RNG can be used for a variety of end-use applications, including:
- Heat and Power Generation: RNG can be combusted in boilers, turbines, or engines to produce heat and electricity for residential, commercial, or industrial applications, providing space heating, water heating, or process steam generation.
- Transportation Fuel: RNG can be used as a renewable transportation fuel for natural gas vehicles (NGVs) or converted into renewable hydrogen for fuel cell vehicles (FCVs), reducing greenhouse gas emissions and improving air quality compared to conventional fossil fuels.
- Industrial Processes: RNG can be utilized as a feedstock for industrial processes such as chemical synthesis, hydrogen production, or thermal applications, replacing fossil-derived methane or hydrogen and reducing the carbon footprint of industrial operations.
RNG offers several environmental, economic, and social benefits as a renewable energy resource:
- Greenhouse Gas Reduction: RNG production and utilization reduce greenhouse gas emissions by capturing and utilizing methane from organic waste streams, mitigating climate change, and reducing dependence on fossil fuels.
- Waste Management: RNG provides a sustainable solution for managing organic waste streams such as agricultural residues, food waste, and wastewater, diverting waste from landfills, reducing methane emissions, and promoting circular economy principles.
- Energy Security: RNG diversifies energy sources and reduces reliance on imported fossil fuels, enhancing energy security, resilience, and independence for communities, industries, and nations.
- Economic Development: RNG production creates jobs, stimulates rural economies, and provides additional revenue streams for farmers, waste management facilities, and renewable energy developers, contributing to economic development and poverty reduction.
- Air Quality Improvement: RNG utilization reduces air pollutants such as sulfur oxides (SOx), nitrogen oxides (NOx), particulate matter (PM), and volatile organic compounds (VOCs) compared to conventional fossil fuels, improving air quality and public health outcomes.
However, RNG production and utilization also present challenges and considerations:
- Feedstock Availability: RNG production depends on the availability and accessibility of organic feedstocks, which may vary seasonally, geographically, or by feedstock type, requiring reliable and diverse feedstock sources to ensure continuous and sustainable production.
- Infrastructure Investment: Expanding RNG production and distribution infrastructure requires significant investment in biogas upgrading facilities, pipeline injection systems, fueling stations, and vehicle fleets, as well as regulatory approvals, permits, and safety standards compliance.
- Policy and Regulation: Regulatory frameworks, incentives, mandates, and market mechanisms play a critical role in supporting RNG development, incentivizing investment, and creating favorable market conditions for RNG production, distribution, and utilization.
- Technology Innovation: Continued research, development, and deployment of advanced RNG production and utilization technologies are needed to improve process efficiency, reduce costs, increase scalability, and enhance environmental performance, ensuring the long-term sustainability and viability of RNG as a renewable energy resource.
Overall, RNG is a versatile and promising renewable energy resource that offers significant potential for decarbonizing energy systems, reducing greenhouse gas emissions, and advancing towards a more sustainable, low-carbon energy future. As global efforts to address climate change, energy security, and sustainable development accelerate, RNG will play an increasingly important role in transitioning towards a circular economy, promoting resource efficiency, and fostering resilience and prosperity for future generations.
Gasification:
Gasification is a thermochemical conversion process that converts carbonaceous feedstocks, such as biomass, coal, or municipal solid waste, into synthesis gas (syngas) consisting primarily of carbon monoxide (CO), hydrogen (H2), carbon dioxide (CO2), and trace contaminants such as methane (CH4) and hydrogen sulfide (H2S). Gasification occurs in the presence of a controlled amount of oxygen (or air) and a gasification agent, such as steam, carbon dioxide, or oxygen, at elevated temperatures (typically between 700°C and 1,500°C) and reduced pressures (usually atmospheric or slightly above atmospheric pressure).
The gasification process can be summarized by the following chemical reactions:
- Partial Oxidation: The carbonaceous feedstock undergoes partial oxidation reactions in the presence of limited oxygen (or air) to produce carbon monoxide (CO) and hydrogen gas (H2) according to the following reactions:C + O2 ⟶ CO2C + H2O ⟶ CO + H2
- Steam Reforming: In addition to partial oxidation, steam reforming reactions occur simultaneously, where carbon monoxide (CO) reacts with steam (H2O) to produce additional hydrogen gas (H2) and carbon dioxide (CO2) through the water-gas shift reaction:CO + H2O ⟶ CO2 + H2
- Carbon Gasification: The remaining carbonaceous residue undergoes further gasification reactions with steam and carbon dioxide to produce additional carbon monoxide (CO) and hydrogen gas (H2) through the following reactions:C + H2O ⟶ CO + H2C + CO2 ⟶ 2CO
The resulting syngas mixture is a versatile and valuable intermediate product that can be utilized for various energy and chemical applications, including:
- Heat and Power Generation: Syngas can be combusted in boilers, turbines, or engines to produce heat and electricity for residential, commercial, or industrial applications, providing space heating, water heating, or process steam generation.
- Chemical Synthesis: Syngas serves as a feedstock for chemical synthesis processes, such as Fischer-Tropsch synthesis, methanol synthesis, ammonia synthesis, and hydrogen production, enabling the production of liquid fuels, chemicals, and intermediates from renewable or non-renewable carbon sources.
- Renewable Fuels: Syngas can be further processed into renewable fuels such as biofuels (e.g., ethanol, biodiesel), renewable natural gas (RNG or biomethane), or hydrogen gas (H2) through catalytic conversion, upgrading, or purification processes, offering alternatives to petroleum-based fuels and reducing greenhouse gas emissions.
Gasification offers several advantages for energy production and resource utilization:
- Feedstock Flexibility: Gasification can utilize a wide range of feedstocks, including biomass, coal, municipal solid waste, or industrial residues, providing flexibility in feedstock selection and availability, and enabling the use of diverse carbon sources for energy production.
- Efficient Energy Conversion: Gasification converts carbonaceous feedstocks into syngas with high energy efficiency, typically exceeding 70%, and allows for the recovery of energy and value-added products from waste streams, reducing waste disposal costs and environmental impact.
- Reduced Environmental Impact: Gasification reduces greenhouse gas emissions, air pollutants, and solid waste generation compared to conventional combustion technologies by promoting cleaner and more efficient energy conversion, minimizing air emissions, and enabling the utilization of renewable or low-carbon feedstocks.
- Resource Recovery: Gasification enables the recovery of energy, heat, and valuable by-products from organic waste streams, promoting resource recovery, circular economy principles, and sustainable development.
However, gasification also presents challenges and considerations:
- Complexity and Integration: Gasification is a complex and capital-intensive process that requires careful design, operation, and integration with downstream processes such as syngas cleanup, gas conditioning, and product upgrading to maximize efficiency, reliability, and performance.
- Feedstock Quality and Variability: Gasification performance depends on the quality, composition, and characteristics of the feedstock, including moisture content, ash content, heating value, and contaminants, which can affect gasification efficiency, process stability, and product quality.
- Syngas Cleanup and Conditioning: Syngas produced from gasification contains impurities such as tar, sulfur compounds, ammonia, and particulates, which must be removed or reduced through syngas cleanup and conditioning processes to meet quality specifications for downstream applications, such as chemical synthesis or power generation.
- Scale-Up and Commercialization: Scaling up gasification technologies from laboratory or pilot-scale to commercial-scale facilities requires overcoming technical, economic, and regulatory barriers, including demonstration of technology readiness, securing financing and investment, and navigating regulatory approvals and permitting processes.
Overall, gasification is a promising technology for converting carbonaceous feedstocks into syngas, renewable fuels, and value-added products, offering opportunities for energy diversification, waste valorization, and environmental stewardship. As the world transitions towards a low-carbon, circular economy, gasification will play an increasingly important role in advancing sustainable energy production, resource management, and climate change mitigation efforts.
Carbon Capture and Storage (CCS):
Carbon Capture and Storage (CCS) is a suite of technologies and processes designed to capture carbon dioxide (CO2) emissions from industrial facilities, power plants, and other large point sources, transport the captured CO2 to suitable storage sites, and inject it into underground geological formations for long-term storage and containment, preventing its release into the atmosphere and mitigating climate change. CCS plays a crucial role in reducing greenhouse gas emissions and achieving climate targets by capturing CO2 emissions from industrial sectors such as power generation, cement production, steel manufacturing, and chemical processing, which account for a significant portion of global CO2 emissions.
The CCS process typically involves the following steps:
- Capture: CO2 capture technologies capture CO2 emissions from industrial processes or power plant flue gases before they are released into the atmosphere. There are three main types of CO2 capture technologies:
- Post-combustion capture: CO2 is captured from flue gases after combustion using chemical solvents or adsorbents.
- Pre-combustion capture: CO2 is captured from syngas produced through gasification of fossil fuels or biomass before combustion.
- Oxy-fuel combustion: Fossil fuels are burned in an oxygen-rich environment to produce a flue gas stream consisting mainly of CO2 and water vapor, which can be easily captured and separated.
- Transport: Captured CO2 is compressed into a dense fluid state and transported via pipelines, ships, or trucks to suitable storage sites, typically located near CO2 sources or industrial hubs. CO2 transport infrastructure is essential for delivering captured CO2 to storage sites efficiently and safely.
- Storage: Captured CO2 is injected into deep underground geological formations, such as depleted oil and gas reservoirs, saline aquifers, or unmineable coal seams, for long-term storage and containment. The injected CO2 displaces formation fluids (e.g., water or hydrocarbons) and becomes trapped in porous rock formations sealed by impermeable caprocks, preventing its migration and ensuring secure storage.
CCS offers several potential benefits for climate change mitigation and sustainable development:
- Emissions Reduction: CCS enables significant reductions in CO2 emissions from industrial sectors and power generation, allowing fossil fuel-based industries to continue operating while reducing their carbon footprint and environmental impact.
- Climate Mitigation: CCS contributes to global efforts to mitigate climate change by capturing and storing CO2 emissions, reducing atmospheric greenhouse gas concentrations, and limiting global warming and climate-related impacts.
- Energy Security: CCS can be deployed in conjunction with fossil fuel-based power generation and industrial processes, providing a bridge to a low-carbon energy future and enhancing energy security and reliability by diversifying energy sources and reducing dependence on imported fossil fuels.
- Economic Opportunities: CCS creates economic opportunities for technology development, infrastructure investment, job creation, and revenue generation, particularly in regions with abundant fossil fuel resources, industrial activity, or suitable geological storage potential.
- Carbon Removal: CCS can be combined with bioenergy production (Bioenergy with Carbon Capture and Storage or BECCS) to achieve negative emissions by removing CO2 from the atmosphere through the growth of biomass feedstocks, capturing CO2 emissions from biomass combustion or gasification, and storing the captured CO2 underground.
However, CCS also presents challenges and considerations:
- Cost and Financing: CCS deployment involves significant upfront capital investment and operating costs, including CO2 capture, transport, and storage infrastructure, which may require financial incentives, subsidies, or carbon pricing mechanisms to be economically viable and competitive with alternative low-carbon technologies.
