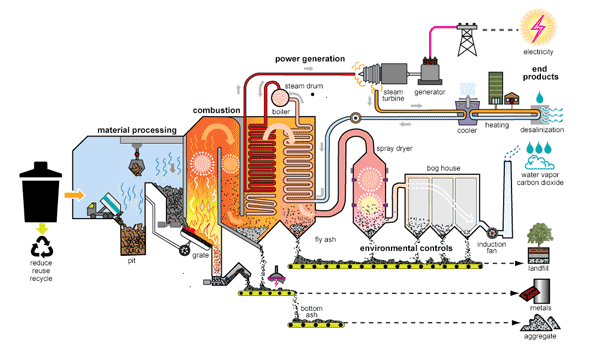
What is Waste Heat Boiler: Waste heat recovery (WHR) refers to the process of capturing and utilizing heat that would otherwise be lost to the environment, typically in industrial processes, power generation, and HVAC systems. There are several types of waste heat recovery systems, and they can be categorized based on the method and application:
1. Heat Recovery Steam Generators (HRSG)
- Description: These are used in combined cycle power plants and other applications where exhaust gases from turbines or engines are used to generate steam. The steam produced can be used for power generation or industrial heating.
- Applications: Combined-cycle power plants, cogeneration systems, industrial processes.
2. Heat Exchangers
- Description: Heat exchangers transfer heat from a hot fluid (e.g., exhaust gases) to a cooler fluid (e.g., water or air) without mixing them. Various types include shell-and-tube, plate, and air-cooled heat exchangers.
- Applications: Industrial processes, chemical plants, HVAC systems.
3. Regenerative Heat Recovery Systems
- Description: These systems use a heat storage medium, such as ceramic or metal, to absorb heat from exhaust gases and then release it to the incoming cold fluid or air. These systems are commonly used in furnaces, kilns, and industrial boilers.
- Applications: Kilns, furnaces, industrial heating processes.
4. Thermoelectric Generators (TEGs)
- Description: TEGs convert temperature differences directly into electrical energy using the Seebeck effect. While less efficient than steam-based recovery methods, they are useful for small-scale applications where other systems are impractical.
- Applications: Small-scale industrial processes, remote power generation.
5. Organic Rankine Cycle (ORC)
- Description: This process uses organic fluids with a low boiling point, which allows for heat recovery from lower temperature sources. The organic fluid is vaporized, and the vapor is used to drive a turbine that generates electricity.
- Applications: Geothermal power plants, industrial waste heat recovery, biomass power plants.
6. Combined Heat and Power (CHP) Systems
- Description: Also known as cogeneration, these systems simultaneously generate electricity and useful heat. Heat that would otherwise be wasted in the power generation process is captured and used for space heating, industrial processes, or hot water production.
- Applications: Hospitals, universities, industrial sites, district heating.
7. Heat Pumps
- Description: Heat pumps can recover waste heat from one source and transfer it to another, such as from exhaust air to space heating. In some cases, waste heat can also be upgraded to a higher temperature.
- Applications: HVAC systems, industrial heating, district heating.
8. Latent Heat Recovery
- Description: This method involves capturing the latent heat from the phase change of materials, such as water vapor condensing into liquid or solidifying. This is commonly seen in air conditioning and refrigeration applications.
- Applications: HVAC, refrigeration, industrial cooling systems.
9. Flue Gas Heat Recovery
- Description: This involves recovering heat from the exhaust gases of combustion processes. The heat is often used to preheat combustion air or water for other processes, thereby improving overall system efficiency.
- Applications: Boilers, furnaces, industrial heating.
10. Solar-Assisted Waste Heat Recovery
- Description: This combines solar energy with waste heat recovery by using solar collectors to provide additional heat to processes or preheating fluids, improving the overall efficiency of the system.
- Applications: Industrial processes, residential and commercial heating.
Each method has its own advantages depending on the type of waste heat available, the temperature, and the desired outcome (such as electricity generation, heating, or process improvement). The effectiveness of these systems can be improved with proper integration into existing processes.
Waste heat recovery (WHR) refers to capturing and reusing heat that would otherwise be lost in various industrial, power generation, and HVAC systems. Several methods are used to recover and utilize waste heat.
Heat Recovery Steam Generators (HRSG) are commonly used in combined cycle power plants. These systems use exhaust gases from turbines to generate steam, which can be used for power generation or industrial heating. Heat exchangers, which transfer heat from hot fluids to cooler ones without mixing them, are another widely used method. They are employed in industrial processes, chemical plants, and HVAC systems.
Regenerative heat recovery systems store heat in a medium like ceramic or metal, which is then released to a colder fluid or air. These are often found in furnaces, kilns, and industrial boilers. Thermoelectric generators (TEGs) convert temperature differences directly into electricity, but they are typically used for small-scale applications due to their lower efficiency compared to steam-based methods.
The Organic Rankine Cycle (ORC) is a process where an organic fluid with a low boiling point is vaporized by waste heat and used to drive a turbine that generates electricity. This method is particularly useful for low-temperature waste heat recovery, such as from geothermal plants or biomass systems. Combined Heat and Power (CHP) systems, also known as cogeneration, generate both electricity and useful heat. The waste heat from power generation is harnessed for space heating, industrial processes, or hot water production, improving system efficiency.
Heat pumps are another effective way to recover waste heat by transferring it from one source, like exhaust air, to another, such as for space heating. In some cases, waste heat can be upgraded to a higher temperature for further use. Latent heat recovery involves capturing heat from phase changes, such as when water vapor condenses into liquid, often used in HVAC and refrigeration systems. Flue gas heat recovery involves capturing heat from combustion exhaust gases and using it to preheat combustion air or water, improving overall system efficiency.
Lastly, solar-assisted waste heat recovery combines solar energy with waste heat recovery systems, using solar collectors to provide additional heat for processes or fluid preheating. This method can improve the overall efficiency of a heating system in industrial processes, residential, or commercial settings. Each of these methods offers unique advantages depending on the temperature and type of waste heat, allowing for better integration into various applications to enhance efficiency
Waste heat recovery can also be integrated with advanced technologies to optimize energy use across different systems. One such method involves integrating multiple waste heat recovery systems to maximize efficiency. For example, a combined cycle power plant can pair an HRSG with a heat pump or ORC system to further harness low-grade waste heat, improving overall energy recovery from exhaust gases or cooling systems.
Another growing trend is the use of advanced materials and nanotechnology to enhance the performance of waste heat recovery systems. For instance, materials with higher thermal conductivity or advanced coatings can be applied to heat exchangers, improving their heat transfer rates. Similarly, nanofluids—fluids containing nanometer-sized particles—are being studied for use in heat exchangers to improve heat transfer and reduce the size and cost of heat recovery equipment.
In industrial settings, the integration of waste heat recovery with automated systems and advanced controls can optimize the timing and temperature of heat recovery, ensuring that energy is harvested when it’s most beneficial. Digital technologies such as IoT sensors and machine learning algorithms can track real-time conditions and adjust the recovery system’s performance based on factors like plant load, ambient temperature, and process demand, maximizing overall energy savings.
Furthermore, the concept of district energy systems is gaining traction, particularly in urban areas. These systems can centralize heat recovery from multiple sources (like industrial facilities, power plants, or even waste incineration) and distribute recovered heat to a network of buildings for space heating, hot water, and even industrial applications. By using heat recovered from diverse sources, district energy systems can significantly reduce reliance on conventional energy sources and improve the overall energy efficiency of communities.
The economic benefits of waste heat recovery are also a strong driver for adoption. Businesses and industries can reduce their operational costs by recovering waste heat for internal use or converting it into electricity. Additionally, regulations and policies in many regions are encouraging or even mandating the use of energy recovery technologies, offering incentives such as tax credits, grants, or regulatory benefits to companies that adopt waste heat recovery systems.
In the long term, the global push toward sustainability and carbon reduction will continue to promote the growth of waste heat recovery technologies. As industries look for ways to reduce their environmental impact, waste heat recovery not only makes good economic sense but also aligns with global efforts to minimize energy consumption and greenhouse gas emissions. These systems will become even more crucial as the demand for clean and efficient energy solutions grows, offering both environmental and financial benefits.
As the demand for sustainable solutions grows, waste heat recovery systems are becoming more critical in industries such as manufacturing, automotive, and even residential sectors. In manufacturing, for example, high-temperature processes like steel production, cement manufacturing, and glass production are significant sources of waste heat. These industries are increasingly integrating waste heat recovery technologies to improve energy efficiency and reduce carbon emissions. The captured waste heat can be repurposed for preheating combustion air, powering turbines, or even producing electricity in some cases.
In the automotive industry, waste heat recovery systems are being integrated into vehicles to enhance fuel efficiency. Technologies such as thermoelectric generators (TEGs) and turbo-compounding are used to convert excess engine heat into electricity, which can be used to power electrical systems or recharge batteries in hybrid and electric vehicles. As the automotive sector pushes toward more sustainable transportation solutions, waste heat recovery plays a key role in improving overall energy utilization.
Residential applications are also benefiting from waste heat recovery systems, particularly in the form of heat pumps and energy-efficient heating systems. In buildings, waste heat recovery can be used to preheat water or air, reducing the need for additional energy inputs. This is especially beneficial in areas where heating demands are high, as it allows homeowners and businesses to reduce their energy consumption while maintaining comfortable indoor temperatures.
Further, the rise of green building standards and energy codes is driving the adoption of waste heat recovery technologies in new construction. Buildings that incorporate integrated heat recovery systems can achieve higher energy efficiency ratings and lower operating costs, contributing to sustainability goals and helping building owners meet stringent environmental standards. This trend is especially prevalent in regions with strong environmental regulations, where waste heat recovery is becoming an essential component of building designs.
The role of waste heat recovery in the energy transition is also worth noting. As countries transition to renewable energy sources, waste heat recovery helps to bridge the gap by utilizing low-grade heat that would otherwise go to waste. This is particularly important in the context of fluctuating renewable energy generation, such as solar and wind power, which are intermittent. Waste heat recovery systems provide a steady, reliable source of energy, making it easier to integrate renewable energy into existing grids and reducing dependency on fossil fuels.
The future of waste heat recovery looks promising as new innovations continue to emerge. One exciting development is the potential for utilizing waste heat in more unconventional ways, such as in district cooling systems, where recovered heat is used to drive cooling processes in urban areas. Additionally, as digital technologies continue to evolve, the optimization of waste heat recovery systems through artificial intelligence (AI) and predictive analytics could further improve efficiency, reliability, and cost-effectiveness.
Lastly, global collaboration and research are helping to push the boundaries of waste heat recovery technologies. International standards, government policies, and public-private partnerships are accelerating the adoption of waste heat recovery in various industries, making it a vital component of global efforts to combat climate change and reduce energy consumption. With continuous advancements, the ability to recover and reuse waste heat will become an increasingly important strategy for building a more energy-efficient and sustainable world.
Plate Heat Exchanger
A plate heat exchanger (PHE) is a type of heat exchanger that uses multiple thin, corrugated metal plates to transfer heat between two fluids. The fluids are separated by the plates, and heat is transferred from one fluid to another through the plate material. Plate heat exchangers are widely used in various industries for efficient heat transfer and compact design.
Structure and Working Principle
The plate heat exchanger consists of a series of metal plates that are stacked together, with each plate having a series of flow channels that allow the fluids to pass through. The plates are usually made of stainless steel or other corrosion-resistant materials. The plates are designed with a specific pattern of corrugations (raised ridges) to enhance heat transfer by increasing the surface area and promoting turbulence in the fluids, which improves heat exchange efficiency.
The fluids are routed through alternate channels, where one fluid flows through one side of the plate, and the other fluid flows through the opposite side of the plate. This creates a counter-current flow arrangement, which is ideal for maximizing heat transfer.
Advantages
- Compact Design: PHEs have a compact and modular design, making them ideal for installations where space is limited. The stacked plate configuration allows for a high heat transfer surface area in a small footprint.
- Efficient Heat Transfer: The corrugated plates create turbulence in the fluid flow, which increases the heat transfer rate. The counter-flow design further improves the efficiency of heat exchange.
- Flexible Capacity: PHEs are modular, so additional plates can be added or removed to adjust the heat transfer capacity as needed. This flexibility allows for customization based on specific process requirements.
- Easy Maintenance: Plate heat exchangers are easier to clean and maintain compared to other types of heat exchangers, like shell-and-tube designs. The plates can be separated for inspection, cleaning, or replacement.
- Energy Efficiency: Due to their high heat transfer efficiency, plate heat exchangers help reduce energy consumption in industrial processes. They are particularly useful in applications where energy savings are a priority.
Applications
Plate heat exchangers are used in a wide range of applications across different industries, including:
- HVAC Systems: They are used in heating, ventilation, and air conditioning systems to transfer heat between air and water or other fluids.
- Food and Beverage: PHEs are commonly used in pasteurization, cooling, and heating processes in the food and beverage industry, where hygiene and efficiency are critical.
- Chemical and Pharmaceutical: They are employed in chemical processes for heating or cooling of reactive fluids, often where precise temperature control is necessary.
- Power Generation: Plate heat exchangers are used in power plants to recover waste heat or to transfer heat between different fluid loops in the system.
- Waste Heat Recovery: PHEs can be used to recover waste heat from exhaust gases or industrial processes and transfer it to another fluid for reuse, such as in district heating systems or preheating water.
Limitations
Despite their many advantages, plate heat exchangers have some limitations:
- Pressure Drop: Depending on the design and the flow rate of fluids, plate heat exchangers can experience significant pressure drop, especially when the flow is not optimized.