- Technology Readiness: CCS technologies are still under development and demonstration at commercial scale, and further research, innovation, and cost reductions are needed to improve technology performance, efficiency, and reliability, and address technical challenges such as solvent degradation, corrosion, and energy penalties.
- Regulatory Framework: CCS deployment requires supportive policy frameworks, regulatory incentives, and legal frameworks for CO2 storage rights, liability, monitoring, and verification, as well as public acceptance and stakeholder engagement to address environmental, safety, and social concerns related to CO2 storage and transport.
- Storage Capacity and Leakage Risks: Identifying suitable CO2 storage sites with adequate geological storage capacity, integrity, and containment security is essential for long-term CO2 storage, and monitoring and verification measures are needed to detect and mitigate potential leakage risks or environmental impacts.
- Integration with Renewable Energy: CCS deployment should be integrated with renewable energy technologies, energy efficiency measures, and sustainable development strategies to achieve synergies, optimize energy systems, and minimize environmental impacts while transitioning to a low-carbon energy future.
Overall, CCS is a promising technology for reducing CO2 emissions from industrial sectors and power generation, contributing to climate change mitigation efforts, and enabling the transition to a sustainable, low-carbon economy. As global demand for energy continues to grow and the urgency to address climate change increases, CCS will play an increasingly important role in decarbonizing energy systems, promoting sustainable development, and safeguarding the environment for future generations.
Coal Gasification:
Coal gasification is a thermochemical process that converts coal into synthesis gas (syngas), a mixture of carbon monoxide (CO), hydrogen (H2), carbon dioxide (CO2), methane (CH4), and other trace gases, through the reaction of coal with a controlled amount of oxygen (or air) and steam at elevated temperatures (typically between 700°C and 1,200°C) and pressures. Coal gasification offers an alternative approach to conventional coal combustion for energy production and chemical synthesis, providing opportunities for cleaner and more efficient utilization of coal resources while reducing environmental emissions and pollutants.
The coal gasification process can be summarized by the following chemical reactions:
- Coal Pyrolysis: In the initial stage of coal gasification, coal undergoes thermal decomposition (pyrolysis) in the absence of oxygen (or air) to produce volatile matter, tar, and char. The volatile matter contains hydrocarbons, aromatic compounds, and other organic constituents, while the char consists of carbonaceous residue.
- Char Gasification: The char produced during coal pyrolysis reacts with oxygen (or air) and steam to produce carbon monoxide (CO) and hydrogen gas (H2) through the following reactions:C + O2 ⟶ CO2C + H2O ⟶ CO + H2
- Water-Gas Shift Reaction: In addition to char gasification, carbon monoxide (CO) reacts with steam (H2O) to produce additional hydrogen gas (H2) and carbon dioxide (CO2) through the water-gas shift reaction:CO + H2O ⟶ CO2 + H2
- Methane Reforming: In the presence of steam and hydrogen, methane (CH4) reforming reactions occur, where methane reacts with steam to produce additional hydrogen gas (H2) and carbon monoxide (CO):CH4 + H2O ⟶ CO + 3H2
The resulting syngas mixture contains carbon monoxide (CO) and hydrogen (H2) as the primary components, along with carbon dioxide (CO2), methane (CH4), and trace contaminants such as hydrogen sulfide (H2S), ammonia (NH3), and volatile organic compounds (VOCs). Syngas can be utilized for various energy and chemical applications, including:
- Power Generation: Syngas can be combusted in boilers, turbines, or engines to produce heat and electricity for residential, commercial, or industrial applications, providing space heating, water heating, or process steam generation.
- Chemical Synthesis: Syngas serves as a feedstock for chemical synthesis processes such as Fischer-Tropsch synthesis, methanol synthesis, ammonia synthesis, and hydrogen production, enabling the production of liquid fuels, chemicals, and intermediates from coal or coal-derived syngas.
- Hydrogen Production: Syngas can be further processed to produce high-purity hydrogen gas (H2) through water-gas shift, methane reforming, or membrane separation processes, which can be utilized as a clean fuel for transportation, industrial processes, or fuel cells.
Coal gasification offers several potential benefits for energy production and environmental stewardship:
- Efficient Coal Utilization: Coal gasification enables the conversion of coal into syngas with higher energy efficiency and lower environmental emissions compared to conventional coal combustion, allowing for cleaner and more efficient utilization of coal resources while reducing greenhouse gas emissions and pollutants.
- Pollution Control: Coal gasification reduces emissions of sulfur dioxide (SO2), nitrogen oxides (NOx), particulate matter (PM), and mercury (Hg) compared to conventional coal combustion by capturing pollutants during gasification and syngas cleanup processes, leading to improved air quality and public health outcomes.
- Carbon Capture: Coal gasification facilitates the integration of carbon capture and storage (CCS) technologies for capturing and storing carbon dioxide (CO2) emissions from coal-fired power plants or industrial facilities, enabling CO2 mitigation and climate change mitigation efforts.
- Fuel Flexibility: Coal gasification can utilize a wide range of coal types, including bituminous coal, sub-bituminous coal, lignite, or anthracite, as well as coal blends or waste coals, providing flexibility in feedstock selection and availability for energy production and chemical synthesis.
However, coal gasification also presents challenges and considerations:
- High Capital Costs: Coal gasification projects involve significant upfront capital investment in gasification plants, syngas cleanup systems, and associated infrastructure, which may require financial incentives, subsidies, or carbon pricing mechanisms to be economically viable and competitive with alternative low-carbon technologies.
- Technology Complexity: Coal gasification is a complex and technically challenging process that requires careful design, operation, and integration with downstream processes such as syngas cleanup, gas conditioning, and product upgrading to maximize efficiency, reliability, and performance.
- Environmental Impacts: While coal gasification reduces emissions of conventional pollutants such as sulfur dioxide (SO2) and nitrogen oxides (NOx), it still produces greenhouse gas emissions, carbon dioxide (CO2), and other trace contaminants such as methane (CH4) and volatile organic compounds (VOCs), which must be managed and mitigated to minimize environmental impacts and comply with regulatory requirements.
- Carbon Management: Coal gasification projects must address carbon management challenges such as carbon capture, utilization, and storage (CCUS), including the development of CO2 capture technologies, identification of suitable CO2 storage sites, and implementation of monitoring, verification, and reporting (MRV) protocols to ensure secure and permanent CO2 storage and containment.
Overall, coal gasification is a promising technology for converting coal into syngas, renewable fuels, and value-added products, offering opportunities for cleaner and more efficient energy production, industrial processes, and chemical synthesis. As the world transitions towards a low-carbon, sustainable energy future, coal gasification will play an increasingly important role in decarbonizing energy systems, promoting environmental stewardship, and addressing global challenges such as climate change and energy security.
Biomass Gasification:
Biomass gasification is a thermochemical process that converts biomass feedstocks, such as wood, agricultural residues, energy crops, or organic waste, into synthesis gas (syngas) consisting primarily of carbon monoxide (CO), hydrogen (H2), carbon dioxide (CO2), methane (CH4), and trace contaminants such as tar, ammonia, and particulates. Biomass gasification offers a sustainable and renewable alternative to fossil fuels for energy production and chemical synthesis, utilizing organic materials derived from plant or animal sources to produce clean and versatile syngas for various applications.
The biomass gasification process can be summarized by the following steps:
- Biomass Preparation: Biomass feedstocks are prepared by size reduction (e.g., chipping, grinding, shredding) and drying to improve handling, transportation, and gasification performance. Biomass materials may undergo pretreatment to remove impurities, moisture, or contaminants that can affect gasification efficiency and syngas quality.
- Gasification Reaction: Biomass feedstocks undergo thermochemical conversion in a gasifier reactor under controlled conditions of temperature, pressure, and residence time. The gasification reaction occurs in the presence of a controlled amount of oxygen (or air) and steam, which react with the biomass to produce syngas through a series of chemical reactions:
- Pyrolysis: Biomass undergoes thermal decomposition (pyrolysis) in the absence of oxygen (or air) to produce volatile gases, tars, and char.
- Char Gasification: Char produced during pyrolysis reacts with oxygen (or air) and steam to produce carbon monoxide (CO) and hydrogen gas (H2) through gasification reactions.
- Water-Gas Shift Reaction: Carbon monoxide (CO) reacts with steam (H2O) to produce additional hydrogen gas (H2) and carbon dioxide (CO2) through the water-gas shift reaction.
- Syngas Cleanup: The raw syngas produced from biomass gasification contains impurities such as tar, ammonia, sulfur compounds, and particulates, which must be removed or reduced to meet quality specifications for downstream applications. Syngas cleanup technologies such as filtration, scrubbing, catalytic conversion, or tar cracking are employed to improve syngas quality and remove contaminants.
- Syngas Utilization: The cleaned syngas can be utilized for various energy and chemical applications, including:
- Heat and Power Generation: Syngas can be combusted in boilers, turbines, or engines to produce heat and electricity for residential, commercial, or industrial applications, providing space heating, water heating, or process steam generation.
- Chemical Synthesis: Syngas serves as a feedstock for chemical synthesis processes such as Fischer-Tropsch synthesis, methanol synthesis, ammonia synthesis, and hydrogen production, enabling the production of liquid fuels, chemicals, and intermediates from biomass-derived syngas.
- Renewable Fuels: Syngas can be further processed into renewable fuels such as biofuels (e.g., ethanol, biodiesel), renewable natural gas (RNG or biomethane), or hydrogen gas (H2) through catalytic conversion, upgrading, or purification processes, offering alternatives to petroleum-based fuels and reducing greenhouse gas emissions.
Biomass gasification offers several advantages for sustainable energy production and environmental stewardship:
- Renewable Energy: Biomass gasification utilizes renewable biomass feedstocks derived from plant or animal sources, providing a sustainable and carbon-neutral alternative to fossil fuels for energy production and chemical synthesis, reducing greenhouse gas emissions and dependence on finite fossil fuel resources.
- Waste Valorization: Biomass gasification enables the utilization of organic waste streams such as agricultural residues, forestry residues, energy crops, or organic waste for energy production, waste reduction, and resource recovery, diverting waste from landfills and promoting circular economy principles.
- Energy Efficiency: Biomass gasification converts biomass feedstocks into syngas with high energy efficiency, typically exceeding 60%, and allows for the recovery of energy and value-added products from biomass residues, reducing waste disposal costs and environmental impact.
- Climate Change Mitigation: Biomass gasification reduces greenhouse gas emissions by capturing and utilizing carbon dioxide (CO2) from biomass feedstocks, mitigating climate change, and contributing to global efforts to achieve carbon neutrality and sustainability goals.
However, biomass gasification also presents challenges and considerations:
- Feedstock Availability: Biomass gasification projects require reliable and sustainable sources of biomass feedstocks, which may vary seasonally, geographically, or by feedstock type, necessitating access to diverse biomass resources and supply chains to ensure continuous and reliable operation.
- Technology Complexity: Biomass gasification is a complex and technically challenging process that requires careful design, operation, and integration with downstream processes such as syngas cleanup, gas conditioning, and product upgrading to maximize efficiency, reliability, and performance, requiring skilled personnel and expertise.
- Syngas Cleanup and Conditioning: Syngas produced from biomass gasification contains impurities such as tar, ammonia, sulfur compounds, and particulates, which must be removed or reduced through syngas cleanup and conditioning processes to meet quality specifications for downstream applications, such as chemical synthesis or power generation.
- Economic Viability: Biomass gasification projects must be economically viable and competitive with alternative energy technologies, requiring consideration of factors such as capital investment costs, feedstock prices, energy prices, government incentives or subsidies, and market demand for syngas or value-added products.
Overall, biomass gasification is a promising technology for converting biomass into syngas, renewable fuels, and value-added products, offering opportunities for sustainable energy production, waste valorization, and environmental stewardship. As the world transitions towards a low-carbon, circular economy, biomass gasification will play an increasingly important role in decarbonizing energy systems, promoting resource efficiency, and fostering resilience and prosperity for future generations.
Syngas Cleaning:
Syngas cleaning, also known as syngas conditioning or gas cleanup, is a crucial step in the gasification process that involves removing impurities and contaminants from raw syngas to meet quality specifications for downstream applications such as power generation, chemical synthesis, or renewable fuels production. Raw syngas produced from gasification processes typically contains various impurities such as tar, particulates, sulfur compounds, ammonia, alkali metals, and trace contaminants, which can adversely affect the performance, efficiency, and durability of syngas utilization technologies and equipment.
The syngas cleaning process typically involves multiple stages of purification and conditioning to remove or reduce impurities and contaminants to acceptable levels. The specific syngas cleaning technologies and methods employed depend on the composition of the raw syngas, the feedstock used for gasification, the desired syngas quality, and the requirements of the downstream applications. Some common syngas cleaning technologies and methods include:
- Particulate Removal: Particulate matter, such as ash, dust, and char fines, is removed from raw syngas through mechanical filtration or cyclone separators to prevent fouling and erosion of downstream equipment such as turbines, engines, or catalyst beds.
- Tar Removal: Tar, a complex mixture of hydrocarbons and organic compounds formed during gasification, is removed from raw syngas through tar cracking, tar absorption, or tar filtration processes to prevent fouling, corrosion, and catalyst deactivation in downstream processes such as chemical synthesis or power generation.
- Sulfur Removal: Sulfur compounds, such as hydrogen sulfide (H2S), carbonyl sulfide (COS), and sulfur dioxide (SO2), are removed from raw syngas through sulfur removal processes such as adsorption, absorption, chemical conversion, or catalytic oxidation to prevent corrosion, catalyst poisoning, and environmental emissions.
- Ammonia Removal: Ammonia (NH3), a common impurity in raw syngas derived from nitrogen-containing feedstocks such as biomass or waste, is removed through ammonia scrubbing, ammonia adsorption, or selective catalytic reduction (SCR) processes to prevent catalyst deactivation and environmental emissions.
- Alkali Metal Removal: Alkali metals such as sodium (Na) and potassium (K), which are present in biomass-derived syngas as ash constituents, are removed through alkali scrubbing, alkali adsorption, or alkali conversion processes to prevent catalyst poisoning and corrosion in downstream processes.
- Particulate Removal: Particulate matter, such as ash, dust, and char fines, is removed from raw syngas through mechanical filtration or cyclone separators to prevent fouling and erosion of downstream equipment such as turbines, engines, or catalyst beds.
- Water Removal: Water vapor and moisture are removed from raw syngas through condensation, dehydration, or membrane separation processes to prevent corrosion, ice formation, and efficiency losses in downstream equipment and processes.
- Gas Cooling: Raw syngas is cooled to ambient temperature or to a specified temperature range to facilitate downstream processing and handling and to prevent overheating or thermal degradation of syngas cleaning equipment.
The selection and optimization of syngas cleaning technologies depend on factors such as syngas composition, impurity concentrations, process conditions, economic considerations, and performance requirements. Syngas cleaning is often integrated into gasification plant design and operation to ensure efficient and reliable syngas production and utilization while minimizing environmental impacts and maximizing product quality and value.
Overall, syngas cleaning is a critical process in gasification-based energy and chemical production systems, ensuring the quality, reliability, and environmental performance of syngas for various applications. As gasification technologies continue to advance and commercialize, syngas cleaning will play an increasingly important role in enabling the widespread deployment and utilization of gasification for sustainable energy production, waste management, and resource utilization.
Gasification Reactor Design:
Gasification reactor design plays a pivotal role in the efficiency, performance, and reliability of gasification processes for converting carbonaceous feedstocks into synthesis gas (syngas). The design of the gasification reactor encompasses various aspects, including reactor type, configuration, operating conditions, heat and mass transfer mechanisms, residence time, and reaction kinetics, which influence gasification efficiency, syngas quality, and process economics. Several types of gasification reactors are employed in practice, each with unique characteristics and advantages:
- Fixed-Bed Gasifiers: Fixed-bed gasifiers consist of a stationary bed of solid carbonaceous feedstock, typically arranged in a vertical or horizontal configuration, through which a gasification agent such as air, oxygen, or steam is introduced from the bottom or sides of the reactor. Gasification reactions occur as the feedstock moves downward through the reactor bed, with heat supplied externally through combustion or through internal heat exchange mechanisms. Fixed-bed gasifiers offer good fuel flexibility, high carbon conversion efficiency, and robust operation but may suffer from bed agglomeration, ash fusion, and limited scalability.
- Fluidized-Bed Gasifiers: Fluidized-bed gasifiers utilize a bed of inert particles (e.g., sand, alumina) that are fluidized by a gasification agent such as air, oxygen, or steam, creating a turbulent and highly reactive environment for gasification reactions to occur. Fluidized-bed gasifiers offer excellent mixing, heat transfer, and residence time control, allowing for efficient conversion of a wide range of feedstocks, high reaction rates, and tolerance to feedstock variability. However, fluidized-bed gasifiers may require sophisticated controls, suffer from bed material attrition, and exhibit higher tar content in syngas compared to other reactor types.
- Entrained-Flow Gasifiers: Entrained-flow gasifiers entrain pulverized or finely ground feedstock particles into a high-velocity stream of gasification agent (e.g., oxygen, steam) and combustible gases (e.g., recycled syngas, natural gas) in a refractory-lined reactor, where gasification reactions occur in suspension at elevated temperatures and pressures. Entrained-flow gasifiers offer high thermal efficiency, rapid heat and mass transfer, and excellent syngas quality, making them suitable for large-scale applications and high-throughput feedstocks such as coal or petroleum coke. However, entrained-flow gasifiers may require sophisticated refractory materials, erosion-resistant designs, and stringent control of operating conditions to prevent slagging, fouling, or corrosion.
- Hybrid Gasifiers: Hybrid gasifiers combine features of multiple reactor types (e.g., fixed-bed, fluidized-bed, entrained-flow) to leverage their respective advantages and address their limitations, offering flexibility, scalability, and performance optimization for specific feedstocks, process conditions, and product requirements. Hybrid gasification systems may incorporate staged reactors, integrated heat exchangers, or novel reactor designs to enhance gasification efficiency, syngas quality, and process integration.
Gasification reactor design considerations include:
- Feedstock Properties: The selection of gasification reactor type and design depends on the properties of the carbonaceous feedstock, including particle size, moisture content, ash composition, heating value, and reactivity, which influence reactor performance, feedstock handling, and gasification kinetics.
- Operating Conditions: Gasification reactor design must accommodate the operating conditions required for efficient gasification, including temperature, pressure, gas residence time, gasification agent composition, and heat transfer mechanisms, which affect reaction kinetics, syngas composition, and reactor performance.
- Syngas Quality: Gasification reactor design impacts syngas composition, temperature, cleanliness, and contaminants content, which influence downstream processing, product quality, and environmental emissions, necessitating optimization of reactor design parameters for desired syngas characteristics.
- Process Integration: Gasification reactor design should consider integration with downstream processes such as syngas cleanup, gas conditioning, heat recovery, and product synthesis to maximize overall process efficiency, resource utilization, and value-added product recovery.
Overall, gasification reactor design is a multidisciplinary endeavor that combines principles of chemical engineering, thermodynamics, fluid dynamics, heat transfer, and materials science to develop innovative and efficient reactor systems for sustainable energy production, waste management, and resource utilization. As gasification technologies continue to evolve and mature, advancements in reactor design will drive improvements in performance, reliability, and cost-effectiveness, enabling the widespread deployment and adoption of gasification for clean energy and chemical production applications.
Syngas Utilization:
Syngas, a versatile mixture of carbon monoxide (CO) and hydrogen (H2), along with carbon dioxide (CO2), methane (CH4), and trace contaminants, is produced through gasification processes from carbonaceous feedstocks such as coal, biomass, or waste materials. Syngas has diverse applications across various industries, including power generation, chemical synthesis, renewable fuels production, and industrial processes, offering opportunities for clean energy production, resource utilization, and value-added product manufacturing.
- Power Generation: Syngas can be combusted in gas turbines, reciprocating engines, or combined-cycle power plants to produce heat and electricity for residential, commercial, or industrial applications. Gasification-based power generation offers higher efficiency, lower emissions, and fuel flexibility compared to conventional combustion technologies, enabling clean and reliable electricity generation from a wide range of feedstocks.
- Chemical Synthesis: Syngas serves as a feedstock for chemical synthesis processes such as Fischer-Tropsch synthesis, methanol synthesis, ammonia synthesis, and hydrogen production, enabling the production of a wide range of chemicals, fuels, and intermediates. Syngas-derived products include liquid fuels (e.g., gasoline, diesel, jet fuel), chemicals (e.g., methanol, hydrogen, ammonia), plastics, solvents, and synthetic materials used in various industrial sectors such as transportation, agriculture, and manufacturing.
- Renewable Fuels Production: Syngas can be further processed into renewable fuels such as biofuels (e.g., ethanol, biodiesel), renewable natural gas (RNG or biomethane), or hydrogen gas (H2) through catalytic conversion, upgrading, or purification processes. Renewable fuels derived from syngas offer alternatives to petroleum-based fuels, reduce greenhouse gas emissions, and enhance energy security and sustainability.
- Hydrogen Production: Syngas can be reformed or purified to produce high-purity hydrogen gas (H2) through water-gas shift, methane reforming, or membrane separation processes. Hydrogen derived from syngas serves as a clean and versatile fuel for transportation, industrial processes, chemical synthesis, and fuel cells, offering zero-emission energy solutions and enabling the transition to a hydrogen economy.
- Bioenergy Production: Syngas can be utilized in bioenergy production processes such as anaerobic digestion, biomass gasification, or thermochemical conversion, where biomass feedstocks are converted into syngas through gasification or pyrolysis processes. Syngas-derived bioenergy offers renewable and carbon-neutral energy sources for heat, power, and fuel production, contributing to climate change mitigation and sustainable development goals.