- Size Limitations: While they are compact, plate heat exchangers are generally limited in terms of the maximum capacity that can be achieved with a single unit. For very large heat transfer requirements, multiple units may need to be used.
- Leakage Risk: The sealing gaskets between the plates can sometimes be vulnerable to leakage, especially if the pressure or temperature conditions exceed the design specifications. Regular maintenance and monitoring are required to avoid this issue.
Conclusion
Plate heat exchangers are highly efficient and versatile heat transfer devices suitable for a wide range of applications. Their compact size, high heat transfer efficiency, and flexibility make them an attractive choice for industries that require heat exchange systems that can handle a variety of fluids and conditions. However, they do require proper maintenance and careful design to avoid issues related to pressure drop and leakage.
Plate heat exchangers (PHEs) are widely appreciated for their compact design and high heat transfer efficiency. They are made up of a series of metal plates stacked together with alternating channels for two fluids to flow through. The plates are often corrugated to increase surface area and create turbulence, which promotes better heat exchange between the fluids. The heat transfer occurs as the fluids flow on opposite sides of the plates, with one fluid absorbing heat and the other releasing it, typically in a counter-flow arrangement.
The modular design of plate heat exchangers allows for easy customization. Plates can be added or removed to increase or decrease the heat transfer capacity, making them highly flexible for varying process requirements. This modularity also allows for easier maintenance compared to other heat exchangers like shell-and-tube types, as the plates can be separated for cleaning, inspection, or replacement. Their high thermal efficiency makes them ideal for applications where space and energy savings are critical, such as in industrial processes, HVAC systems, and food production.
In industries such as food and beverage processing, plate heat exchangers are used for pasteurization and cooling because of their ability to handle both heat-sensitive and high-temperature fluids effectively. In chemical and pharmaceutical industries, they are used for controlling the temperature of reactive fluids. They also find applications in power generation for waste heat recovery, helping to optimize energy use by reusing heat that would otherwise be lost.
One of the key benefits of PHEs is their energy efficiency. By increasing the heat transfer surface area and improving flow dynamics, they help reduce energy consumption, which is particularly important for processes that require significant heat exchange. Additionally, because they are modular and customizable, they can be adapted to different heat transfer capacities, making them versatile across many different systems.
However, plate heat exchangers do have some limitations. They can experience a higher pressure drop compared to other designs, particularly when flow rates are not well-optimized. For very large applications, multiple units may be required, which can increase system complexity and space requirements. Also, the gaskets that seal the plates can sometimes fail under extreme conditions, leading to leaks, so regular maintenance and careful monitoring are necessary.
Overall, plate heat exchangers offer a highly efficient, space-saving solution for heat exchange needs across a variety of industries. Their ability to be easily customized, their efficiency in transferring heat, and the ease of maintenance make them a preferred choice in many settings. Nonetheless, their limitations in terms of pressure drop and the risk of leakage mean they need to be designed and maintained carefully to ensure optimal performance.
As industries continue to seek more efficient and sustainable solutions, plate heat exchangers (PHEs) play an increasingly vital role in enhancing energy efficiency and reducing operational costs. Their ability to recover and reuse heat helps to reduce the overall energy consumption of a system, which is especially crucial in energy-intensive industries such as petrochemical, steel manufacturing, and paper mills. The versatility of PHEs means they can handle a wide range of fluids, from liquids and gases to even highly viscous materials, without compromising on efficiency.
One of the key advantages of PHEs in many industries is their flexibility in terms of design. The heat transfer performance can be adjusted to suit the specific requirements of the application by varying the number of plates or by using different types of plate corrugation patterns. This allows them to be tailored for both low- and high-temperature applications, from cooling hot process fluids to heating cold fluids in closed-loop systems. Their design also enables them to recover waste heat from exhaust gases or cooling systems, providing a cost-effective solution for reducing waste and improving overall system performance.
In addition to their use in industrial processes, plate heat exchangers are increasingly being used in district heating and cooling systems. In these applications, they help to efficiently transfer heat between different parts of a district heating network, where waste heat from one building or industrial process can be transferred to another. This kind of application is becoming more common as cities and municipalities look for ways to reduce energy consumption and lower carbon footprints.
The integration of PHEs with renewable energy sources is another growing trend. For example, they are used in solar thermal systems to transfer heat collected from solar collectors to water or air, which can then be used for space heating or industrial processes. The same principles are applied to geothermal energy systems, where heat exchangers are used to transfer heat from the ground to fluids circulating in the system. This integration enhances the overall sustainability and efficiency of the systems.
While plate heat exchangers are very effective, their performance can be affected by factors such as fouling and scaling. Fouling occurs when particles or other contaminants build up on the heat transfer surfaces, reducing the efficiency of heat exchange. In industries where fluids contain high levels of particulates or where temperatures fluctuate significantly, fouling can be a persistent issue. Therefore, regular cleaning and maintenance are critical to ensuring that the PHE continues to operate at optimal efficiency.
Additionally, the design of the plates and the materials used can impact the long-term reliability and performance of plate heat exchangers. Stainless steel is commonly used because of its resistance to corrosion and high-temperature stability. However, for certain aggressive fluids or extreme conditions, other materials, such as titanium or special alloys, may be necessary to prevent corrosion or damage.
As technology advances, digital monitoring and predictive maintenance techniques are being applied to plate heat exchangers. Sensors and IoT devices can track temperature, pressure, and flow rates in real time, providing operators with valuable data on system performance. This data can be used to predict maintenance needs before issues arise, reducing downtime and improving overall system reliability. Such integration of smart technologies is transforming the way industries approach heat exchanger maintenance, helping to extend the life of equipment and further enhance operational efficiency.
Ultimately, plate heat exchangers offer significant advantages in terms of energy savings, space efficiency, and versatility across a broad range of applications. With continuous improvements in materials, design, and monitoring technologies, PHEs are likely to play an even more prominent role in helping industries reduce their energy consumption, minimize environmental impact, and improve operational performance in the years to come.
As the need for energy efficiency continues to grow across all sectors, the role of plate heat exchangers (PHEs) in industrial, commercial, and even residential applications becomes increasingly essential. In addition to traditional uses in industries like food processing, HVAC, and power generation, PHEs are also being integrated into more specialized areas such as heat recovery from waste streams, climate control in data centers, and even in the emerging field of thermal energy storage.
In the field of thermal energy storage, PHEs are being used to facilitate the transfer of heat to and from storage media, allowing heat to be stored during off-peak hours and used when demand is higher. This can be especially beneficial in renewable energy systems like solar or wind, which produce variable outputs. By using PHEs to store excess heat produced during periods of high renewable generation, energy can be more effectively utilized when renewable sources are not available, contributing to a more stable and sustainable energy supply.
The integration of plate heat exchangers with industrial heat recovery systems is also an area of growing interest. In many industries, large amounts of heat are wasted in the form of exhaust gases or cooling water. By capturing and transferring this waste heat to useful processes, PHEs can help improve energy efficiency while reducing operational costs. For example, in large chemical plants or oil refineries, waste heat from distillation towers or furnaces can be recovered using PHEs and directed toward preheating fluids or generating steam for other parts of the plant. This approach reduces the overall fuel consumption and improves the bottom line.
For data centers, which consume significant amounts of energy to keep their servers cool, PHEs are becoming a key part of energy-efficient cooling systems. In these facilities, the heat generated by servers is captured using PHEs and can be redirected for heating purposes in other areas of the building or even to nearby facilities. This not only helps reduce cooling energy consumption but also provides a way to repurpose the waste heat for other beneficial uses, contributing to sustainability goals.
In district cooling systems, plate heat exchangers are used to transfer heat from one building or industrial facility to another in centralized systems. This allows multiple buildings in an urban area to share the same source of cooling, improving efficiency and reducing energy consumption. Similarly, in district heating systems, PHEs are used to capture waste heat from industrial processes or even urban waste-to-energy facilities, transferring it to a district heating loop for residential or commercial use.
The demand for green buildings and sustainable infrastructure has also led to increased use of plate heat exchangers in building systems. PHEs are integral components in modern HVAC systems, where they help to efficiently manage the temperature and airflow in buildings, reducing the need for additional heating or cooling systems. In this way, they contribute to the overall energy efficiency of buildings, helping meet sustainability standards like LEED (Leadership in Energy and Environmental Design) and BREEAM (Building Research Establishment Environmental Assessment Method).
Innovative designs are pushing the boundaries of plate heat exchangers even further. For instance, flexible plate heat exchangers are being developed for use in mobile applications or in places where space is at a premium. These systems can be folded or bent, offering more versatility in installation. Additionally, double-wall plate heat exchangers are being used to ensure that fluids do not mix in the event of a gasket failure, which is particularly important when handling hazardous fluids.
Finally, ongoing research is focused on improving the material properties of the plates themselves. Traditional materials such as stainless steel are commonly used, but for more specialized applications, materials like titanium or nickel alloys may be required to resist corrosion or withstand higher temperatures. New coatings or surface treatments are also being developed to further enhance the thermal conductivity and durability of the plates, ensuring longer service life and more efficient heat transfer.
As industries and technologies continue to evolve, the use of plate heat exchangers is expected to expand, providing ever more efficient ways to manage heat across a variety of applications. Their adaptability, high performance, and energy-saving capabilities make them an essential part of the global effort to optimize energy use, reduce waste, and build a more sustainable future.
What is Waste Heat Boiler
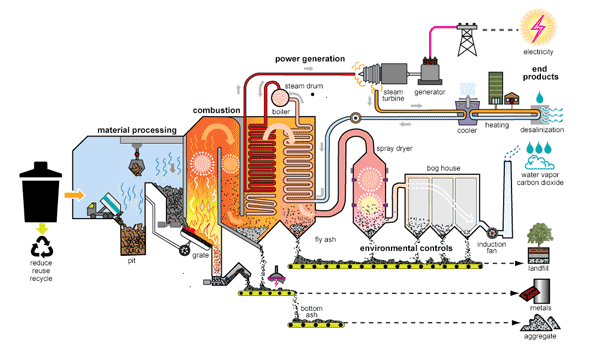
A waste heat boiler is a type of heat exchanger used to capture and recover heat from exhaust gases or other waste heat sources in industrial processes, combustion systems, or power plants. The purpose of a waste heat boiler is to utilize this otherwise lost heat to produce steam or hot water, which can then be used for various applications, such as power generation, heating, or as part of a process in the plant.
Working Principle
A waste heat boiler operates by channeling hot gases from a combustion process, industrial system, or exhaust stream through the boiler system. These gases pass over or through heat exchange surfaces, such as tubes, plates, or coils, transferring their thermal energy to water or another fluid circulating through the system. The heated fluid (usually water) is then converted into steam, which can be used for electricity generation or other heating purposes. In some designs, the waste heat is used directly to heat process fluids or preheat combustion air, further improving energy efficiency.
The waste heat boiler typically operates at lower temperatures than conventional boilers, as it recovers heat from exhaust gases or flue gases, which are often at lower temperatures (compared to direct combustion). Depending on the design, the boiler can be equipped with additional features like economizers or superheaters to increase the temperature and pressure of the steam produced, making it suitable for different applications.
Types of Waste Heat Boilers
- Vertical Waste Heat Boilers: These are typically compact units used for smaller applications. They are designed to accommodate gases flowing vertically through the system, making them more efficient in compact spaces.
- Horizontal Waste Heat Boilers: These are used in larger-scale systems and allow for better heat transfer due to the increased surface area. They tend to have larger volumes, making them suitable for power plants or large industrial plants.
- Fire Tube Waste Heat Boilers: These boilers have tubes filled with water, and exhaust gases flow over the outside of the tubes, transferring heat to the water inside. These are more common in applications where the waste heat source has relatively lower temperatures.
- Water Tube Waste Heat Boilers: In these boilers, water circulates inside tubes, while hot gases flow around the tubes. This type is more efficient for high-temperature applications and is often used in large industrial processes and power plants.
Applications
Waste heat boilers are commonly used in industries where high-temperature exhaust gases are generated, including:
- Power Generation: In combined cycle power plants, waste heat from gas turbines is used to generate additional steam for a steam turbine, increasing overall efficiency.
- Cement and Steel Manufacturing: These industries produce large amounts of waste heat from kilns, furnaces, or other high-temperature processes, which can be captured and converted into steam for process heating or power generation.
- Petrochemical and Chemical Industries: Waste heat from reactors, distillation columns, and combustion processes can be used to produce steam or hot water, reducing the need for additional fuel.
- Refineries: In refineries, waste heat boilers are used to recover heat from various high-temperature processes such as cracking or distillation, contributing to energy savings and reducing the need for external energy sources.
- Marine Applications: On ships and offshore platforms, waste heat boilers are used to recover energy from engine exhaust or other systems, producing steam for auxiliary systems or reducing fuel consumption.
Benefits
- Energy Efficiency: The primary benefit of a waste heat boiler is that it helps recover energy from exhaust gases, reducing the need for additional fuel. This improves overall system efficiency and reduces operating costs.
- Reduced Environmental Impact: By capturing and utilizing waste heat, these boilers help lower greenhouse gas emissions and reduce the carbon footprint of industrial processes.
- Cost Savings: Recovering waste heat can lead to significant savings in energy consumption. In many cases, the steam or hot water produced can be used in other processes, further reducing the need for purchased fuel or electricity.
- Sustainability: Waste heat recovery contributes to more sustainable operations by reducing waste, improving energy use, and promoting cleaner production methods.