- Waste Management: Syngas can be produced from organic waste materials such as agricultural residues, forestry residues, municipal solid waste, or wastewater sludge through gasification processes, providing opportunities for waste valorization, resource recovery, and circular economy principles. Syngas-derived energy and products reduce waste disposal costs, landfill emissions, and environmental impacts while promoting resource efficiency and sustainability.
Syngas utilization offers several advantages for clean energy production, environmental stewardship, and economic development, including:
- Fuel Flexibility: Syngas can be produced from a wide range of feedstocks, including coal, biomass, waste materials, or natural gas, providing flexibility and resilience in energy supply and reducing dependence on finite fossil fuel resources.
- Emissions Reduction: Syngas utilization enables lower emissions of greenhouse gases, particulates, sulfur compounds, and other pollutants compared to conventional combustion technologies, contributing to improved air quality, public health, and environmental sustainability.
- Resource Efficiency: Syngas utilization maximizes the energy and value recovery from carbonaceous feedstocks, converting waste materials into useful energy products, chemicals, and materials, while minimizing waste generation, disposal costs, and environmental impacts.
- Energy Security: Syngas-derived energy offers reliable, decentralized, and diversified energy sources that can enhance energy security, resilience, and independence, particularly in regions with abundant biomass resources, waste streams, or stranded natural gas reserves.
- Technological Innovation: Syngas utilization drives technological innovation and research in gasification, catalysis, process integration, and product development, advancing clean energy technologies, sustainable manufacturing processes, and circular economy solutions.
Despite these advantages, syngas utilization also presents challenges and considerations, including:
- Technology Maturity: Syngas utilization technologies such as gasification, synthesis, and purification are still evolving and may require further research, development, and demonstration to improve efficiency, reliability, and cost-effectiveness for widespread commercial deployment.
- Economic Viability: Syngas utilization projects must be economically viable and competitive with alternative energy sources or products, requiring consideration of factors such as capital costs, feedstock prices, energy prices, government incentives, and market demand.
- Infrastructure Development: Syngas utilization may require investment in infrastructure for feedstock collection, processing, transportation, and distribution, as well as integration with existing energy, chemical, or industrial infrastructure, which may pose logistical, regulatory, or financial challenges.
- Environmental Impacts: Syngas utilization processes may generate emissions, byproducts, or waste streams that require management and mitigation to minimize environmental impacts such as greenhouse gas emissions, air and water pollution, land use, and ecosystem disruption.
Overall, syngas utilization offers promising opportunities for clean energy production, sustainable development, and environmental stewardship, driving the transition towards a low-carbon, circular economy. As technology advances, policy support, and market demand grow, syngas utilization will play an increasingly important role in decarbonizing energy systems, promoting resource efficiency, and fostering resilience and prosperity for future generations.
Syngas Conditioning:
Syngas conditioning, also known as syngas cleanup or gas treatment, is a critical process in gasification systems that involves removing impurities and contaminants from raw syngas to meet quality specifications for downstream applications such as power generation, chemical synthesis, or renewable fuels production. Raw syngas produced from gasification processes typically contains various impurities such as tar, particulates, sulfur compounds, ammonia, alkali metals, and trace contaminants, which can adversely affect the performance, efficiency, and durability of syngas utilization technologies and equipment.
Syngas conditioning aims to achieve the following objectives:
- Tar Removal: Tar, a complex mixture of hydrocarbons and organic compounds formed during gasification, is removed from raw syngas to prevent fouling, corrosion, and catalyst deactivation in downstream processes such as power generation or chemical synthesis. Tar removal technologies include tar cracking, tar absorption, or tar filtration processes, which break down or capture tar molecules and convert them into simpler, more manageable compounds.
- Particulate Removal: Particulate matter, such as ash, dust, and char fines, is removed from raw syngas through mechanical filtration, cyclone separators, or electrostatic precipitators to prevent fouling and erosion of downstream equipment such as turbines, engines, or catalyst beds. Particulate removal improves syngas cleanliness, reduces wear and tear on equipment, and enhances process reliability and efficiency.
- Sulfur Removal: Sulfur compounds, such as hydrogen sulfide (H2S), carbonyl sulfide (COS), and sulfur dioxide (SO2), are removed from raw syngas through sulfur removal processes such as adsorption, absorption, chemical conversion, or catalytic oxidation to prevent corrosion, catalyst poisoning, and environmental emissions. Sulfur removal technologies utilize sorbents, scrubbing solutions, or catalytic reactors to capture or convert sulfur compounds into harmless byproducts.
- Ammonia Removal: Ammonia (NH3), a common impurity in raw syngas derived from nitrogen-containing feedstocks such as biomass or waste, is removed through ammonia scrubbing, ammonia adsorption, or selective catalytic reduction (SCR) processes to prevent catalyst deactivation and environmental emissions. Ammonia removal technologies utilize acidic or basic solutions, adsorbents, or catalytic converters to capture or convert ammonia into inert nitrogen gas.
- Alkali Metal Removal: Alkali metals such as sodium (Na) and potassium (K), which are present in biomass-derived syngas as ash constituents, are removed through alkali scrubbing, alkali adsorption, or alkali conversion processes to prevent catalyst poisoning and corrosion in downstream processes. Alkali metal removal technologies utilize acidic or basic solutions, adsorbents, or chemical reactions to capture or convert alkali metals into stable compounds.
- Water Removal: Water vapor and moisture are removed from raw syngas through condensation, dehydration, or membrane separation processes to prevent corrosion, ice formation, and efficiency losses in downstream equipment and processes. Water removal technologies include chilling, cooling, desiccation, or membrane filtration processes, which reduce water content to acceptable levels for downstream applications.
Syngas conditioning technologies and methods are selected based on factors such as syngas composition, impurity concentrations, process conditions, economic considerations, and performance requirements. Syngas conditioning is often integrated into gasification plant design and operation to ensure efficient and reliable syngas production and utilization while minimizing environmental impacts and maximizing product quality and value.
Overall, syngas conditioning is a critical step in gasification-based energy and chemical production systems, ensuring the quality, reliability, and environmental performance of syngas for various applications. As gasification technologies continue to advance and commercialize, advancements in syngas conditioning will drive improvements in performance, reliability, and cost-effectiveness, enabling the widespread deployment and adoption of gasification for clean energy and sustainable development.
Syngas Cleanup Technologies:
Syngas cleanup technologies are essential components of gasification processes, responsible for removing impurities and contaminants from raw syngas to meet quality specifications for downstream applications. Raw syngas produced from gasification processes typically contains various impurities such as tar, particulates, sulfur compounds, ammonia, alkali metals, and trace contaminants, which can adversely affect the performance, efficiency, and durability of syngas utilization technologies and equipment. Syngas cleanup technologies employ a variety of physical, chemical, and catalytic processes to achieve efficient removal and conversion of impurities, ensuring the cleanliness, reliability, and environmental performance of syngas for various applications.
- Tar Cracking: Tar, a complex mixture of hydrocarbons and organic compounds formed during gasification, is decomposed or cracked into simpler, more manageable compounds through thermal or catalytic processes. Tar cracking technologies include high-temperature pyrolysis, catalytic cracking, or reforming reactions, which break down tar molecules into smaller hydrocarbons, hydrogen, and carbon monoxide. Tar cracking improves syngas cleanliness, reduces fouling and corrosion in downstream equipment, and enhances process reliability and efficiency.
- Particulate Filtration: Particulate matter, such as ash, dust, and char fines, is removed from raw syngas through mechanical filtration, cyclone separators, or electrostatic precipitators. Particulate filtration technologies capture solid particles suspended in syngas streams, preventing fouling and erosion of downstream equipment such as turbines, engines, or catalyst beds. Particulate filtration improves syngas quality, reduces wear and tear on equipment, and enhances process reliability and efficiency.
- Sulfur Removal: Sulfur compounds, such as hydrogen sulfide (H2S), carbonyl sulfide (COS), and sulfur dioxide (SO2), are removed from raw syngas through sulfur removal processes such as adsorption, absorption, chemical conversion, or catalytic oxidation. Sulfur removal technologies utilize sorbents, scrubbing solutions, or catalytic reactors to capture or convert sulfur compounds into harmless byproducts. Sulfur removal improves syngas cleanliness, prevents corrosion and catalyst poisoning, and reduces environmental emissions of sulfur-containing compounds.
- Ammonia Scrubbing: Ammonia (NH3), a common impurity in raw syngas derived from nitrogen-containing feedstocks such as biomass or waste, is removed through ammonia scrubbing processes using acidic or basic solutions. Ammonia scrubbing technologies utilize chemical reactions between ammonia and scrubbing agents such as sulfuric acid, aqueous ammonia, or alkaline solutions to capture ammonia and convert it into stable compounds. Ammonia scrubbing prevents catalyst deactivation and environmental emissions of ammonia, enhancing syngas quality and environmental performance.
- Alkali Metal Capture: Alkali metals such as sodium (Na) and potassium (K), which are present in biomass-derived syngas as ash constituents, are captured through alkali scrubbing processes using acidic or basic solutions. Alkali scrubbing technologies utilize chemical reactions between alkali metals and scrubbing agents such as sulfuric acid, hydrochloric acid, or alkaline solutions to capture alkali metals and convert them into stable compounds. Alkali metal capture prevents catalyst poisoning and corrosion in downstream processes, improving syngas quality and reliability.
- Water-Gas Shift Reaction: The water-gas shift reaction (WGSR) converts carbon monoxide (CO) and steam (H2O) into hydrogen (H2) and carbon dioxide (CO2) through catalytic or non-catalytic reactions. WGSR technologies utilize catalysts such as iron oxide, copper oxide, or cobalt-molybdenum to enhance reaction rates and selectivity, producing high-purity hydrogen gas (H2) for various applications. WGSR improves syngas composition, increases hydrogen yield, and reduces carbon monoxide content, enhancing downstream processes such as chemical synthesis or hydrogen production.
- Carbon Dioxide Capture: Carbon dioxide (CO2), a major component of raw syngas and a greenhouse gas, is captured through carbon dioxide capture and storage (CCS) technologies. CCS technologies utilize physical or chemical processes such as absorption, adsorption, or membrane separation to capture CO2 from syngas streams and sequester it underground or utilize it for enhanced oil recovery (EOR) or industrial applications. CO2 capture reduces greenhouse gas emissions, mitigates climate change, and enhances environmental sustainability of gasification processes.
Syngas cleanup technologies are selected and optimized based on factors such as syngas composition, impurity concentrations, process conditions, economic considerations, and performance requirements. Syngas cleanup is often integrated into gasification plant design and operation to ensure efficient and reliable syngas production and utilization while minimizing environmental impacts and maximizing product quality and value.
Overall, syngas cleanup technologies are critical enablers of gasification-based energy and chemical production systems, ensuring the quality, reliability, and environmental performance of syngas for various applications. As gasification technologies continue to advance and commercialize, advancements in syngas cleanup will drive improvements in performance, reliability, and cost-effectiveness, enabling the widespread deployment and adoption of gasification for clean energy and sustainable development.
Syngas Utilization in Chemical Synthesis:
Syngas, a versatile mixture of carbon monoxide (CO) and hydrogen (H2), along with carbon dioxide (CO2), methane (CH4), and trace contaminants, is a valuable feedstock for chemical synthesis processes. Chemical synthesis using syngas enables the production of a wide range of chemicals, fuels, and intermediates through catalytic reactions, offering opportunities for clean energy production, resource utilization, and value-added product manufacturing.
- Fischer-Tropsch Synthesis (FTS): Fischer-Tropsch synthesis is a catalytic process that converts syngas into liquid hydrocarbons, such as gasoline, diesel, and jet fuel, as well as waxes and lubricants. The FTS process involves three main steps: (1) synthesis gas generation from carbonaceous feedstocks, (2) catalytic conversion of syngas into hydrocarbons over Fischer-Tropsch catalysts (e.g., iron, cobalt, ruthenium), and (3) product separation and upgrading to obtain desired fuel or chemical products. FTS offers opportunities for producing liquid fuels from diverse feedstocks, including coal, natural gas, biomass, or waste materials, and can contribute to energy security, transportation fuel diversification, and reduced greenhouse gas emissions.
- Methanol Synthesis: Methanol synthesis is a catalytic process that converts syngas into methanol (CH3OH), a versatile chemical intermediate used in various industrial applications, such as solvents, fuels, plastics, and chemicals. The methanol synthesis process typically employs copper-based catalysts under high pressure and temperature conditions to facilitate the conversion of CO and H2 into methanol. Methanol synthesis offers opportunities for producing renewable and sustainable chemicals from biomass-derived syngas or waste-derived syngas, contributing to carbon neutrality, circular economy principles, and green chemistry initiatives.
- Ammonia Synthesis: Ammonia synthesis is a catalytic process that converts syngas into ammonia (NH3), a key building block for nitrogen-based fertilizers, explosives, and chemicals. The Haber-Bosch process, developed in the early 20th century, utilizes iron-based catalysts under high pressure and temperature conditions to facilitate the conversion of nitrogen (N2) from air and hydrogen (H2) from syngas into ammonia. Ammonia synthesis plays a critical role in global agriculture, providing essential nutrients for crop production, food security, and sustainable agriculture practices.
- Methane Synthesis: Methane synthesis, also known as methanation, is a catalytic process that converts syngas into methane (CH4), the primary component of natural gas. Methane synthesis typically employs nickel-based catalysts under moderate pressure and temperature conditions to facilitate the conversion of CO and H2 into methane. Methane synthesis offers opportunities for producing renewable natural gas (RNG or biomethane) from biomass-derived syngas or waste-derived syngas, enabling energy recovery from organic waste materials and reducing greenhouse gas emissions from landfills, wastewater treatment plants, or agricultural operations.
- Higher Alcohol Synthesis: Higher alcohol synthesis is a catalytic process that converts syngas into higher alcohols, such as ethanol, propanol, butanol, and pentanol, which serve as valuable chemicals, fuels, or blending components for gasoline or diesel. Higher alcohol synthesis typically employs copper- or cobalt-based catalysts under specific reaction conditions to facilitate the conversion of CO and H2 into longer-chain alcohols. Higher alcohol synthesis offers opportunities for producing renewable and sustainable alcohols from biomass-derived syngas or waste-derived syngas, contributing to biofuel production, energy security, and carbon mitigation strategies.
- Olefins Synthesis: Olefins synthesis is a catalytic process that converts syngas into olefins, such as ethylene (C2H4) and propylene (C3H6), which serve as key building blocks for plastics, chemicals, and polymers. Olefins synthesis typically employs zeolite-based catalysts under specific reaction conditions to facilitate the conversion of CO and H2 into light olefins. Olefins synthesis offers opportunities for producing renewable and sustainable olefins from biomass-derived syngas or waste-derived syngas, enabling the transition towards bio-based plastics, renewable materials, and green chemistry initiatives.
Syngas utilization in chemical synthesis offers several advantages for sustainable development, environmental stewardship, and economic prosperity:
- Feedstock Flexibility: Syngas can be produced from a wide range of feedstocks, including coal, natural gas, biomass, or waste materials, providing flexibility and resilience in feedstock supply and enabling utilization of diverse and renewable resources for chemical synthesis.
- Product Diversification: Syngas utilization enables the production of a wide range of chemicals, fuels, and intermediates through catalytic reactions, offering opportunities for product diversification, value-added processing, and market differentiation in chemical, fuel, and polymer industries.
- Carbon Neutrality: Syngas-derived chemicals and fuels offer opportunities for carbon neutrality or negative emissions when produced from renewable feedstocks such as biomass or waste materials, contributing to climate change mitigation, carbon sequestration, and sustainable development goals.
- Resource Efficiency: Syngas utilization maximizes the energy and value recovery from carbonaceous feedstocks, converting waste materials into useful chemicals, fuels, and materials, while minimizing waste generation, disposal costs, and environmental impacts.
Despite these advantages, syngas utilization in chemical synthesis also presents challenges and considerations,
Syngas Utilization in Power Generation:
Syngas, a mixture primarily composed of carbon monoxide (CO) and hydrogen (H2), along with carbon dioxide (CO2), methane (CH4), and trace contaminants, is utilized in power generation applications as a clean and versatile fuel source. Derived from gasification processes, syngas offers several advantages for power generation, including fuel flexibility, reduced emissions, and efficient energy conversion. Syngas can be utilized in various power generation technologies, including gas turbines, internal combustion engines, and combined heat and power (CHP) systems, enabling the production of electricity and thermal energy for residential, commercial, and industrial applications.
- Gas Turbines: Gas turbines are a common technology for syngas-based power generation, offering high efficiency, fast startup times, and low emissions. In a gas turbine system, syngas is combusted in a combustion chamber, producing high-temperature, high-pressure gases that drive a turbine to generate electricity. Gas turbines are suitable for both base-load and peaking power generation applications, providing flexibility and reliability in electricity supply. Advanced gas turbine designs incorporate features such as heat recovery steam generators (HRSGs) and combined cycle configurations to maximize energy efficiency and thermal performance.
- Internal Combustion Engines: Internal combustion engines, such as reciprocating engines or gas engines, are another option for syngas-based power generation, particularly in distributed energy systems or remote locations. In an internal combustion engine system, syngas is mixed with air and ignited in a combustion chamber, driving pistons or rotors to generate mechanical energy, which is then converted into electricity through a generator. Internal combustion engines offer flexibility in fuel use, scalability, and fast response times, making them suitable for decentralized power generation, backup power systems, or grid stabilization applications.
- Combined Heat and Power (CHP) Systems: Combined heat and power (CHP) systems, also known as cogeneration systems, utilize syngas for simultaneous generation of electricity and thermal energy (e.g., steam or hot water) in a single integrated system. In a CHP system, syngas is combusted in a prime mover (e.g., gas turbine, engine, or fuel cell) to generate electricity, while waste heat from the combustion process is captured and utilized for heating, cooling, or industrial processes. CHP systems offer high energy efficiency, reduced emissions, and cost savings compared to separate electricity and heat generation, making them attractive for district heating, industrial facilities, and commercial buildings.
- Combined Cycle Power Plants: Combined cycle power plants integrate gas turbine technology with steam turbine technology to maximize energy efficiency and overall performance. In a combined cycle power plant, syngas is combusted in a gas turbine to generate electricity, and the exhaust gases are then directed to a heat recovery steam generator (HRSG), where they produce steam to drive a steam turbine for additional electricity generation. Combined cycle power plants offer high efficiency, fuel flexibility, and low emissions, making them ideal for large-scale power generation applications such as utility-scale power plants or industrial facilities.
Syngas utilization in power generation offers several advantages for clean energy production, environmental sustainability, and energy security:
- Fuel Flexibility: Syngas can be produced from a wide range of feedstocks, including coal, natural gas, biomass, or waste materials, providing flexibility and resilience in fuel supply and reducing dependence on finite fossil fuel resources.
- Emissions Reduction: Syngas-based power generation technologies offer lower emissions of greenhouse gases, particulates, sulfur compounds, and other pollutants compared to conventional combustion technologies, contributing to improved air quality, public health, and environmental sustainability.
- Energy Efficiency: Syngas-based power generation technologies such as gas turbines, internal combustion engines, and combined cycle plants offer high energy efficiency, rapid startup times, and load-following capabilities, enabling efficient and reliable electricity generation to meet fluctuating demand.
- Decentralized Energy: Syngas-based power generation technologies can be deployed in decentralized or distributed energy systems, providing reliable electricity supply to remote locations, off-grid communities, or industrial facilities with limited access to centralized power grids.
- Grid Integration: Syngas-based power generation technologies can be integrated into existing power grids, microgrids, or renewable energy systems to enhance grid stability, reliability, and resilience, contributing to the transition towards a sustainable and resilient energy infrastructure.
Despite these advantages, syngas utilization in power generation also presents challenges and considerations, including:
- Technology Maturity: Syngas-based power generation technologies such as gas turbines, internal combustion engines, and combined cycle plants require mature and reliable equipment, controls, and operational practices to ensure efficient and safe operation over the long term.
- Feedstock Quality: Syngas quality and composition can vary depending on the gasification process, feedstock type, and operating conditions, requiring careful monitoring, control, and conditioning to meet performance and emissions requirements for power generation applications.
- Infrastructure Requirements: Syngas-based power generation systems may require investment in infrastructure for syngas production, storage, transportation, and utilization, as well as integration with existing power generation, transmission, and distribution infrastructure, which may pose logistical, regulatory, or financial challenges.
- Economic Viability: Syngas-based power generation projects must be economically viable and competitive with alternative energy sources or technologies, requiring consideration of factors such as capital costs, operating costs, fuel prices, electricity prices, government incentives, and market demand.
Overall, syngas utilization in power generation offers promising opportunities for clean energy production, environmental sustainability, and energy security, driving the transition towards a low-carbon, resilient, and sustainable energy future. As technology advances, policy support, and market demand grow, syngas-based power generation will play an increasingly important role in decarbonizing energy systems, promoting energy access, and fostering economic development for future generations.
Syngas Utilization in Renewable Fuels Production:
Syngas, a versatile mixture of carbon monoxide (CO) and hydrogen (H2), along with carbon dioxide (CO2), methane (CH4), and trace contaminants, serves as a valuable feedstock for the production of renewable fuels. Derived from gasification processes using biomass, waste materials, or renewable feedstocks, syngas offers opportunities for sustainable and carbon-neutral fuels production, including biofuels, renewable natural gas (RNG), and hydrogen (H2). Syngas-derived renewable fuels contribute to reducing greenhouse gas emissions, enhancing energy security, and promoting the transition towards a low-carbon, sustainable energy economy.