Limitations
While waste heat boilers offer many benefits, there are some challenges:
- Capital and Installation Costs: The initial cost of installing a waste heat recovery system can be significant, particularly for large-scale operations.
- Maintenance: Over time, the heat exchanger surfaces can accumulate soot, dust, or other contaminants, leading to a reduction in efficiency. Regular cleaning and maintenance are required to keep the system operating at peak efficiency.
- Temperature and Composition of Waste Heat: The effectiveness of a waste heat boiler depends on the temperature and composition of the exhaust gases. In some cases, the waste heat may not be hot enough to produce steam effectively, limiting the recovery potential.
Conclusion
A waste heat boiler is a valuable piece of equipment in any industrial setting that generates exhaust gases or waste heat. By recovering this otherwise lost energy, waste heat boilers can significantly enhance energy efficiency, reduce operating costs, and contribute to environmental sustainability. Their application spans a wide range of industries, from power generation to manufacturing, where they play a crucial role in reducing energy consumption and optimizing process efficiency.
A waste heat boiler is designed to harness and recover heat from exhaust gases or waste heat streams generated by industrial processes, combustion systems, or power plants. The recovered heat is typically used to generate steam or hot water, which can then be reused in various applications like power generation, process heating, or heating systems. This process of capturing waste heat helps reduce the overall energy consumption by making use of heat that would otherwise be lost to the atmosphere or surrounding environment.
These boilers work by directing hot exhaust gases or flue gases over heat exchange surfaces, such as tubes, coils, or plates, transferring their thermal energy to water or another circulating fluid. As the fluid absorbs heat, it is heated to the required temperature, converting water into steam, which can be used in steam turbines for power generation or in other processes requiring steam. In some systems, waste heat is also used to preheat incoming fluids, thereby reducing the amount of fuel needed to heat them to the required temperatures.
Waste heat boilers come in various designs, tailored to different applications and scales. In industries where high-temperature exhaust gases are produced, like cement or steel manufacturing, these systems can be quite large and robust, capable of handling and recovering significant amounts of waste heat. In smaller-scale applications, waste heat boilers can be more compact and integrated into processes with lower waste heat generation.
The benefits of waste heat boilers are substantial. They significantly improve energy efficiency by capturing heat that would otherwise be wasted. By reusing this heat, businesses can reduce the amount of fuel required for their processes, lowering operational costs and energy bills. This also contributes to a reduction in greenhouse gas emissions, supporting environmental sustainability goals by lessening the dependency on additional energy sources and cutting down on the overall carbon footprint of industrial operations. In industries like chemical processing, power generation, and refining, where heat is a byproduct of the core processes, the integration of waste heat boilers is an effective way to optimize energy use and improve the overall environmental impact of the operations.
However, waste heat recovery through boilers is not without its challenges. For one, the temperature and quality of the exhaust gases play a critical role in determining the effectiveness of the system. If the waste heat is at a relatively low temperature, it may not be efficient enough to produce significant amounts of steam, which can limit the potential for recovery. Additionally, while waste heat recovery systems can offer long-term energy savings, they can have high initial installation costs, particularly for larger-scale operations. Maintenance of these systems can also be demanding, as the heat exchange surfaces can accumulate soot, dust, or other contaminants over time, requiring regular cleaning and servicing to maintain efficiency.
Despite these challenges, the role of waste heat boilers is becoming more prominent as industries focus on reducing energy consumption and improving sustainability. Whether in large-scale industrial plants, power stations, or even marine applications, the recovery of waste heat is an essential strategy for minimizing energy use and reducing environmental impact. As technology advances and more efficient designs are developed, the ability to harness waste heat will continue to play a vital part in building energy-efficient and sustainable systems.
The use of waste heat boilers extends beyond just reducing operational costs and improving energy efficiency; they also contribute significantly to the overall sustainability of industrial processes. By integrating waste heat recovery systems, industries can lower their dependency on external energy sources, leading to reduced fuel consumption and a lower environmental footprint. This shift toward utilizing waste heat aligns with broader global initiatives aimed at combating climate change and reducing energy consumption.
In sectors such as power generation, where combined cycle plants are increasingly common, waste heat boilers recover heat from gas turbines and use it to produce additional steam for steam turbines, boosting the overall efficiency of the power plant. This combination of gas and steam turbines creates a more energy-efficient system that reduces the need for additional fuel and maximizes the use of the energy produced. In large industrial processes like cement manufacturing, where extremely high temperatures are used in kilns, waste heat boilers capture the heat from exhaust gases to produce steam that can be used elsewhere in the plant for process heating, thereby reducing fuel consumption.
In the petrochemical and chemical industries, where high temperatures are prevalent in reaction processes and distillation columns, waste heat boilers capture the heat from exhaust gases or flue gas stacks to generate steam for various uses, such as preheating feedwater or producing electricity. This integration not only reduces energy costs but also makes industrial operations more sustainable by minimizing waste heat emissions and recovering a portion of the energy that would otherwise be wasted.
Another important aspect of waste heat boilers is their role in district heating and cooling systems. By using waste heat from industrial processes or even from municipal waste-to-energy plants, waste heat boilers can provide a source of hot water or steam for district heating networks. These networks can distribute the recovered heat to nearby residential, commercial, and industrial buildings, significantly reducing the need for separate heating systems and improving the overall energy efficiency of a city or region. Similarly, waste heat boilers can be part of district cooling systems, where heat is recovered from industrial processes to cool buildings or processes in urban areas.
The integration of waste heat boilers into combined heat and power (CHP) systems has also become increasingly popular. CHP systems generate both electricity and useful heat from a single energy source, and waste heat boilers are an essential component in improving the overall efficiency of these systems. By using the waste heat from electricity generation processes to produce steam for heating, these systems can achieve efficiencies of 70% to 90%, much higher than traditional power generation methods that only convert a small percentage of energy into useful work. This combined approach not only reduces fuel consumption but also increases the utility of the energy produced.
As industries continue to innovate and push for greater efficiency, the technology behind waste heat boilers is also evolving. New materials and coatings are being developed to improve the durability and heat transfer performance of these systems. Advanced computational fluid dynamics (CFD) modeling is also being used to optimize the design and flow characteristics of waste heat boilers, allowing for better heat recovery and reduced energy losses. Additionally, the incorporation of digital technologies and sensors enables real-time monitoring and control of waste heat recovery systems, helping operators to ensure optimal performance and detect potential issues before they lead to system failure.
In applications where the waste heat is not sufficient to generate steam for power generation, innovations in hybrid systems are emerging. These systems combine waste heat recovery with renewable energy sources such as solar or geothermal energy, creating even more sustainable, energy-efficient solutions. By using renewable energy to supplement the waste heat, these systems can achieve higher efficiencies and further reduce environmental impact.
In summary, waste heat boilers are a crucial component of modern industrial and energy systems. They allow industries to recover and repurpose heat that would otherwise be wasted, leading to significant improvements in energy efficiency and reductions in energy costs. The benefits extend beyond just financial savings—waste heat boilers also contribute to environmental sustainability by reducing the reliance on external energy sources and minimizing greenhouse gas emissions. As the technology continues to evolve and integrate with other energy-efficient systems, waste heat recovery will play an increasingly important role in achieving global energy goals and building more sustainable industrial processes.
How to Reduce Steam Consumption in Plant
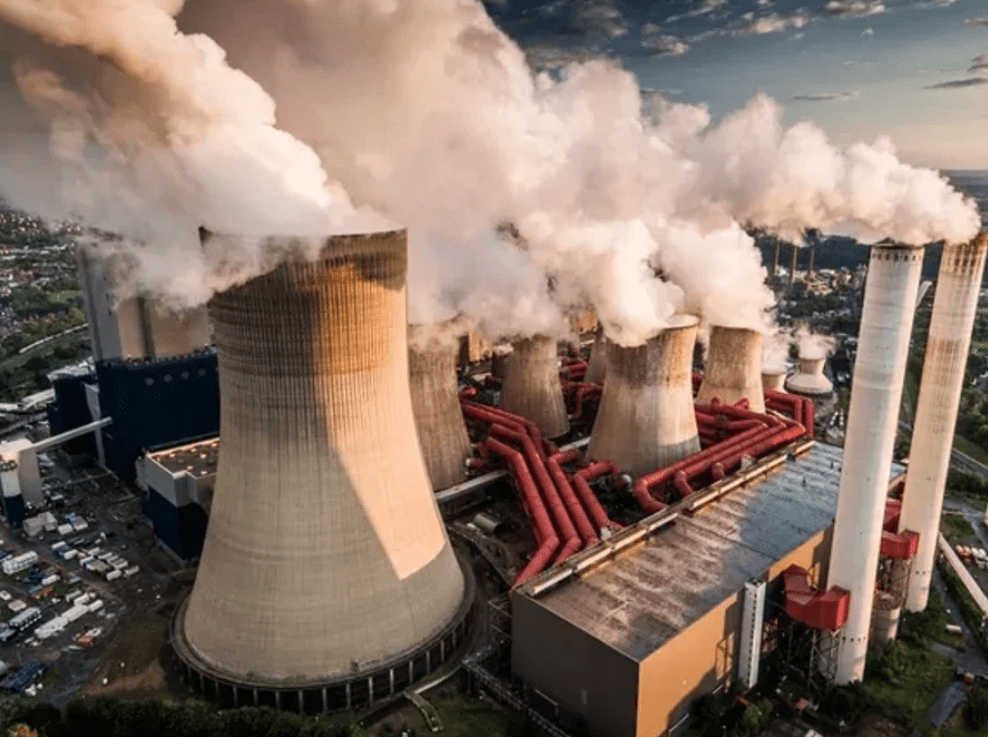
Reducing steam consumption in a plant can lead to significant energy savings, reduced operational costs, and improved overall efficiency. Since steam is often one of the most energy-intensive utilities used in industrial plants, focusing on minimizing its consumption is crucial for enhancing sustainability and profitability. Here are several strategies to reduce steam consumption:
1. Optimize Steam Traps and Condensate Recovery
- Check and maintain steam traps: Steam traps are essential for preventing steam from escaping, but they can malfunction over time, leading to steam wastage. Regular inspection, maintenance, and replacement of faulty traps can ensure steam is used efficiently.
- Recover condensate: Condensate is hot water produced when steam cools and condenses. It contains a significant amount of energy that can be recovered and reused. Installing a condensate recovery system ensures that this thermal energy is returned to the boiler, reducing the need for fresh steam generation.
2. Insulate Steam Lines and Equipment
- Insulate steam piping and equipment: Steam is typically transported through long piping systems, which can lose significant amounts of heat if not properly insulated. Insulating steam lines, valves, and other equipment helps to minimize heat loss and ensures that steam maintains its temperature during transit.
- Maintain proper insulation: Over time, insulation materials can degrade or become damaged, allowing heat to escape. Regularly inspect and replace damaged insulation to keep steam consumption to a minimum.
3. Use Steam More Effectively
- Match steam pressure to the needs of the process: Many plants generate steam at higher pressures than required by the process. By lowering the steam pressure to the actual requirements of the process, unnecessary steam consumption can be avoided.
- Improve heat exchanger performance: Using heat exchangers more efficiently allows you to recover and transfer heat from steam to other processes. Using the recovered heat in preheating systems, or in heat recovery units, reduces the need to generate fresh steam.
- Reduce steam losses: Minimizing the losses through leaks and inefficiencies in the system is key. Regular audits, tight seals, and proper maintenance of valves, fittings, and flanges can help to prevent steam from escaping.
4. Implement Process Optimization and Control
- Review steam usage data: Conduct regular audits and analyze steam consumption patterns to identify areas of excessive or unnecessary steam usage. Monitoring tools like flow meters and pressure gauges can provide real-time data, enabling better control of steam flow.
- Optimize process parameters: Make adjustments to production schedules, processes, and operating conditions to reduce steam demand. For example, adjusting the temperature or pressure requirements of certain processes may lower steam consumption without sacrificing product quality.
- Improve steam pressure regulation: Using a pressure-reducing valve system to maintain consistent pressure across the steam system can help optimize steam distribution and ensure that excess steam is not being generated.
5. Adopt Energy-Efficient Equipment
- Upgrade to more energy-efficient steam boilers: Older boilers tend to have lower efficiency due to wear and tear. Investing in newer, more efficient boiler systems can reduce steam consumption and enhance overall plant performance.
- Install Variable Frequency Drives (VFDs) on steam pumps: VFDs help adjust the speed of pumps and fans based on actual demand, thereby reducing the amount of steam used by preventing over-pumping or over-heating.
6. Improve Plant Automation and Control
- Automated control systems: Integrating automated control systems that adjust steam flow according to real-time demand can minimize unnecessary steam generation. By fine-tuning control systems, you can ensure that steam is only generated and distributed when and where it is needed.
- Use advanced monitoring systems: Implementing monitoring systems that track the real-time performance of steam systems (like flow rates, temperature, and pressure) can identify inefficiencies and alert plant operators to issues such as steam leaks, excessive heat losses, or improper operating conditions.
7. Implement Combined Heat and Power (CHP) Systems
- CHP systems: Combined Heat and Power systems, also known as cogeneration, generate both electricity and useful heat (usually steam) from the same energy source. By utilizing waste heat from power generation, these systems can reduce the amount of steam needed for heating and industrial processes.
8. Adopt Steam-Saving Technologies
- Steam economizers: Economizers capture the heat from flue gases or exhaust steam and use it to preheat feedwater, reducing the need for additional fuel or steam to raise water temperature. Installing economizers is an effective way to reduce the energy required to produce steam.