- Biofuels Production: Syngas can be converted into liquid biofuels such as ethanol, biodiesel, or renewable diesel through catalytic conversion, Fischer-Tropsch synthesis, or alcohol synthesis processes. Biofuels produced from syngas offer renewable alternatives to petroleum-based fuels, reducing dependence on fossil fuels, mitigating greenhouse gas emissions, and promoting sustainable transportation solutions. Syngas-derived biofuels can be blended with conventional fuels or used as drop-in replacements in existing transportation infrastructure, engines, and vehicles, enabling the decarbonization of the transportation sector and enhancing energy security and resilience.
- Renewable Natural Gas (RNG) Production: Syngas can be upgraded and purified to produce renewable natural gas (RNG) or biomethane through methanation, purification, or upgrading processes. RNG produced from syngas offers a renewable and sustainable alternative to conventional natural gas, reducing methane emissions from organic waste materials such as agricultural residues, landfill gas, wastewater treatment plants, or anaerobic digestion processes. RNG can be injected into natural gas pipelines, distributed through existing gas infrastructure, or used as a transportation fuel for compressed natural gas (CNG) or liquefied natural gas (LNG) vehicles, contributing to carbon neutrality, circular economy principles, and waste valorization.
- Hydrogen (H2) Production: Syngas can be reformed, purified, or electrolyzed to produce high-purity hydrogen gas (H2) through water-gas shift, steam reforming, or electrolysis processes. Renewable hydrogen produced from syngas offers a clean and versatile energy carrier for transportation, industry, power generation, and chemical synthesis applications. Renewable hydrogen can be used in fuel cell vehicles, hydrogen fueling stations, industrial processes, ammonia production, or energy storage systems, enabling zero-emission energy solutions, grid balancing, and decarbonization of hard-to-abate sectors such as heavy industry, aviation, and shipping.
- Synthetic Natural Gas (SNG) Production: Syngas can be further processed into synthetic natural gas (SNG) through methanation or upgrading processes, where CO and H2 are converted into methane (CH4) to mimic the composition and properties of conventional natural gas. SNG produced from syngas offers a renewable and sustainable alternative to fossil natural gas, enabling energy storage, grid balancing, and renewable energy integration. SNG can be injected into natural gas pipelines, stored in underground storage facilities, or utilized for power generation, heating, or industrial processes, contributing to energy security, reliability, and flexibility in energy systems.
Syngas utilization in renewable fuels production offers several advantages for clean energy production, environmental sustainability, and economic development:
- Carbon Neutrality: Syngas-derived renewable fuels offer opportunities for carbon neutrality or negative emissions when produced from biomass-derived syngas or waste-derived syngas, contributing to climate change mitigation, carbon sequestration, and sustainable development goals.
- Resource Efficiency: Syngas utilization maximizes the energy and value recovery from renewable feedstocks, converting organic waste materials into useful energy products, fuels, and chemicals, while minimizing waste generation, disposal costs, and environmental impacts.
- Energy Security: Syngas-derived renewable fuels enhance energy security and resilience by diversifying energy sources, reducing dependence on finite fossil fuels, and promoting domestic production of renewable energy resources.
- Technological Innovation: Syngas utilization drives technological innovation and research in biomass conversion, catalysis, process integration, and product development, advancing clean energy technologies, sustainable manufacturing processes, and circular economy solutions.
Despite these advantages, syngas utilization in renewable fuels production also presents challenges and considerations, including:
- Feedstock Availability: Syngas production from biomass or waste materials may be limited by feedstock availability, quality, seasonality, and logistics, requiring sustainable sourcing strategies, feedstock management practices, and supply chain optimization to ensure reliable and cost-effective feedstock supply.
- Technology Scalability: Syngas utilization technologies such as gasification, synthesis, and upgrading processes may require further research, development, and demonstration to scale up from pilot or demonstration projects to commercial-scale deployment, reducing costs, improving efficiency, and enhancing reliability for widespread adoption.
- Market Acceptance: Syngas-derived renewable fuels may face challenges in market acceptance, pricing, and regulatory compliance compared to conventional fossil fuels, requiring policy support, market incentives, and public awareness to promote adoption, investment, and deployment in energy markets.
- Infrastructure Development: Syngas-derived renewable fuels may require investment in infrastructure for production, storage, distribution, and utilization, as well as integration with existing energy, transportation, and industrial infrastructure, which may pose logistical, regulatory, or financial challenges.
Overall, syngas utilization in renewable fuels production offers promising opportunities for clean energy production, sustainable development, and environmental stewardship, driving the transition towards a low-carbon, circular economy. As technology advances, policy support, and market demand grow, syngas-based renewable fuels will play an increasingly important role in decarbonizing energy systems, promoting energy independence, and fostering economic prosperity for future generations.
Wood Gasifier
A wood gasifier electric generator is a system that converts wood or other biomass into a combustible gas called wood gas (or syngas) through a process known as gasification. This gas, primarily composed of carbon monoxide, hydrogen, and traces of methane, can then be used to fuel an internal combustion engine connected to an electric generator, producing electricity. Here’s a breakdown of how it works and its key aspects:
How It Works
- Gasification Process: Wood or biomass is placed in a gasifier, a device that heats the material in a low-oxygen environment. Instead of burning completely, the wood undergoes pyrolysis and partial combustion, releasing volatile gases (syngas) while leaving behind ash and charcoal.
- Gas Cleaning: The raw syngas contains impurities like tar, particulates, and moisture. It’s filtered and cooled to make it suitable for use in an engine.
- Power Generation: The cleaned syngas is fed into an internal combustion engine (often a modified gasoline or diesel engine), which drives a generator to produce electricity.
Benefits
- Renewable Energy: Uses wood or biomass, which can be sustainably sourced, making it a carbon-neutral option compared to fossil fuels.
- Versatility: Can power homes, farms, or remote locations where grid access is limited.
- Fuel Availability: Wood and biomass are often abundant and inexpensive, especially in rural or forested areas.
- Combined Heat and Power (CHP): Waste heat from the engine can be captured for heating, increasing overall efficiency.
Challenges
- Feedstock Quality: The wood needs to be dry (typically less than 20% moisture) and properly sized (e.g., chips or pellets) for efficient gasification.
- Maintenance: Tar and particulates can clog engines if not properly filtered, requiring regular upkeep.
- Efficiency: Output depends on the gasifier design and engine, often producing less power per unit of fuel than gasoline or diesel.
- Scale: While small systems exist for personal use, larger setups can be complex and costly.
Applications
Historically, wood gasifiers were widely used during World War II to power vehicles and machinery when gasoline was scarce. Today, they’re popular among off-grid enthusiasts, preppers, and sustainable energy advocates. Modern examples include small DIY setups for powering generators (e.g., 1-10 kW) and commercial systems producing up to hundreds of kilowatts for industrial use.
Practical Example
A typical small-scale system might use 20 pounds of wood to generate about 1 gallon of gasoline-equivalent energy, roughly enough to run a 1 kW generator for a few hours. Designs like the “stratified downdraft gasifier” (popularized by FEMA in 1989) are simple and effective for emergency power.
If you’re considering building or using one, factors like wood availability, system size, and intended use (backup power, off-grid living, etc.) will guide your approach. It’s a hands-on, sustainable way to generate electricity, but it requires some technical know-how and effort to operate effectively.
A wood gasifier electric generator converts wood or biomass into a combustible gas called wood gas or syngas through gasification, a process where the material is heated in a low-oxygen environment, undergoing pyrolysis and partial combustion to release gases like carbon monoxide, hydrogen, and methane, leaving ash and charcoal behind. This raw syngas, full of impurities like tar and particulates, gets filtered and cooled before being fed into an internal combustion engine—often a tweaked gasoline or diesel one—that drives a generator to produce electricity. It’s a renewable setup since wood can be sustainably sourced, making it carbon-neutral compared to fossil fuels, and it’s versatile enough to power homes, farms, or remote spots off the grid. Plus, the waste heat from the engine can be tapped for heating, boosting efficiency. But it’s not all smooth sailing—the wood needs to be dry, under 20% moisture, and chopped into chips or pellets, or the system clogs up, and tar can gum up the engine if the gas isn’t cleaned well, so maintenance is a regular chore. Efficiency-wise, it lags behind gasoline or diesel, and while small DIY rigs work for personal use, scaling up gets tricky and pricey. Back in World War II, these systems kept vehicles and machines running when fuel was scarce, and now they’re a hit with off-grid folks and sustainability buffs. A small setup might burn through 20 pounds of wood to match a gallon of gasoline’s energy, running a 1 kW generator for a few hours—think of designs like the FEMA stratified downdraft gasifier for something simple and emergency-ready. It’s a practical, hands-on option if you’ve got wood to spare and don’t mind tinkering, though it takes effort to keep it humming.
A wood gasifier electric generator transforms wood or other biomass into a flammable gas known as wood gas or syngas through a fascinating process called gasification, where the material is heated in a low-oxygen chamber, triggering pyrolysis and partial combustion that releases a mix of gases—mainly carbon monoxide, hydrogen, and a dash of methane—while leaving behind a residue of ash and charcoal. This raw syngas, though, isn’t ready straight out of the gasifier; it’s loaded with impurities like sticky tar, fine particulates, and moisture, so it has to be scrubbed, filtered, and cooled down to a clean state before it can be piped into an internal combustion engine—often a modified gasoline or diesel model—that spins a generator to churn out electricity. The beauty of this system lies in its renewable nature, tapping into wood or biomass that can be grown and harvested sustainably, offering a carbon-neutral alternative to fossil fuels, especially appealing in a world increasingly wary of emissions. It’s incredibly versatile too, capable of lighting up homes, powering farms, or keeping the lights on in remote corners where the grid doesn’t reach, and if you’re clever about it, you can capture the waste heat from the engine to warm water or a living space, squeezing out extra efficiency from the setup.
But it’s not without its quirks and demands. The wood going in has to be just right—dry as a bone, ideally below 20% moisture, and chopped into uniform chips or pellets—because wet or uneven fuel can choke the gasifier or slash its output. And then there’s the maintenance: tar and grit in the syngas can wreak havoc on an engine if the filtration isn’t spot-on, meaning you’re in for regular cleaning and tinkering to keep things running smoothly. Efficiency-wise, it’s no match for gasoline or diesel—pound for pound, you’re getting less bang for your buck—and while a small backyard rig might do the trick for personal use, scaling it up to industrial levels brings a whole new layer of complexity and cost that might give you pause. Historically, these contraptions were a lifeline during World War II, when fuel shortages forced folks to rig up cars and tractors to run on wood gas, and that legacy lives on today with off-grid enthusiasts, preppers, and green-energy advocates who see it as a rugged, self-reliant power source.
Picture this: a modest setup might chew through 20 pounds of wood to produce energy equivalent to a gallon of gasoline, enough to keep a 1 kW generator humming for a few hours, and something like the FEMA-designed stratified downdraft gasifier—laid out in a 1989 manual for emergency power—offers a straightforward blueprint for anyone handy with tools. It’s not a plug-and-play solution, though; you need a steady supply of wood, a willingness to get your hands dirty, and a bit of technical savvy to troubleshoot the inevitable hiccups. The gasifier itself can be a beast to master—too much air and you’re just burning the wood to ash, too little and the gas won’t flow—and the engine needs to be tuned to handle the lower energy density of syngas compared to liquid fuels. Still, there’s something satisfying about turning a pile of logs into usable electricity, especially if you’re in a spot where trees are plentiful and power lines are a distant dream. Modern takes on the tech range from scrappy DIY builds cobbled together from barrels and pipes to sleek commercial units pushing hundreds of kilowatts, but they all share that core promise: energy independence with a nod to the past. Whether you’re prepping for a blackout, cutting ties with the utility company, or just experimenting with sustainable tech, a wood gasifier generator is a gritty, practical option—assuming you’re ready to roll up your sleeves and keep it fed.
What Is a Wood Gasifier Electric Generator?
A wood gasifier electric generator is a system that turns wood or other biomass into a combustible gas called wood gas or syngas through a process known as gasification. Inside a gasifier, the wood is heated in a low-oxygen environment, triggering pyrolysis and partial combustion. This releases a mixture of gases—mostly carbon monoxide, hydrogen, and a bit of methane—while leaving behind ash and charcoal. The raw syngas is then cleaned of impurities like tar, particulates, and moisture, cooled, and fed into an internal combustion engine, typically a modified gasoline or diesel model, which drives a generator to produce electricity. It’s a clever way to harness renewable energy from something as simple as wood, making it a sustainable alternative to fossil fuels with a carbon-neutral footprint if the biomass is sourced responsibly.
How It Works
The process starts in the gasifier, where wood or biomass is loaded into a sealed chamber and heated with limited oxygen—enough to break it down but not enough to burn it completely. This produces syngas, a flammable gas that’s drawn off the top while ash and leftover charcoal settle at the bottom. That raw gas, though, is messy, full of tar and grit, so it’s passed through filters—sometimes as basic as cloth or sawdust—or cooled in a heat exchanger to strip out the junk. Once it’s clean, the syngas flows into an engine, where it’s ignited to power a generator, churning out electricity. You can even siphon off the engine’s waste heat for other uses, like warming a house or heating water, which bumps up the system’s overall efficiency. A small rig might use 20 pounds of wood to match a gallon of gasoline’s energy, running a 1 kW generator for a few hours.
Benefits
This setup shines as a renewable energy source—wood grows back, unlike oil or coal—and it’s perfect for off-grid living, powering homes, farms, or remote outposts where the grid’s a nonstarter. It’s also dirt cheap if you’ve got trees or biomass lying around, and it’s got a DIY appeal that draws in tinkerers and preppers alike. The ability to use waste heat for something useful, like hot water, is a bonus that makes it more efficient than it might seem at first glance. Plus, it’s got a storied past—during World War II, people kept cars and machinery running on wood gas when fuel was scarce, proving it’s a legit fallback in tough times.
Challenges
It’s not all rosy, though. The wood has to be dry—under 20% moisture—or the gasifier struggles, and it needs to be chipped or pelleted just right, or you’ll clog the works. Maintenance is a big deal; tar and particulates can gum up the engine if the gas isn’t filtered well, so you’re stuck cleaning filters and checking pipes regularly. Efficiency-wise, it’s a step down from gasoline or diesel—you’re burning more fuel for less power—and while a small system is manageable, scaling up to something industrial-sized gets complicated and expensive fast. It’s hands-on, too; you can’t just flip a switch and walk away like with a solar panel or diesel generator.
Applications and Practicality
Historically, wood gasifiers were a wartime hero, powering vehicles and factories when gasoline dried up, and today they’re a niche favorite for off-grid folks, survivalists, and green-energy buffs. A basic setup, like the stratified downdraft gasifier from FEMA’s 1989 emergency power guide, is simple enough for a DIY build—think barrels, pipes, and some elbow grease—and can keep a small generator humming. Modern versions range from scrappy backyard projects to commercial units pumping out hundreds of kilowatts. If you’ve got a steady wood supply and don’t mind the upkeep, it’s a rugged way to generate power, whether you’re prepping for a blackout, living off the land, or just experimenting with sustainable tech. But it takes know-how—balancing air flow, tuning the engine for syngas, troubleshooting tar buildup—and a willingness to get dirty to make it work.
How It Works
A wood gasifier electric generator operates by transforming wood or biomass into a usable fuel gas through a multi-step process that ultimately generates electricity. It begins with the gasifier, a specialized device where the magic of gasification happens. Wood—chopped into chips or pellets and ideally dried to below 20% moisture—is loaded into a sealed chamber. Here, it’s heated in a carefully controlled, low-oxygen environment. Unlike regular burning, where flames consume everything, this limited oxygen supply triggers pyrolysis and partial combustion. Pyrolysis breaks down the wood’s complex molecules into volatile gases, while the partial combustion keeps the process going without turning the whole load to ash. The result is a flammable gas mixture called wood gas or syngas, primarily made up of carbon monoxide, hydrogen, and traces of methane, with ash and charcoal left as byproducts at the bottom.
This raw syngas, however, isn’t ready to use straight away—it’s dirty, loaded with tar, fine particulates, and water vapor that could wreck an engine. So, it’s drawn out of the gasifier and sent through a cleanup phase. The gas might pass through filters—sometimes as simple as cloth bags, sawdust beds, or steel wool—or run through a cyclone separator to spin out heavier particles. It’s also cooled, often via a heat exchanger or radiator-like setup, which condenses out moisture and some of the stickier tar. By the end, you’ve got a cleaner, engine-ready syngas that’s still not as potent as gasoline but perfectly capable of doing the job.
Next, the cleaned syngas is piped into an internal combustion engine, typically a modified gasoline or diesel model tweaked to handle the gas’s lower energy density. A common tweak might involve adjusting the air-fuel mix or swapping out the carburetor for a gas mixer, since syngas burns differently than liquid fuel. Once in the engine, the syngas is ignited, driving pistons that turn a crankshaft, which is hooked up to an electric generator. The generator converts that mechanical energy into electricity—enough to power lights, tools, or whatever else you’ve got plugged in. For a rough idea, a small system might burn 20 pounds of wood to produce energy equivalent to a gallon of gasoline, running a 1 kW generator for a few hours.
There’s an extra perk, too: the engine throws off a lot of heat, and if you’re savvy, you can capture it with a heat exchanger to warm water or a space, boosting the system’s overall efficiency. The whole process is a balancing act—too much air in the gasifier and you’re just incinerating the wood, too little and the gas flow stalls—so it takes some finesse to keep it humming. But when dialed in, it’s a reliable, hands-on way to turn a pile of wood into usable power.
The journey of a wood gasifier electric generator from raw wood to humming electricity is a blend of chemistry, engineering, and a bit of grit, and it doesn’t stop with the basics—there’s more to unpack in how each piece comes together. Inside the gasifier, the process hinges on maintaining just the right conditions. The wood or biomass is typically fed into a hopper at the top, where it gradually descends into the reaction zone. This zone is where the heat—often kickstarted by a small fire or external burner—works its magic, reaching temperatures between 800°C and 1,200°C (1,472°F to 2,192°F) in the hottest spots. The limited oxygen, carefully controlled by air inlets, ensures the wood doesn’t burst into full flame. Instead, it smolders and decomposes. Pyrolysis kicks off first, vaporizing the wood’s volatile components into gases like carbon monoxide and hydrogen. Then, partial combustion reacts some of the carbon with oxygen to form more carbon monoxide, while any water in the wood can break down into hydrogen and oxygen in the heat, adding to the mix. What’s left—charcoal and ash—either burns further to keep the reaction going or drops out as waste.
The design of the gasifier matters here. Take a stratified downdraft gasifier, a popular type: the wood moves downward through distinct zones—drying at the top, pyrolysis in the middle, and combustion and reduction at the bottom—where the gases are pulled through a bed of hot charcoal, refining them before they exit. This setup cuts down on tar compared to an updraft design, where gases rise through unburned fuel and pick up more impurities. Either way, the syngas leaves the gasifier through an outlet, but it’s still a rough brew—hot, smoky, and laden with stuff you don’t want in an engine.
That’s where the cleanup comes in, and it’s more involved than it sounds. The syngas might hit a cyclone first, a spinning chamber that flings heavier particulates like ash and soot to the walls, letting cleaner gas pass through. Then it’s onto filters—coarse ones like gravel or wood shavings to catch bigger bits, followed by finer ones like cloth or even oil-soaked pads to snag the smallest particles. Cooling is critical too; the gas might flow through a radiator or a coiled pipe submerged in water, dropping its temperature from hundreds of degrees to something the engine can handle, usually below 100°C (212°F). This cooling also condenses tar and moisture into liquids that can be drained off, though some stubborn tar might need a dedicated scrubber—a wet filter that bubbles the gas through water or oil—to trap it. By the end, the syngas is lean but clean, with an energy content of about 4-6 megajoules per cubic meter, roughly half that of natural gas but plenty to work with.
Now, feeding this gas into the engine takes some finesse. A standard gasoline engine might need a mixing valve swapped in for the carburetor, blending syngas with air at a ratio of about 1:1, since it’s less energy-dense than liquid fuel. Diesel engines can run on it too, though they often need a small amount of diesel injected to ignite the mix, a setup called dual-fuel mode. The engine fires up, and the syngas combusts in the cylinders, pushing pistons that spin the crankshaft, which drives the generator—usually an alternator producing AC power. Output varies: a small 10 kW system might need 20-30 pounds of wood per hour, depending on the wood’s quality and the gasifier’s efficiency. Meanwhile, the engine’s exhaust and heat—hundreds of degrees worth—can be redirected through a heat exchanger, warming water or air for practical use, pushing the system’s total efficiency toward 70-80% if you’re capturing both power and heat.
It’s a dynamic process, full of variables. Too much moisture in the wood, and the gas gets watery and weak; too little air flow, and the gasifier stalls; too much tar slipping through, and the engine chokes. Tuning it requires constant attention—adjusting air valves, checking filters, monitoring the flame’s color (a blue tint means clean gas)—but when it’s dialed in, you’ve got a steady stream of electricity from something as basic as a woodpile, a testament to ingenuity and resourcefulness.
The operation of a wood gasifier electric generator is a dance of precision and adaptation, with each step building on the last to keep the system churning out power. Once the gasification process is underway, the gasifier becomes a living thing of sorts, reacting to every tweak and shift. The wood or biomass, steadily consumed in that low-oxygen chamber, doesn’t just vanish—it transforms through overlapping reactions that are both art and science. As the heat ramps up, the wood first dries out, releasing steam that can either dilute the syngas or, if conditions are right, crack into extra hydrogen. Then pyrolysis takes over, charring the wood into a volatile stew of gases, while the glowing charcoal below reacts with carbon dioxide and steam in a process called reduction, turning them into more carbon monoxide and hydrogen. This isn’t a clean split—some methane sneaks in, along with nitrogen from the air, diluting the mix—but the goal is a steady flow of syngas, pulsing out of the gasifier like a heartbeat.