- Condensing steam turbines: For plants that use steam turbines for power generation, upgrading to condensing turbines can reduce the steam flow required to generate electricity, as they operate more efficiently by recovering more heat from the steam.
9. Energy Management and Employee Training
- Energy management practices: Implementing an energy management system (EnMS) aligned with standards like ISO 50001 can help track and manage energy use, identify opportunities for improvement, and implement energy-saving strategies across the plant.
- Employee training: Ensuring that plant personnel are trained in steam system management and energy-efficient practices can lead to significant reductions in steam consumption. Awareness of steam system operations, leak prevention, and efficient steam use can make a big difference in the overall efficiency of the plant.
10. Reevaluate Process Design
- Redesign processes to be more steam-efficient: In some cases, it may be possible to redesign the process itself to use less steam. For example, in certain heating applications, it might be more efficient to use hot water or thermal oil instead of steam. In these cases, analyzing the overall process and considering alternative heating methods could lead to reduced steam consumption.
By implementing these strategies, plant managers can significantly reduce steam consumption, leading to cost savings, improved energy efficiency, and a smaller environmental footprint. It requires a combination of operational adjustments, technological upgrades, and ongoing maintenance to ensure that steam is used as efficiently as possible throughout the plant.
Reducing steam consumption in a plant is essential for improving energy efficiency, cutting operational costs, and minimizing environmental impact. To achieve this, one of the first steps is optimizing the operation of steam traps. Faulty steam traps can lead to the unnecessary release of steam or allow condensate to accumulate in the system, which wastes energy. Regularly inspecting, maintaining, and replacing steam traps ensures they operate correctly and do not cause steam loss. Additionally, recovering condensate and reusing it in the system can recover much of the energy from the steam, reducing the need to generate fresh steam.
Proper insulation of steam lines, valves, and equipment is also crucial. Steam loses heat as it travels through uninsulated pipes, which increases the amount of steam required to meet process needs. By insulating steam pipes and equipment, plants can retain heat and prevent unnecessary energy consumption. Maintenance of insulation is equally important, as worn or damaged insulation can lead to significant heat loss.
Ensuring that steam pressure is appropriately matched to the process is another effective way to reduce steam consumption. Many plants generate steam at higher pressures than needed, which wastes energy. By adjusting the steam pressure to better align with the specific requirements of each process, you can minimize steam production and consumption. Heat exchangers also play a critical role, as improving their efficiency can allow for better recovery of heat from steam, which can be used elsewhere in the plant to reduce the need for additional steam generation.
One of the most effective methods for reducing steam consumption is to focus on process optimization. Regular audits of steam usage help identify areas where steam is being wasted. Monitoring tools, such as flow meters and pressure gauges, can give operators a clear view of steam usage patterns and help pinpoint inefficiencies. Moreover, optimizing process parameters—such as adjusting temperatures or pressures to better match operational needs—can lead to reductions in steam demand without compromising the quality of the output.
Using energy-efficient equipment, such as upgrading to more modern and efficient steam boilers, can also help reduce steam consumption. Newer boilers typically operate more efficiently, using less fuel to produce the same amount of steam, which ultimately leads to lower steam usage. Additionally, installing variable frequency drives (VFDs) on steam pumps can help adjust the flow based on actual demand, preventing the system from pumping more steam than necessary.
Automation and control systems also contribute significantly to reducing steam consumption. By implementing automated controls that regulate steam flow based on real-time demand, plants can minimize excess steam generation and reduce the likelihood of steam waste. Monitoring systems that track steam performance in real-time, such as sensors for temperature and pressure, can help identify problems, such as leaks or system inefficiencies, allowing operators to make adjustments before more significant issues arise.
Incorporating combined heat and power (CHP) systems into the plant can further reduce steam consumption. CHP systems generate both electricity and steam from a single energy source, allowing for the efficient use of otherwise wasted heat. By using waste heat for steam generation, these systems reduce the need for additional fuel consumption and maximize energy utilization.
Energy management practices and employee training can also help in steam reduction efforts. Implementing an energy management system (EnMS) to track and optimize energy use across the plant is an effective way to identify areas where steam consumption can be minimized. Employee training is essential to ensure that workers understand steam system management and energy-efficient practices. When plant staff are well-trained, they are better equipped to identify steam waste, optimize usage, and make adjustments as needed.
In some cases, rethinking process design can yield further reductions in steam consumption. For example, if steam is being used for heating, it might be more efficient to switch to alternative heating methods, such as hot water or thermal oil, depending on the process requirements. This reevaluation can lead to substantial savings by replacing steam with a more energy-efficient alternative where possible.
By combining these strategies—optimizing equipment, improving processes, investing in energy-efficient technologies, and ensuring proper maintenance—plants can reduce their steam consumption significantly. The result is lower operational costs, enhanced energy efficiency, and a reduced environmental footprint.
Beyond the typical optimization strategies, it’s also important to consider implementing more advanced technologies and innovative approaches to reduce steam consumption. For example, waste heat recovery systems are an excellent way to capture and reuse heat from exhaust gases or other industrial processes. This recovered heat can be used to preheat feedwater or even generate additional steam without the need for extra fuel, helping to reduce the overall demand for steam. Installing economizers, which recover heat from flue gases, is a great example of waste heat recovery in action, as it reduces the energy required to bring water to the required temperature.
Another way to reduce steam consumption is through the use of advanced control algorithms and predictive analytics. These systems analyze historical data and real-time conditions to optimize steam usage in real-time. Predictive maintenance systems can also help by forecasting when components are likely to fail, allowing operators to fix issues before they result in excessive steam waste. This proactive approach can greatly enhance steam efficiency and reduce unexpected losses.
Moreover, improving the design and layout of steam distribution systems can have a significant impact on steam consumption. By minimizing steam leakage, optimizing steam pipe sizing, and reducing the number of elbows and valves in the distribution network, steam flow can be more efficiently managed. Ensuring that the steam system is properly balanced—so that steam reaches the intended process areas without significant pressure loss—can also reduce the amount of steam needed to meet the demand.
Adopting digital twin technology is another advanced method that can optimize steam consumption. A digital twin creates a virtual model of the steam system, simulating how it behaves under various conditions. This model can help operators visualize steam flow, detect inefficiencies, and test different operating scenarios to find the most efficient methods for steam production and consumption.
In industries where steam is used for multiple purposes, such as heating, power generation, or process heat, segmentation of steam networks can be a useful approach. By creating dedicated steam circuits for different areas or processes, plants can avoid the unnecessary use of steam for processes that don’t require it at full pressure or temperature. For instance, processes that only require moderate heat can use lower-pressure steam, while high-temperature steam can be directed to areas where it is needed most.
Some facilities may also benefit from combining renewable energy sources like solar or geothermal with steam systems to supplement the energy needed for heating and power generation. For example, using solar thermal systems to preheat water before it enters the steam generator can reduce the energy load on the boiler, leading to lower fuel consumption and ultimately reduced steam production. Similarly, geothermal energy can be used to provide consistent heat for industrial processes, reducing the reliance on steam altogether.
Lastly, in some cases, switching to a closed-loop water heating system rather than relying on open-loop steam systems can reduce overall steam demand. Closed-loop systems recirculate water that is heated through various energy sources, requiring much less steam input and minimizing waste. This approach works especially well in processes where precise temperature control is necessary, as it ensures that the same heated water can be used multiple times without the need to generate additional steam.
By continuing to integrate new technologies, refine system design, and optimize operations, plants can make substantial reductions in steam consumption. This not only improves energy efficiency and reduces costs but also contributes to a plant’s long-term sustainability goals. Over time, these strategies lead to a more energy-efficient operation, less environmental impact, and a greater return on investment.
Waste Heat Recovery in Industry
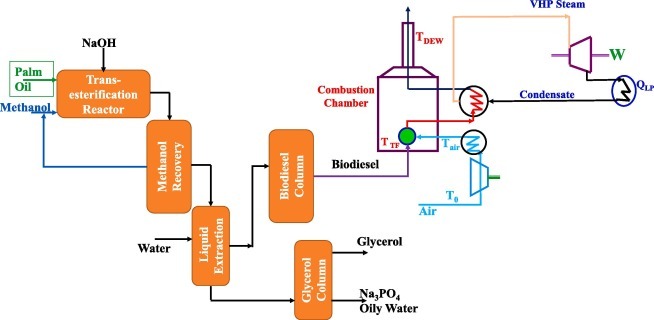
Waste heat recovery (WHR) in industry refers to the process of capturing and reusing heat that would otherwise be lost to the environment during industrial processes. This heat can be recovered from exhaust gases, hot liquids, or other byproducts of industrial activities. By capturing this waste heat and redirecting it for use in other parts of the operation, companies can significantly improve energy efficiency, reduce fuel consumption, and lower greenhouse gas emissions.
In industrial settings, waste heat is typically generated from processes that operate at high temperatures, such as in power generation, manufacturing, chemical processing, and metal production. The recovery and reuse of this heat not only reduces operational costs but also contributes to environmental sustainability by minimizing energy waste.
There are several methods of waste heat recovery, and they vary based on the temperature, type, and source of the waste heat, as well as the specific requirements of the industrial process. Here are some common methods used in industry:
- Heat Exchangers: Heat exchangers are devices that transfer heat between two fluids without mixing them. They are commonly used in waste heat recovery systems to capture heat from exhaust gases or hot liquids and transfer it to another fluid, such as water or oil. This recovered heat can then be used for preheating feedwater, space heating, or even steam generation for other processes. Plate heat exchangers, shell-and-tube heat exchangers, and air-to-air heat exchangers are common designs used for this purpose.
- Heat Recovery Steam Generators (HRSG): Heat recovery steam generators (HRSGs) are used in power plants and industrial facilities to recover waste heat from gas turbines, engines, or other combustion processes. The waste heat from exhaust gases is captured by the HRSG and used to produce steam, which can then be used for power generation or other processes in the plant. HRSGs are an essential component in combined-cycle power plants, where both gas and steam turbines are used to generate electricity efficiently.
- Waste Heat Boilers: Waste heat boilers are designed to recover heat from exhaust gases or other hot process streams and use it to generate steam or hot water. The recovered heat is typically used in applications such as district heating, process heating, or power generation. These boilers are particularly common in industries such as cement manufacturing, steel production, and petrochemical refining, where large amounts of waste heat are produced.
- Thermal Storage Systems: Thermal storage systems allow recovered waste heat to be stored for later use. These systems store heat in the form of molten salts, phase-change materials, or hot water. When heat is needed, it can be retrieved from the storage medium and used to generate steam, provide space heating, or assist in power generation. Thermal storage helps to address the issue of intermittent waste heat generation, allowing for more efficient use of the recovered energy.
- Organic Rankine Cycle (ORC): The Organic Rankine Cycle is a thermodynamic process used to recover low-temperature waste heat (typically below 400°F or 200°C) and convert it into electricity. The ORC works similarly to the traditional steam Rankine cycle, but instead of water, it uses an organic fluid with a lower boiling point. This allows the ORC system to recover heat from lower-temperature sources, such as exhaust gases from industrial processes, geothermal sources, or solar thermal systems. The ORC system generates electricity by expanding the organic fluid through a turbine, which drives a generator.
- Absorption Heat Pumps: Absorption heat pumps can be used to recover waste heat and elevate its temperature for use in other applications. These systems use a refrigerant or absorbent to capture heat from a low-temperature source (such as exhaust gases) and upgrade it to a higher temperature for heating or other process needs. This technology is particularly useful in industries where waste heat is available but cannot be directly used due to temperature limitations.
- Direct Use of Waste Heat: In some cases, waste heat can be used directly in the industrial process without further conversion. For example, in the chemical industry, hot exhaust gases can be used to preheat raw materials or process fluids, thereby reducing the need for additional energy input. In other cases, waste heat can be used for space heating in industrial buildings or drying applications.
- Combined Heat and Power (CHP) Systems: Combined heat and power (CHP) systems, also known as cogeneration, generate both electricity and useful heat from a single energy source. These systems are highly efficient because they capture waste heat from power generation and use it for heating purposes, reducing the need for separate heating systems. CHP systems are commonly used in industrial applications, such as in food processing, chemical production, and paper mills.
Benefits of Waste Heat Recovery in Industry:
- Energy Efficiency: The primary benefit of waste heat recovery is improved energy efficiency. By reusing waste heat, industries can reduce their reliance on external energy sources, cutting down on fuel consumption and electricity costs. This leads to substantial savings over time.
- Cost Savings: By reducing the need for additional fuel or electricity to generate steam, hot water, or power, waste heat recovery systems can significantly lower operational costs, particularly in energy-intensive industries.
- Reduced Emissions: Waste heat recovery contributes to reducing greenhouse gas emissions by decreasing the amount of fuel required for energy generation. This is particularly important for industries aiming to meet sustainability targets or comply with environmental regulations.
- Increased Competitiveness: Companies that invest in waste heat recovery technologies can improve their bottom line by reducing operating costs, which can enhance their competitiveness in the marketplace.
- Energy Independence: By recovering and reusing waste heat, industries can become less reliant on external energy suppliers, increasing their energy security and reducing vulnerability to fluctuations in energy prices.
- Regulatory Compliance: Many industries are subject to regulations and policies aimed at reducing energy consumption and emissions. Waste heat recovery can help companies comply with these requirements and avoid penalties or fines.
Challenges of Waste Heat Recovery:
- High Initial Costs: The installation of waste heat recovery systems, particularly those involving complex technologies like HRSGs or ORC systems, can have high upfront costs. However, these costs are often offset by long-term energy savings.