The type of gasifier shapes this flow. In a downdraft model, the air is sucked downward through the fuel bed, passing through the hottest zone last, which burns off some of the tar before the gas exits—a rugged, practical choice for engine use. An updraft gasifier flips this, pulling air up through the wood, producing more tar but also more gas, better suited for heating than power generation. There’s even the crossdraft design, where air cuts sideways through the fuel, simpler but less efficient. Whatever the build, the gasifier’s output depends on the fuel: hardwood like oak or maple gives a dense, energy-rich gas, while softwoods like pine might load it with sticky resins that complicate cleanup. Moisture’s the real wildcard—above 20%, and the gas turns weak and wet, forcing the system to work harder for less.
That raw syngas, steaming out at 300°C to 500°C (572°F to 932°F), demands a serious cleanup before it’s engine-worthy. The cooling and filtering stretch can feel like a gauntlet. After the cyclone knocks out the heavy ash, the gas might hit a heat exchanger—say, a car radiator rigged with a fan—dropping its temperature fast to condense out water and tar. Some setups get crafty, running the gas through a pipe coiled in a barrel of cold water, or even a misting chamber where water droplets snatch tar mid-flight. Filters come next, layered for precision: coarse gravel or biochar catches the big stuff, then finer mesh or fabric grabs the dust, and sometimes a final oil bath traps the last wisps of tar. A well-tuned system might even use the charcoal left in the gasifier as a filter, letting the gas percolate through it to burn off extra impurities. By the time it’s done, the syngas is cool—maybe 40°C (104°F)—and lean, with an energy punch of 4-6 MJ/m³, ready to roll.
Getting that gas into the engine is where the rubber meets the road. The syngas flows into a mixing valve or manifold, blending with air at a near-even split, since it burns slower and cooler than gasoline. A gasoline engine might need its timing advanced to spark earlier, compensating for the gas’s sluggish combustion, while a diesel might sip a 10-20% diesel pilot to ignite the mix. The engine roars to life—though it might cough at first if the gas isn’t steady—and spins the generator, cranking out watts. A 5-horsepower engine paired with a 3 kW alternator might hum along on 10-15 pounds of wood per hour, enough for lights and a fridge in a pinch. The exhaust, still hot at 200°C (392°F) or more, carries waste gases like carbon dioxide and nitrogen, but a smart setup pipes it through a heat exchanger—maybe copper tubing wrapped around a water tank—capturing heat for a shower or radiator.
The whole rig is a feedback loop. If the gasifier starves for air, the flame dims and power drops; if the filters clog, backpressure stalls the flow. You’re watching gauges, listening to the engine’s rhythm, tweaking valves to keep the gas blue and clean. A small system might churn out 1-10 kW, enough for a homestead, while bigger ones hit 50 kW or more, demanding industrial-grade plumbing and automation. It’s not set-and-forget—wood needs loading, ash needs dumping, filters need swapping—but when it’s dialed in, it’s a marvel, spinning electricity from logs with a raw, mechanical soul.
The wood gasifier electric generator’s operation is a relentless interplay of heat, chemistry, and mechanical grit, and as the process hums along, every component has to pull its weight to keep the power flowing. Inside the gasifier, the transformation of wood into syngas is a dynamic beast, shifting with every handful of fuel tossed in. The reaction zones—drying, pyrolysis, combustion, and reduction—don’t sit still; they overlap and migrate as the wood burns down. At the top, where fresh chips or logs enter, heat rising from below drives off moisture, turning it into steam that either escapes or gets sucked into the mix. Below, pyrolysis chars the wood into a volatile fog of gases, releasing carbon monoxide and hydrogen as the cellulose and lignin break apart. Deeper still, the combustion zone glows red-hot, where a trickle of air feeds just enough oxygen to burn some of the carbon into carbon dioxide, which then reacts with the surrounding charcoal in the reduction zone, flipping it back to carbon monoxide. It’s a messy cycle—some wood turns to ash, some to tarry vapor—but when it’s running right, the syngas streams out steady, a hazy lifeline to the engine.
The gasifier’s quirks depend on its build and the fuel. A downdraft unit, with its downward air pull, keeps tar low by dragging the gas through the hottest coals, cracking it into simpler compounds—ideal for a generator setup. An updraft model, pulling air upward, churns out more gas but loads it with tar and water, better for a furnace than a piston. The fuel’s character matters too: dense oak or hickory packs more energy per pound, yielding a richer gas, while sappy pine or green wood spits out extra tar and smoke, gumming things up. Temperature’s the puppet master—too low (below 700°C or 1,292°F), and the gas turns weak; too high, and you’re just torching the wood to ash. A seasoned operator watches the exhaust flame—blue means clean combustion, yellow flags tar—and tweaks the air vents or fuel feed to keep it in the sweet spot.
That syngas, roaring out of the gasifier at 400°C to 600°C (752°F to 1,112°F), is a hot mess—literally. Cooling and cleaning it is a marathon, not a sprint. First, it might hit a cyclone, a spinning steel drum where centrifugal force slings ash and soot to the sides, letting cleaner gas slip through. Then it’s into a cooling rig—maybe a salvaged car radiator with fins shedding heat, or a homemade pipe coil dunked in a creek-fed tank—dropping the temperature to 50°C (122°F) or lower. This condenses water and tar into sticky runoff, drained off through a trap. Filters follow, staged like a gauntlet: coarse sand or biochar snags the chunky bits, a wad of steel wool or burlap grabs the dust, and a final wet scrubber—gas bubbled through a shallow oil pool—traps the last tar wisps. Some setups get fancy, recycling the gasifier’s own charcoal as a filter bed, letting it char-broil any lingering impurities. The result? A cool, dry syngas with a heating value of 4-6 MJ/m³—less punchy than propane, but enough to drive an engine.
Feeding that gas to the engine is where theory meets reality. The syngas pipes into a mixer—often a hacked-together valve bolted where a carburetor once sat—blending with air at a tight 1:1 ratio, tuned for its slow burn. A gasoline engine might need its spark timing bumped forward 10-15 degrees, sparking earlier to catch the gas’s lazy flame, while a diesel might chug along on 80% syngas and a 20% diesel squirt to light it off. The engine fires—sometimes with a sputter if the gas wavers—and pistons hammer away, spinning the generator. A 10-horsepower rig might crank out 5-7 kW, burning 20-25 pounds of wood an hour, enough for a small shop or cabin. The exhaust, still scalding at 250°C (482°F), can be routed through a heat exchanger—say, a steel box with water pipes—harvesting warmth for a radiator or hot tub, pushing total efficiency past 75% if you’re thorough.
It’s a system that fights to stay alive. A wet wood batch can drown the gas in steam, clogging filters; a lazy draft can stall the flow, choking the engine. You’re on constant watch—tapping ash out, swapping filters, sniffing for tar’s telltale stink. A small setup might light a few bulbs and a pump; a beefy one could run a sawmill. It’s raw, temperamental, and alive—electricity wrung from wood with sweat and steel.
EMS Power Machines
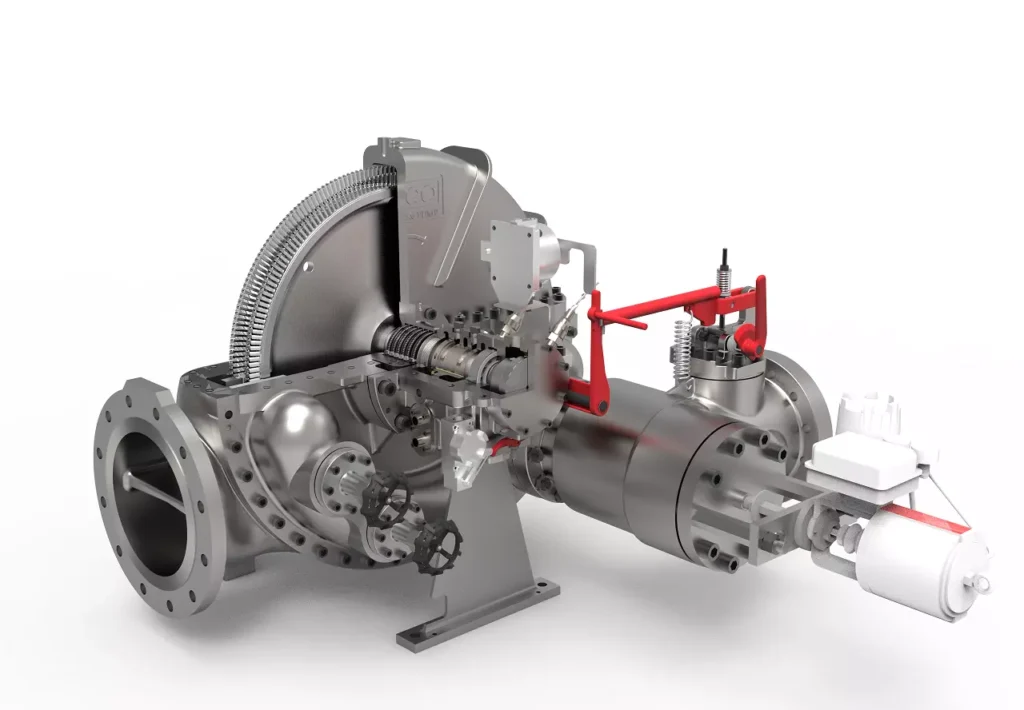
We design, manufacture and assembly Power Machines such as – diesel generators, electric motors, vibration motors, pumps, steam engines and steam turbines
EMS Power Machines is a global power engineering company, one of the five world leaders in the industry in terms of installed equipment. The companies included in the company have been operating in the energy market for more than 60 years.
EMS Power Machines manufactures steam turbines, gas turbines, hydroelectric turbines, generators, and other power equipment for thermal, nuclear, and hydroelectric power plants, as well as for various industries, transport, and marine energy.
EMS Power Machines is a major player in the global power industry, and its equipment is used in power plants all over the world. The company has a strong track record of innovation, and it is constantly developing new and improved technologies.
Here are some examples of Power Machines’ products and services:
- Steam turbines for thermal and nuclear power plants
- Gas turbines for combined cycle power plants and industrial applications
- Hydroelectric turbines for hydroelectric power plants
- Generators for all types of power plants
- Boilers for thermal power plants
- Condensers for thermal power plants
- Reheaters for thermal power plants
- Air preheaters for thermal power plants
- Feedwater pumps for thermal power plants
- Control systems for power plants
- Maintenance and repair services for power plants
EMS Power Machines is committed to providing its customers with high-quality products and services. The company has a strong reputation for reliability and innovation. Power Machines is a leading provider of power equipment and services, and it plays a vital role in the global power industry.
EMS Power Machines, which began in 1961 as a small factory of electric motors, has become a leading global supplier of electronic products for different segments. The search for excellence has resulted in the diversification of the business, adding to the electric motors products which provide from power generation to more efficient means of use.