- System Complexity: Waste heat recovery systems can be complex to design, implement, and maintain, especially when retrofitting them into existing facilities. This requires specialized expertise and may involve additional costs for integration.
- Temperature and Quality of Waste Heat: The effectiveness of waste heat recovery depends on the temperature and quality of the waste heat. Higher temperature waste heat is more valuable for recovery, while low-temperature waste heat may require specialized technologies like ORC or absorption heat pumps.
- Space Requirements: Some waste heat recovery systems, such as heat exchangers or thermal storage units, can take up considerable space. This can be a challenge in facilities where space is limited.
Conclusion:
Waste heat recovery is a powerful strategy for improving energy efficiency, reducing costs, and contributing to environmental sustainability in industrial operations. By capturing and reusing waste heat, industries can minimize energy waste, lower operational costs, and reduce their carbon footprint. Despite the challenges, the long-term benefits of waste heat recovery make it a worthwhile investment for industries looking to optimize their energy use and enhance sustainability.
Waste heat recovery (WHR) in industrial settings is a crucial approach to improving energy efficiency, cutting operational costs, and reducing environmental impacts. Capturing and reusing waste heat that would otherwise be lost can make significant contributions to a company’s bottom line and sustainability goals. Industries that generate large amounts of waste heat, such as power generation plants, chemical factories, cement plants, steel mills, and food processing facilities, have the potential to benefit the most from WHR technologies.
By capturing heat from exhaust gases, flue gases, or other high-temperature byproducts of industrial processes, companies can reduce their reliance on external energy sources, like fuel and electricity. This means that processes such as heating, power generation, and steam production can be accomplished using heat that was previously wasted. Additionally, the captured heat can be used for other purposes in the plant, such as preheating feed water for boilers or even providing district heating for nearby communities.
One of the key methods of waste heat recovery is using heat exchangers, which transfer heat from one medium to another without the two mixing. For example, hot exhaust gases can pass through a heat exchanger to transfer heat to water, which can then be used in a steam generation process or for heating purposes. This method is commonly used in industries like petrochemical refining, cement manufacturing, and paper mills.
Another popular approach is employing waste heat boilers, which capture heat from exhaust gases or other waste streams and use it to generate steam or hot water. This steam can then be reused in various industrial applications, reducing the need for new steam generation. Waste heat boilers are a common sight in power plants, where they capture heat from combustion gases produced by turbines or engines.
For lower-temperature waste heat sources, technologies like Organic Rankine Cycle (ORC) systems come into play. These systems are designed to convert low-temperature heat (below 400°F or 200°C) into usable power. The ORC works by utilizing an organic fluid with a lower boiling point than water, allowing it to be vaporized by waste heat and subsequently drive a turbine to generate electricity. This is particularly useful for industries with significant waste heat at lower temperatures, such as food processing or waste-to-energy plants.
Thermal storage systems also play a role in maximizing the use of recovered waste heat. These systems allow industries to store heat for later use, providing flexibility and ensuring that the recovered energy is available even when waste heat is not being produced. For example, molten salts are often used as a thermal storage medium due to their high heat retention properties. This allows heat to be stored and retrieved when needed, improving the overall energy efficiency of the plant.
The integration of absorption heat pumps can also be a valuable method for recovering and upgrading waste heat. These systems capture low-grade waste heat and elevate its temperature for use in other parts of the plant or even for district heating applications. Absorption heat pumps are typically used in industries where waste heat is available at lower temperatures but is still too valuable to be discarded.
Waste heat recovery isn’t just limited to the capture of heat for direct reuse—it can also be a part of combined heat and power (CHP) systems, also known as cogeneration. These systems generate both electricity and useful heat from a single fuel source, maximizing the efficiency of the energy used. In a CHP system, the waste heat from power generation is captured and used for heating or other processes, reducing the need for additional fuel consumption and lowering overall energy costs. CHP systems are particularly common in industrial facilities like paper mills, chemical plants, and large manufacturing sites.
However, implementing waste heat recovery systems does come with its challenges. The initial investment in equipment and infrastructure can be significant, especially for advanced systems like Organic Rankine Cycles or heat recovery steam generators (HRSG). Furthermore, industries must carefully assess the quality and temperature of the waste heat available, as low-temperature heat may not be easily recoverable unless specific technologies, such as ORC systems, are used.
Another challenge is the space required to install waste heat recovery systems. Some of these systems, especially heat exchangers and thermal storage units, can take up considerable space in an industrial facility, which may be a concern for operations with limited available room. The integration of these systems with existing infrastructure can also require significant planning and engineering, particularly in older facilities or those with complex layouts.
Despite these challenges, the benefits of waste heat recovery far outweigh the drawbacks. By recovering heat, industries can significantly reduce their energy consumption, lower their operational costs, and minimize their carbon footprint. In addition, waste heat recovery contributes to regulatory compliance in industries with strict energy efficiency and environmental standards, such as those operating in the European Union or North America.
Ultimately, waste heat recovery is an essential tool for industries aiming to reduce their energy costs and carbon emissions. The recovery and reuse of waste heat not only contribute to a more sustainable operation but also enhance the economic viability of industrial processes. As technology continues to advance and industries face increasing pressure to adopt more sustainable practices, the importance of waste heat recovery will only continue to grow.
As industries continue to face rising energy costs and increasing environmental regulations, the role of waste heat recovery becomes even more significant. The ability to reduce energy consumption and improve operational efficiency is not just a matter of cost savings; it is also a key factor in reducing the carbon footprint of industrial operations. Waste heat recovery technologies help reduce the demand for fossil fuels, decrease greenhouse gas emissions, and move industries closer to their sustainability goals.
To further capitalize on the potential of waste heat recovery, industries are increasingly integrating these systems into smart energy management systems. By using digital monitoring and control technologies, companies can track waste heat production and optimize its recovery in real-time. These systems use sensors, meters, and software to provide operators with valuable data on temperature, flow rates, and system performance, which helps in making informed decisions about when and how to capture and reuse waste heat. In some cases, these systems can even predict waste heat generation based on factors like production schedules and environmental conditions, making it easier to plan for energy needs in advance.
One of the emerging trends in waste heat recovery is the development of modular and scalable systems. Instead of large, complex installations that require significant upfront investment, modular systems allow companies to gradually scale up their waste heat recovery capabilities over time. These smaller systems can be added incrementally as needed, helping companies manage their investment and adapt to changing operational requirements. Modular systems are particularly appealing for smaller businesses or those operating in facilities where space and capital investment may be limited.
Another significant trend is the integration of renewable energy sources alongside waste heat recovery systems. By combining waste heat recovery with renewable technologies like solar thermal systems or geothermal energy, industries can create more sustainable energy solutions. For example, solar thermal collectors can preheat water or air, which can then be further heated by waste heat recovery systems, significantly improving the overall efficiency of the energy system. Similarly, geothermal energy can provide a stable and sustainable heat source, which can be combined with waste heat recovery to reduce reliance on conventional energy sources.
In some sectors, such as district heating, waste heat recovery can have a broader impact. In district heating systems, waste heat from industrial processes or power plants is used to provide space heating and hot water to nearby residential, commercial, or industrial buildings. This creates a win-win scenario where both the industry and the surrounding community benefit from the recovered heat. For instance, a factory or power plant that generates significant amounts of waste heat can transfer that heat to a district heating network, allowing the heat to be used by homes or businesses without the need for additional fuel consumption.
Another innovative development is the use of artificial intelligence (AI) and machine learning (ML) in optimizing waste heat recovery. AI can analyze vast amounts of operational data to identify patterns, inefficiencies, and potential opportunities for improved waste heat recovery. By predicting the most efficient use of waste heat based on real-time data and historical trends, AI can help companies fine-tune their processes and recovery systems for maximum energy savings. Over time, as AI algorithms learn from plant operations, they can make increasingly accurate predictions that result in even greater energy savings.
Waste heat recovery can also be applied to industrial symbiosis, where multiple companies in a shared industrial park or region collaborate to exchange waste heat. For example, one facility might have excess waste heat that can be used by another nearby facility, reducing the need for additional heating or steam production. This kind of collaboration can create a more integrated and energy-efficient ecosystem, where resources are shared and waste is minimized.
Moreover, as the global focus on sustainability and energy efficiency intensifies, governments are introducing incentives and policies to promote waste heat recovery adoption. These can include tax credits, grants, and subsidies for companies that invest in energy-saving technologies, as well as stricter regulations on industrial emissions and energy consumption. Such measures not only help industries lower their operational costs but also support their transition to more sustainable practices.
While waste heat recovery systems offer significant advantages, it’s important to highlight that they are not a one-size-fits-all solution. Each industrial process has its unique energy requirements, and the effectiveness of waste heat recovery depends on the nature of the waste heat, its temperature, and the specific needs of the facility. As such, companies must carefully evaluate the potential benefits and costs of implementing waste heat recovery systems and select the technologies that are best suited to their operations.
In some cases, the decision to invest in waste heat recovery may depend on the scale of the operation. Large-scale industries with significant waste heat production, such as steel mills or cement factories, are more likely to see immediate, substantial returns on investment. Smaller industries, on the other hand, may benefit from modular systems or combining waste heat recovery with other energy-saving technologies to maximize efficiency without requiring large capital expenditures.
Ultimately, the adoption of waste heat recovery technologies is a crucial step toward more sustainable industrial operations. By recovering and reusing waste heat, industries can improve their energy efficiency, reduce operational costs, and significantly lower their environmental impact. As technology continues to evolve and industries explore new ways to optimize energy use, waste heat recovery will undoubtedly play an even more important role in creating energy-efficient, environmentally friendly industrial processes.
Reusing Heat from Work Processes Convection
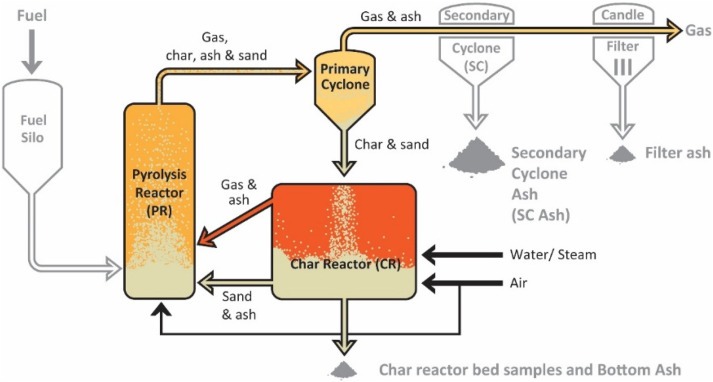
Reusing heat from work processes through convection is an effective method of waste heat recovery, especially in industrial applications where large amounts of heat are generated as a byproduct of various processes. Convection, in this context, refers to the transfer of heat through the movement of air, water, or other fluids. By capturing this waste heat, industries can improve energy efficiency, lower operational costs, and reduce environmental impact. Here’s how convection-based heat recovery systems can work and be applied in industrial settings:
Convection-Based Heat Recovery Systems
In industrial processes, heat is often lost to the environment in the form of hot gases, liquids, or surfaces. Convection can be used to transfer heat from these high-temperature sources to a medium that can either store or redirect the energy for use elsewhere in the process. Typically, heat recovery systems involve heat exchangers that use convection to recover heat from hot air, exhaust gases, or other waste heat streams.
Heat Exchangers for Convection
Heat exchangers designed for convection-based heat recovery typically use air or water as the heat transfer medium. The process works by transferring the heat from a hot exhaust or fluid stream to a cooler one, effectively “conveying” thermal energy through convection. Two common types of heat exchangers used in these systems include:
- Air-to-Air Heat Exchangers: These are typically used in environments where hot air is vented as a byproduct of processes like drying, baking, or combustion. In this system, the hot air is passed through a series of tubes or fins, which transfer the heat to cooler incoming air. This preheated air can then be used in the facility, reducing the need for additional energy to heat it to the desired temperature.
- Water-to-Air Heat Exchangers: In some processes, waste heat is transferred to a circulating water stream, which then absorbs the heat through convection. This heated water can be used for a variety of purposes, including preheating feedwater for boilers, space heating, or even cooling down other process streams in the facility.
Use in Industrial Applications
The potential for reusing heat from work processes via convection can be applied in a wide range of industries. Some common industrial applications include:
- Cement and Steel Production: Both of these industries generate substantial amounts of waste heat during the high-temperature processes used to make cement or steel. In these settings, convection-based heat recovery systems can be used to capture heat from exhaust gases and transfer it to either incoming air or water for reuse. The heat recovered can then be used for preheating combustion air, saving energy in the furnace and improving overall efficiency.
- Food and Beverage Industry: In food processing plants, particularly in processes like drying, baking, or frying, convection heat recovery systems can capture waste heat from the exhaust air and reuse it for heating other parts of the production line. This helps to reduce energy costs associated with generating steam or hot air for cooking and drying.
- Chemical and Petrochemical Industries: Chemical and petrochemical plants often use large amounts of energy to maintain high temperatures for various reactions and distillation processes. Heat exchangers that capture heat through convection can help reclaim heat from hot air or gases and transfer it to other process streams, reducing the need for additional heating and improving energy efficiency.
- Power Generation: In power plants, particularly those that use steam turbines, waste heat is often released from the cooling systems or exhaust gases. Convection-based heat recovery systems can capture this heat and use it to preheat feedwater before it enters the boiler, which can reduce fuel consumption and increase overall plant efficiency.
Benefits of Convection-Based Heat Recovery
- Energy Savings: By capturing and reusing waste heat through convection, industries can significantly reduce their need for additional energy sources, such as natural gas or electricity. This results in cost savings and a more efficient energy system.
- Reduced Carbon Footprint: Waste heat recovery systems reduce the amount of energy that needs to be produced, leading to a decrease in emissions. This contributes to the overall reduction in the carbon footprint of industrial operations.
- Improved Operational Efficiency: By making use of available waste heat, processes can be optimized to run at more efficient temperatures, reducing the strain on heating systems and allowing for more consistent performance across operations.
- Cost-Effectiveness: The initial investment in heat recovery systems is typically offset by the long-term savings in energy consumption. Over time, these systems can result in substantial cost reductions, especially for energy-intensive industries.
- Compliance with Regulations: Many industries face increasing pressure to meet environmental standards and regulations. Implementing heat recovery systems can help companies comply with these regulations by reducing their energy use and carbon emissions.
Challenges and Considerations
While convection-based heat recovery is an effective solution, there are challenges to consider:
- Initial Capital Investment: The cost of installing convection-based heat recovery systems can be high, especially in industries where retrofitting is required. However, these systems generally pay off in the long term through energy savings.
- System Integration: Integrating heat recovery systems into existing processes can be complex. It requires careful design to ensure that recovered heat can be efficiently used in the system without disrupting existing operations.
- Temperature Gradients: The effectiveness of convection heat recovery depends on the temperature difference between the heat source and the medium that is absorbing the heat. Lower temperature gradients result in less efficient heat transfer, which can limit the potential for heat recovery.
- Maintenance: Heat exchangers and other components of convection-based recovery systems need regular maintenance to ensure optimal performance. Over time, systems can accumulate dust, soot, or corrosion, which can reduce efficiency.
Conclusion
Reusing heat from work processes through convection is a highly effective way to improve energy efficiency in industrial applications. By recovering and reusing heat that would otherwise be wasted, industries can lower their energy consumption, reduce operational costs, and minimize environmental impacts. Heat exchangers designed to recover heat through convection offer a relatively simple and cost-effective way to recycle thermal energy within industrial systems. Although there are challenges in terms of initial investment and system integration, the long-term benefits make convection-based heat recovery a valuable solution for industries seeking to optimize their energy use and sustainability efforts.
Reusing heat from work processes through convection is a highly effective approach for industries to recover waste heat and optimize energy use. The main advantage of this method is its ability to capture heat that would otherwise be lost to the environment, such as through exhaust gases or hot surfaces, and redirect it for beneficial use within the process. The key to making this process work efficiently lies in the application of heat exchangers, which transfer heat from hot streams (such as exhaust air or gases) to cooler fluids like water or air.
In industries like cement, steel, food processing, and power generation, convection-based heat recovery systems can be integrated to reclaim heat from waste gases or hot fluids. This recovered heat can be used in a variety of ways, such as preheating combustion air, generating steam, or heating water for other processes. For example, in cement production, heat recovery from exhaust gases can be redirected to preheat the raw materials before they enter the furnace, reducing the amount of fuel required for the process. In the food and beverage industry, hot exhaust air from ovens or drying equipment can be captured and used to preheat air or water for other parts of the production process, reducing energy consumption.
Additionally, convection-based systems can be employed to capture heat in power plants. In these plants, hot exhaust gases or cooling water are often discharged into the environment, but through the use of heat exchangers, this heat can be recovered and used to preheat water before it enters the boiler, reducing the need for additional energy to raise the water temperature. This improves the overall efficiency of the power generation process.
The benefits of convection-based heat recovery systems are considerable. By capturing and reusing waste heat, companies can significantly reduce their reliance on external energy sources like natural gas or electricity. This results in energy savings and a reduction in operational costs. Moreover, since less fuel is required to produce the same amount of energy, companies can reduce their carbon emissions, helping them meet environmental sustainability goals and comply with regulations.
While the initial investment in convection-based heat recovery systems can be high, particularly when retrofitting existing facilities, the long-term savings typically outweigh the costs. Furthermore, as energy prices continue to rise and regulatory pressures increase, the economic case for investing in waste heat recovery becomes even stronger. The operational efficiency improvements also result in better performance across the board, helping industries reduce energy waste and operate at a more consistent and predictable level.
The challenges with convection-based systems mainly revolve around the need for careful design and integration. For instance, the system must be tailored to the specific temperature, flow rates, and characteristics of the waste heat generated by the process. The system’s performance is largely dependent on the temperature difference between the hot and cold streams, with higher temperature gradients leading to more efficient heat recovery. If the temperature gradient is low, the system may not be as effective, and alternative recovery methods may be necessary.
Additionally, the heat exchangers and other components in these systems need regular maintenance to ensure that they continue to perform at their optimal level. Over time, heat exchangers can accumulate dust, soot, or corrosion, which can degrade their efficiency and reduce heat transfer rates. To maintain system effectiveness, periodic cleaning and inspection are essential, which adds to the overall maintenance cost.
Despite these challenges, the benefits of reusing heat from work processes through convection remain clear. Industries that adopt this approach not only lower their energy costs but also contribute to their sustainability efforts by reducing waste heat and emissions. As technologies continue to evolve and more industries recognize the value of heat recovery, convection-based systems will play an even larger role in helping businesses achieve greater energy efficiency, reduce operating costs, and minimize their environmental footprint. With the ongoing focus on energy optimization, convection-based heat recovery offers a valuable tool for industries to become more sustainable and cost-effective in the long term.
As industries continue to explore more efficient ways to reduce energy consumption and lower operational costs, convection-based heat recovery remains a vital strategy. The adoption of heat recovery technologies is accelerating as companies seek to address both economic and environmental challenges. The ability to reuse heat from work processes through convection has gained attention because it can provide immediate savings, increase system efficiencies, and contribute to more sustainable practices.
Another aspect of heat recovery systems is their flexibility. They can be tailored to different process requirements, making them adaptable to various industries. For instance, some processes might generate heat at higher temperatures, making them suitable for direct use in steam generation or preheating. In contrast, lower temperature waste heat can still be effectively recovered and reused for applications like space heating or domestic water heating. This versatility allows companies to prioritize where heat recovery is most beneficial, whether it’s in industrial processes, facility heating, or even district energy systems.
Technological advancements also continue to improve the efficiency of convection-based heat recovery systems. One such development is the use of advanced materials for heat exchangers. New alloys and coatings can improve heat transfer rates and reduce the impact of fouling, which is a common issue in heat exchangers exposed to high temperatures and particulate matter. Moreover, some systems are designed to automatically clean or self-regulate to maintain high efficiency, reducing the need for manual maintenance.
The integration of sensors and smart controls into heat recovery systems further enhances their performance. These systems can monitor temperature, flow rates, and energy usage in real time, allowing for better optimization of heat recovery processes. For example, sensors can adjust the flow of air or water in response to changes in temperature or production demands, ensuring that the system operates at peak efficiency without wasting energy.
District heating networks, which involve the distribution of recovered heat from industrial processes to nearby buildings, are another promising application of convection-based heat recovery. In large industrial zones or clusters of facilities, waste heat from one company can be transferred to others, reducing the need for external heating sources like natural gas boilers. This creates a more circular and efficient energy system, where industries and communities work together to minimize waste and maximize energy utilization.
Additionally, the economic landscape is changing in favor of energy-efficient solutions. As global energy prices fluctuate, businesses are increasingly incentivized to adopt technologies that reduce their dependence on external energy suppliers. In many regions, governments are also offering financial incentives, subsidies, and tax breaks for companies that invest in energy-efficient technologies, including heat recovery systems. These incentives can help offset the initial investment required for implementing heat recovery systems, making them more attractive to businesses looking to optimize their operations.
At the same time, environmental regulations are becoming stricter, pushing industries to reduce their carbon emissions and minimize environmental impacts. This regulatory pressure, combined with the growing public and shareholder demand for sustainability, is driving companies to adopt waste heat recovery systems. By utilizing recovered heat, industries can significantly cut their energy use, reduce emissions, and comply with environmental standards.
One potential growth area for convection-based heat recovery is its application in emerging industries. Sectors such as data centers and renewable energy production are seeing an increased need for heat management. Data centers, for example, consume large amounts of energy to power servers and cool their environments. The waste heat generated in these facilities can be captured and used for heating nearby buildings or used in other applications, such as district heating or agricultural heating. Similarly, renewable energy plants, such as those using geothermal or solar thermal energy, can benefit from advanced heat recovery systems to maximize the efficiency of energy production.
However, it’s important to recognize that not all waste heat can be effectively recovered using convection methods. Some processes generate waste heat at low temperatures, where traditional convection-based systems may not be effective. In these cases, companies may need to consider alternative heat recovery technologies such as organic Rankine cycle (ORC) systems, which are designed to recover heat from lower-temperature sources. The combination of convection systems with these alternative methods can provide a more holistic solution for waste heat recovery in industries with varying heat characteristics.
As the focus on sustainable industrial practices continues to grow, industries are finding that heat recovery is not just a way to cut costs, but also a vital strategy for ensuring long-term competitiveness. Companies that embrace waste heat recovery, including convection-based systems, position themselves to become leaders in energy efficiency and sustainability. These systems not only make good economic sense but also contribute to a broader, global effort to reduce energy consumption, mitigate climate change, and build a more sustainable industrial future.
The future of waste heat recovery through convection looks promising, with ongoing advancements in materials, system design, and integration with smart technologies. As more industries adopt these systems, the collective impact on global energy use and emissions will grow, making waste heat recovery a central part of industrial decarbonization and energy optimization strategies.
Reusing Heat from Work Processes Co-generation
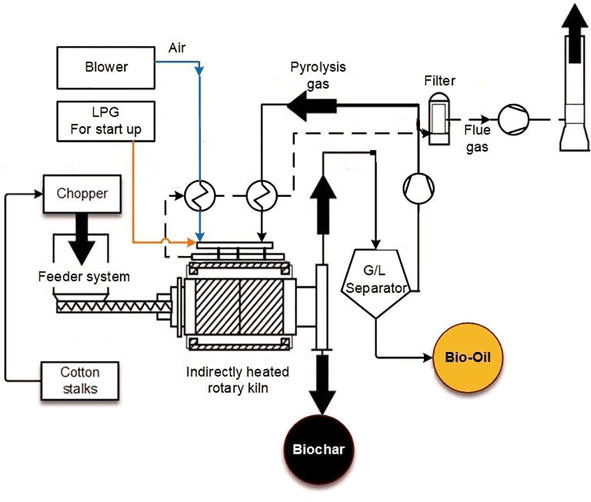
Reusing heat from work processes through co-generation (also known as combined heat and power, or CHP) is a highly efficient and sustainable method of utilizing waste heat for additional energy production. Co-generation involves the simultaneous production of both electricity and useful heat from a single energy source, typically in an industrial, commercial, or power generation setting. By capturing and reusing the heat that would otherwise be wasted in electricity generation, co-generation systems increase the overall energy efficiency and reduce the need for separate heat and power generation units.
In a typical co-generation system, a fuel (such as natural gas, biomass, or coal) is burned in a prime mover, such as a gas turbine, steam turbine, or internal combustion engine. The primary function of the prime mover is to generate electricity. However, the process also produces excess heat as a byproduct, which can be recovered and used for other applications, such as heating, steam generation, or industrial processes. This combined approach significantly improves overall efficiency compared to conventional separate power generation and heating systems, where each process operates independently and with lower overall efficiency.
How Co-Generation Works
- Electricity Generation: The prime mover (e.g., turbine or engine) generates electricity using the fuel. This electricity is either used on-site for operational needs or fed into the grid.
- Heat Recovery: The exhaust gases or steam from the prime mover are at high temperatures. This waste heat is captured through heat recovery systems, such as heat recovery steam generators (HRSGs), which capture the exhaust heat and use it to produce steam or hot water.
- Heat Utilization: The recovered heat is then directed to various systems that require heat for industrial processes, space heating, or water heating. In many cases, the recovered heat is used to produce steam for further power generation, creating a continuous cycle of energy production and consumption. Alternatively, heat can be used directly in industrial processes such as chemical manufacturing, food processing, or district heating systems for surrounding communities.
Benefits of Co-Generation Systems
- Improved Energy Efficiency: Co-generation systems are more efficient than traditional methods of power generation and heat production. In conventional systems, electricity is generated through combustion, and the byproduct heat is typically released into the atmosphere. In co-generation, this waste heat is captured and used for useful purposes, improving overall energy efficiency. Co-generation systems can achieve efficiencies of 70-90%, compared to conventional systems that typically operate at around 30-40% efficiency.
- Reduced Fuel Consumption: By generating both electricity and heat from the same fuel source, co-generation systems reduce the overall demand for fuel compared to separate heat and power generation. This not only results in cost savings for companies but also decreases the environmental impact by lowering the amount of fuel burned and reducing emissions.
- Cost Savings: Co-generation systems can lead to substantial savings on both energy bills and infrastructure costs. Since electricity and heat are generated simultaneously, companies can reduce the need for separate heating systems, such as boilers, and can rely on the power generated by the system, reducing dependence on external electricity suppliers.
- Environmental Benefits: By increasing the efficiency of energy production and reducing fuel consumption, co-generation systems also contribute to lower greenhouse gas emissions. This makes co-generation a critical component of sustainable energy strategies for industries looking to reduce their carbon footprint and comply with regulatory standards.
- Flexibility: Co-generation systems are versatile and can be customized to suit the specific energy needs of different industries. The size, configuration, and type of fuel used can be tailored based on the scale of operation and the specific requirements of the facility. For example, smaller-scale co-generation systems can be used for hospitals, universities, or large commercial buildings, while larger systems are suited for industrial plants like refineries or chemical manufacturing facilities.
- Grid Stability and Reliability: Co-generation systems can also improve the stability and reliability of the power grid. Since the system is capable of generating both heat and electricity, it can reduce reliance on grid-based electricity, providing backup power during outages or periods of high demand. In regions with unreliable electricity supply, co-generation systems can help improve energy security.
Industrial Applications of Co-Generation
- Chemical and Petrochemical Industries: Co-generation is widely used in chemical plants and refineries, where both electricity and steam are critical to operations. By integrating co-generation systems, these industries can improve the efficiency of their energy use, reduce operational costs, and meet their significant energy needs.
- Food and Beverage Industry: The food and beverage sector, especially in processes like drying, cooking, and pasteurization, requires a large amount of heat. Co-generation systems can efficiently meet both the power and heat demands of these processes, improving the overall energy efficiency and reducing reliance on external energy sources.
- Pulp and Paper Industry: Pulp and paper mills are typically large consumers of energy, using both electricity and heat in their processes. Co-generation systems in these facilities can recover waste heat from boilers or turbines, supplying steam for drying and cooking, and electricity for running machinery.
- District Heating: In regions with high population density or industrial clusters, co-generation systems can be used for district heating. The waste heat from industrial co-generation systems can be distributed through a network of pipes to provide heating for homes, businesses, and public buildings in the surrounding area. This is particularly beneficial in areas with colder climates where heating demands are high.
- Data Centers: Data centers, which require substantial amounts of power for cooling and running servers, are increasingly adopting co-generation systems to improve their energy efficiency. By generating both electricity and heat on-site, data centers can reduce the need for external cooling systems and lower operational costs while improving their environmental footprint.
Challenges and Considerations
- Initial Capital Investment: The upfront costs of installing a co-generation system can be significant, particularly for large-scale installations. However, the long-term savings from reduced energy consumption and lower fuel costs typically offset these initial expenses over time.
- Maintenance and Operation: Co-generation systems require ongoing maintenance to ensure optimal performance. The efficiency of the system depends on regular inspections, cleaning, and replacement of parts such as turbines, generators, and heat exchangers.
- Fuel Availability and Cost: The economic viability of co-generation systems is influenced by the availability and cost of fuel. In areas where fuel prices fluctuate significantly or are subject to supply constraints, the cost-effectiveness of co-generation systems may be impacted.
- Complexity in Integration: Integrating a co-generation system into an existing industrial process can be complex, requiring careful planning and customization. Proper system design is crucial to ensure that both electricity and heat demands are met efficiently without overloading or underutilizing components of the system.
Conclusion
Co-generation offers a powerful solution for industries looking to optimize energy use, reduce operational costs, and improve sustainability. By capturing and reusing waste heat for both electricity generation and heating purposes, co-generation systems significantly increase energy efficiency compared to conventional methods. This not only leads to substantial savings on energy bills but also reduces greenhouse gas emissions, helping companies meet environmental standards and contribute to a more sustainable energy future. As energy costs continue to rise and regulatory pressures increase, the adoption of co-generation systems will likely become even more widespread, especially in industries with high energy demands. Through continued advancements in technology, co-generation will remain a key component in efforts to create more sustainable and efficient industrial operations.
Co-generation continues to be a vital solution for industries aiming to optimize energy efficiency and minimize waste. One of the most significant advantages of co-generation systems is their ability to simultaneously generate electricity and recover heat, which enhances overall energy utilization. This results in a drastic reduction in fuel consumption compared to traditional methods where heat and power are produced separately, which often leads to energy wastage. By recovering and reusing waste heat, co-generation systems can reach efficiencies as high as 70-90%, compared to the 30-40% efficiency typical of conventional power generation.
Another important factor driving the adoption of co-generation is its ability to meet both the heat and power needs of industries, making it particularly valuable for energy-intensive sectors. Industries like chemicals, petrochemicals, paper, and food processing all benefit from co-generation systems, as they require significant amounts of heat for their production processes, while also needing reliable electricity to power equipment and machinery. By integrating co-generation systems into these operations, companies can simultaneously meet both needs, reducing their dependency on external sources for both power and heat.
Additionally, co-generation systems offer substantial cost savings. Since the heat recovered from electricity generation is reused in the process, companies reduce the amount of fuel needed to produce heat, which lowers energy costs over time. This dual benefit of both heat and power generation enables organizations to make a quicker return on their investment. While the initial setup costs for co-generation systems may be high, the energy savings and operational efficiencies typically lead to payback within a few years, depending on the size and complexity of the system.
On a larger scale, co-generation can also contribute to grid stability. In regions where electricity supply is unreliable, co-generation systems can function as a distributed generation source, providing backup power during peak demand or outages. This ability to generate both heat and electricity on-site ensures that industries are less reliant on the central power grid, providing greater energy security. In some instances, co-generation facilities even feed excess power into the grid, which can help support the broader energy system, especially in areas where renewable energy sources like solar or wind are intermittently available.
The environmental benefits of co-generation are another compelling reason for its widespread adoption. By improving energy efficiency, these systems reduce the need for burning additional fuel, which leads to lower emissions of carbon dioxide (CO2) and other greenhouse gases. With growing concerns about climate change and stricter regulatory standards for emissions, co-generation represents a clear path for industries to reduce their carbon footprint while still meeting their energy demands. Moreover, as industries increasingly aim for carbon neutrality, co-generation systems can play a critical role in reducing fossil fuel consumption and contributing to more sustainable practices.
Despite these benefits, implementing co-generation systems does come with challenges. Initial capital investment can be significant, especially for large-scale systems that require high-capacity turbines, generators, and associated heat recovery equipment. The complexity of installation and integration with existing infrastructure can also pose challenges, particularly in industries where space and resources are limited. In such cases, a careful feasibility study and system design are crucial to ensure that the system delivers the expected returns on investment.
Another consideration is the availability and cost of fuel. Co-generation systems rely on consistent access to fuel, such as natural gas, biomass, or coal, and fluctuations in fuel prices can impact the cost-effectiveness of these systems. Additionally, industries located in regions with limited access to affordable fuel may face higher operational costs, making alternative energy sources or technologies more attractive.
Maintenance is another crucial factor for ensuring that co-generation systems operate efficiently over time. Regular monitoring and maintenance are necessary to keep turbines, generators, heat exchangers, and other components in optimal working condition. Over time, wear and tear from high-temperature operations can reduce system efficiency, and preventive maintenance is required to avoid costly breakdowns.
Despite these challenges, the flexibility of co-generation systems allows for their adaptation to various energy needs. Systems can be scaled up or down based on the specific requirements of the operation, and the choice of fuel can be tailored to the resources available in the region. Furthermore, advancements in technology, such as combined cycle systems, which involve using both gas and steam turbines to improve efficiency, are making co-generation systems even more powerful and efficient.
The future of co-generation is promising, particularly as industries continue to prioritize energy efficiency and sustainability. With smart technology integration, such as sensors and automated controls, co-generation systems can be more easily optimized in real-time, adjusting to fluctuations in energy demand or changes in fuel availability. Energy management systems can integrate with co-generation to better control heat and power distribution, ensuring that energy is being used as efficiently as possible across the entire facility.
As the global push for sustainable development intensifies, industries are likely to increase their adoption of co-generation systems, especially in regions where energy prices are high, or environmental regulations are stringent. Co-generation offers a path to reduce both operating costs and environmental impact while contributing to a more resilient and energy-efficient future. With ongoing advancements in technology and growing emphasis on sustainable practices, co-generation will continue to be a key element in the drive toward cleaner, more efficient industrial energy solutions.
The growing emphasis on energy efficiency and sustainability in industrial processes is pushing more industries to explore co-generation systems as a core part of their operations. As energy costs rise and environmental pressures increase, co-generation becomes even more appealing for businesses looking to reduce costs while improving their environmental footprint. Additionally, with global energy demand continuing to increase, co-generation systems are increasingly seen as an essential technology for addressing both energy production and consumption challenges.
One of the advancements that are making co-generation systems even more effective is the integration with renewable energy sources. For example, combining co-generation with biomass power plants allows industries to use renewable fuels, such as wood pellets or agricultural waste, in their co-generation systems. This not only reduces reliance on fossil fuels but also contributes to a lower carbon footprint, aligning with sustainability goals. Similarly, integrating solar thermal systems with co-generation could help increase the overall efficiency by providing pre-heated water or air, reducing the amount of energy required for heating.
Additionally, district heating networks are an emerging trend that benefits from co-generation systems. In areas with multiple industrial or residential buildings, waste heat generated by large-scale industrial co-generation systems can be captured and distributed through a centralized heating network. This helps lower the cost of heating for surrounding buildings while reducing emissions. In countries with colder climates or densely populated areas, co-generation and district heating are seen as a practical way to make energy use more efficient and environmentally friendly. By recovering and reusing waste heat from one facility and sharing it with others, co-generation systems create a circular energy economy, which further reduces waste and improves the overall sustainability of energy systems.
The integration of smart grids and digital technologies is another promising trend that will further enhance the effectiveness of co-generation systems. Smart grids can optimize the flow of electricity generated by co-generation units by distributing power based on real-time demand and system conditions. This integration allows businesses to better manage their energy usage, improve grid reliability, and even sell excess energy back to the grid, generating additional revenue. Energy storage technologies, such as batteries, can also play a role in improving the efficiency of co-generation systems by storing excess energy for use when demand spikes or when the system is not generating enough electricity.
Another exciting development is the use of fuel cells in combination with co-generation. Fuel cells are devices that convert chemical energy directly into electrical energy through an electrochemical process, and they are typically highly efficient and environmentally friendly. When used in co-generation systems, fuel cells can provide an additional source of electricity while producing minimal emissions. By coupling fuel cells with traditional power generation and heat recovery methods, businesses can further increase system efficiency and reduce their reliance on conventional fossil fuels. This hybrid approach may become increasingly important as industries strive to meet both energy and environmental goals.
As industrial sectors continue to evolve, it’s clear that energy efficiency is not just a cost-saving measure but also a competitive advantage. Co-generation systems provide a way for companies to improve their efficiency, enhance sustainability, and reduce their carbon footprint. Government incentives, including subsidies, tax credits, and rebates for energy-efficient technologies, are further making it easier for industries to invest in co-generation systems. These incentives can help offset the initial investment costs, making co-generation a more attractive solution for many companies looking to modernize their energy systems.
For example, corporate sustainability goals are increasingly demanding that businesses take action to reduce their environmental impact. Co-generation systems align perfectly with these goals by improving energy efficiency, reducing emissions, and lowering fuel consumption. By integrating co-generation into their operations, companies can not only reduce costs but also demonstrate their commitment to sustainable business practices, which is becoming a critical factor for consumers, investors, and regulatory authorities.
Despite these advances and benefits, some challenges remain for industries looking to adopt co-generation systems. Regulatory hurdles are one such challenge, as permitting and compliance requirements can be complex, particularly when retrofitting existing systems or implementing large-scale projects. Governments may also impose limits on emissions or have regulations around fuel use, which could affect the operation of certain co-generation units. Additionally, while co-generation is highly efficient, fuel flexibility can still pose a challenge, particularly in regions where certain fuels (such as biomass or natural gas) may be in limited supply or subject to price fluctuations.
Another consideration is the maintenance and lifecycle management of co-generation systems. While they offer long-term savings and efficiency, proper operation and maintenance are critical to ensuring the system continues to perform well. This involves regular inspections, part replacements, and system optimization to avoid issues such as corrosion or inefficient combustion. Preventive maintenance is important to maintain system performance and to prevent costly repairs or unplanned downtime.
Furthermore, the scale of co-generation systems can be both an advantage and a challenge. While large industrial facilities can benefit from high-capacity co-generation units, smaller businesses or industries may struggle with the initial investment and complexity. For smaller industries, modular and scalable co-generation systems are becoming more common. These systems can be designed to fit the specific energy needs of smaller facilities while still providing the energy savings and environmental benefits of larger systems.
As the global economy continues to shift towards sustainable energy solutions, co-generation is poised to play a crucial role in the transformation of industrial energy systems. Through technological advancements, cost-effective integration with renewable energy, and digital optimizations, co-generation will continue to improve energy efficiency, reduce environmental impacts, and support the drive towards a more sustainable energy future. As industries and governments prioritize carbon reduction and energy efficiency, the role of co-generation in both improving business performance and contributing to global sustainability goals will only increase.
In conclusion, co-generation represents a powerful and efficient solution for industries looking to optimize their energy use and reduce waste. By generating both electricity and heat from a single source, these systems significantly improve overall energy efficiency while lowering operational costs and reducing emissions. As technology continues to evolve and industries become more focused on sustainability, co-generation will remain a key strategy for companies aiming to meet their energy needs while contributing to a greener, more sustainable future. With the ongoing drive toward decarbonization and energy optimization, the adoption of co-generation systems will continue to grow, making it a critical component of modern industrial energy solutions.
Industrial Waste Heat
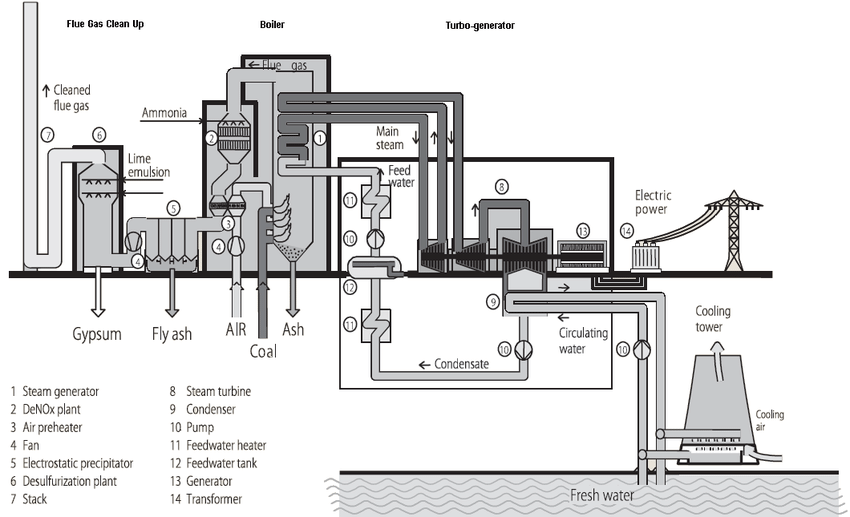
Industrial waste heat refers to the heat that is produced as a byproduct of industrial processes, which is typically released into the environment without being used for any productive purpose. This heat is often lost through exhaust gases, cooling systems, or hot surfaces, but it can represent a significant amount of energy that, if recovered, could improve energy efficiency and reduce operational costs.
Many industrial processes, especially those in energy-intensive industries, generate substantial amounts of waste heat. Examples include steel production, cement manufacturing, chemical processing, and petroleum refining. This waste heat typically exists in the form of hot gases, liquids, or even solid materials. If not recovered or reused, it can contribute to increased energy consumption, higher operational costs, and unnecessary carbon emissions.
Sources of Industrial Waste Heat
- Exhaust Gases: Many industrial processes, particularly combustion-based operations, produce high-temperature exhaust gases. For example, in furnaces and boilers, the exhaust gases from burning fuels contain significant thermal energy. If not captured, this heat is vented into the atmosphere, leading to wasted energy.
- Cooling Water: In industries where cooling is required (e.g., chemical plants, power generation), water is used to absorb excess heat. This water, often discharged at elevated temperatures, represents another source of waste heat.
- Hot Surfaces: Industrial processes involving hot equipment, such as reactors, kilns, or heat exchangers, can have surfaces that radiate or transfer heat. These surfaces, if left uninsulated or poorly managed, contribute to heat loss.
- Process Waste Streams: In certain manufacturing processes, waste heat can be generated through byproducts or residual materials, such as molten slag in metal production or hot gases from chemical reactions. These streams can be another source of thermal energy.
- Flash Steam: In industries that deal with steam generation, such as power plants or food processing, flash steam—the steam produced from the sudden release of pressure on hot water—represents another potential source of recoverable heat.
Methods of Waste Heat Recovery
- Heat Exchangers: These devices are used to transfer heat from one fluid to another without mixing them. In industrial settings, heat exchangers can recover heat from exhaust gases or cooling water, which can then be used for heating, preheating, or steam generation. Common types of heat exchangers used for waste heat recovery include shell and tube heat exchangers, plate heat exchangers, and air-to-air heat exchangers.
- Heat Recovery Steam Generators (HRSGs): In combined heat and power (CHP) or co-generation systems, HRSGs recover heat from exhaust gases (e.g., from a gas turbine or industrial furnace) to produce steam. This steam can then be used to drive a turbine, generate electricity, or provide process heat.
- Organic Rankine Cycle (ORC): For low-temperature waste heat recovery, the Organic Rankine Cycle is a popular technology. It works similarly to a steam turbine but uses organic fluids (such as refrigerants) with a lower boiling point, making it ideal for recovering heat from lower-temperature waste streams.
- Absorption Chillers: Waste heat can be used for cooling purposes via absorption chillers, which use heat to drive a refrigeration cycle. This system is particularly beneficial in industries where both cooling and waste heat recovery are required.
- Thermoelectric Generators (TEGs): These devices convert heat directly into electricity using the Seebeck effect, which occurs when there is a temperature difference between two different materials. While still in the early stages of development for industrial use, TEGs offer a potential method for recovering waste heat and generating electricity.
- District Heating: Large industrial facilities can feed recovered waste heat into a district heating system, where the heat is distributed to nearby buildings or communities for residential and commercial heating purposes. This is commonly done in industrial zones or urban areas.
- Molten Salt Heat Storage: For industries that generate heat continuously, such as in steel mills or cement kilns, molten salts can be used to store waste heat for later use. The salt absorbs and stores heat and can then release it when needed, such as during periods of peak demand or when processes require additional heating.
Applications of Recovered Waste Heat
- Preheating: One of the most common uses of recovered waste heat is to preheat air, water, or raw materials entering a process. For example, recovered heat from exhaust gases can be used to preheat combustion air in a furnace, reducing the amount of fuel needed to achieve the desired temperature.
- Steam Generation: Waste heat can be used to generate steam, which can then be used in processes that require heat, such as in food processing, chemical production, or power generation. This steam can also be used to drive turbines for electricity generation in co-generation systems.
- Space Heating: In industrial plants, especially those located in colder climates, recovered waste heat can be used for heating the plant itself or surrounding buildings. This is an energy-efficient way to meet heating needs without relying on external energy sources.
- Power Generation: High-temperature waste heat can be converted into electricity using technologies like steam turbines or ORC systems, which can then power the plant itself or be fed into the grid. This is particularly common in industries like metal production and cement manufacturing, which generate significant amounts of waste heat.
- Drying and Evaporation: In industries such as food processing, paper manufacturing, and chemical production, waste heat can be used in drying or evaporation processes, where heat is required to remove moisture from materials.
- Desalination: In regions where water scarcity is a concern, recovered waste heat can be used for desalination processes, helping to produce potable water from seawater or brackish sources.
Benefits of Waste Heat Recovery
- Energy Efficiency: Recovering waste heat improves the overall energy efficiency of industrial processes. Rather than losing heat to the environment, industries can reuse it, lowering the overall energy required to run their operations.
- Cost Savings: By recovering and reusing waste heat, industries can reduce their fuel consumption and energy costs. In some cases, the recovered energy can also be sold back to the grid or used to power other parts of the operation, creating an additional revenue stream.
- Reduced Emissions: Reducing the amount of energy consumed through waste heat recovery also leads to a reduction in carbon dioxide (CO2) emissions. This is especially important as industries face increasing pressure to meet environmental regulations and sustainability goals.
- Increased Operational Efficiency: The use of waste heat reduces the need for external heating and power generation, allowing industrial operations to become more self-sufficient and less reliant on outside energy sources.
- Compliance with Regulations: Many regions have strict energy efficiency and emissions standards that require industries to reduce their environmental impact. Waste heat recovery is one effective way for companies to comply with these regulations and avoid penalties.
Challenges and Considerations
- Initial Investment: Setting up waste heat recovery systems can involve significant capital expenditure, particularly for large or complex systems like HRSGs or ORC units. However, the long-term savings in energy costs and potential revenue generation can offset these initial costs.
- Heat Quality: The efficiency of waste heat recovery depends on the temperature and quality of the waste heat. Low-temperature waste heat is more difficult to recover and use efficiently, although technologies like ORC can help capture lower-temperature heat.
- Space and Infrastructure: Installing heat recovery systems often requires significant space and modifications to existing infrastructure, especially in facilities that were not originally designed for waste heat recovery. The integration of these systems into existing processes can be challenging.
- Maintenance: Like any system, waste heat recovery equipment requires regular maintenance to ensure it operates at peak efficiency. Failure to maintain these systems can lead to reduced performance and increased operational costs.
Conclusion
Industrial waste heat represents a largely untapped resource that, if recovered and reused, can greatly enhance energy efficiency, reduce operating costs, and minimize environmental impacts. The technologies available for recovering waste heat are diverse and can be applied to a wide range of industrial processes. As industries continue to face pressure to lower their energy consumption and reduce their carbon footprint, waste heat recovery is becoming an increasingly attractive solution for improving sustainability and meeting regulatory requirements. With advancements in heat recovery technologies and greater awareness of its potential, industrial waste heat recovery is poised to become a critical component of future energy-efficient industrial operations.
The integration of waste heat recovery into industrial operations is becoming more critical as industries strive to meet sustainability goals, reduce operational costs, and comply with increasingly stringent environmental regulations. Beyond simply improving energy efficiency, these systems can also have broader positive effects on the economy and the environment. As energy prices rise and concerns about climate change grow, industries that fail to invest in waste heat recovery may find themselves at a competitive disadvantage.
The potential for waste heat recovery in industrial settings is vast. For example, industries like glass manufacturing, petrochemicals, and refining produce waste heat at temperatures high enough to power turbines and generate electricity, which can then be used to offset energy consumption or even be sold back to the grid. This electricity generation can provide additional revenue or help reduce dependence on external power sources, further contributing to a plant’s overall energy independence.
Additionally, recovering waste heat offers a reliable and sustainable solution for industries looking to maintain constant energy supply without relying on external energy sources. For instance, using waste heat for power generation or district heating ensures that the energy produced is both efficient and consistent. This is particularly advantageous in industries that operate around the clock, such as cement production or steel manufacturing, where energy demand remains constant.
Another important benefit of waste heat recovery is the ability to enhance the sustainability profile of industries. By implementing heat recovery systems, companies can reduce their carbon footprint and greenhouse gas emissions. This is not just a matter of regulatory compliance, but also a growing consumer expectation. Businesses that prioritize sustainability and reduce their environmental impact tend to have a better reputation with both customers and investors. This can translate into improved market positioning, enhanced brand loyalty, and access to new financing opportunities, particularly in markets where sustainability practices are incentivized.
Industries can also consider alternative fuels as part of their waste heat recovery strategies. For example, utilizing biomass or biofuels in combination with recovered heat can further enhance energy efficiency and reduce reliance on fossil fuels. These renewable sources of energy can be integrated into existing systems to improve the sustainability of the entire operation. For instance, in an industry where biomass is readily available, it can be used in co-generation systems, reducing waste and further lowering carbon emissions.
While the technology and methods for waste heat recovery are continuously evolving, there are still challenges that need to be addressed. The upfront costs for installation and the need for specialized equipment can be significant, especially for smaller operations. However, with proper planning, detailed cost-benefit analysis, and support from governmental incentives or financing options, the long-term savings typically outweigh the initial costs. Furthermore, the development of modular and scalable systems has made waste heat recovery more accessible for industries of various sizes.
In the coming years, technological innovations in areas like digital controls, artificial intelligence, and machine learning will likely play a major role in optimizing waste heat recovery systems. Smart systems that monitor energy consumption and adjust heat recovery processes in real time can further improve the efficiency and effectiveness of these systems. As industries become more data-driven, these technologies will enable continuous improvements in energy management, making waste heat recovery an even more vital tool in achieving operational excellence.
Government policies and regulations are also likely to evolve, driving more industries to adopt waste heat recovery solutions. Governments across the world are setting ambitious climate goals, including carbon-neutrality targets and energy-efficiency improvements. In response, industries will be increasingly encouraged or required to recover waste heat to meet energy efficiency standards and lower emissions. Many governments already offer financial incentives, such as tax credits, grants, or subsidies, to encourage businesses to invest in energy-efficient technologies, including waste heat recovery systems.
Additionally, as industrial processes continue to diversify and evolve, new opportunities for waste heat recovery will emerge. For example, industries that are just beginning to adopt advanced technologies like 3D printing and additive manufacturing may discover novel ways to capture and utilize waste heat from their new processes. As industries move toward more complex and dynamic systems, the need for innovative solutions to harness and recover waste heat will grow.
One of the critical aspects of waste heat recovery is ensuring that the system is well-integrated into existing plant infrastructure. This requires careful planning, coordination, and expertise to ensure that the heat recovery system complements the existing process flow without causing disruptions. Successful integration also requires regular maintenance and monitoring to maintain peak efficiency. This is where system optimization becomes crucial. It’s not just about installing a heat recovery unit but about continuously assessing its performance and making adjustments as needed to ensure maximum energy savings.
Ultimately, the future of industrial waste heat recovery is optimistic. As industries continue to focus on energy efficiency, sustainability, and carbon reduction, waste heat recovery technologies will play an increasingly significant role in helping them meet these objectives. The technological innovations in this field, paired with government support and growing environmental awareness, make waste heat recovery one of the most promising strategies for industries looking to enhance their operations, save costs, and reduce their environmental impact.
The potential to recover industrial waste heat remains largely untapped in many sectors, and with the right infrastructure and commitment, industries have the opportunity to transform their operations into more sustainable, energy-efficient systems. Waste heat recovery is not just a technical upgrade—it’s an essential component of the broader transition toward a low-carbon economy and a more sustainable industrial future. By embracing these technologies, industries can significantly contribute to global energy conservation efforts while reaping substantial economic and environmental rewards.
As industries continue to face increasing pressure to adopt sustainable practices, the adoption of waste heat recovery systems offers a clear path to achieving significant energy savings while reducing environmental impact. Improved efficiency is one of the primary motivations for industries to invest in waste heat recovery technologies. In addition to reducing the amount of energy required for a particular process, recovering waste heat allows industries to reduce their reliance on external energy sources. This, in turn, helps lower energy costs and provides a more resilient energy supply, which is especially important in the face of fluctuating energy prices.
Moreover, waste heat recovery can significantly enhance process stability and reliability. For instance, recovered heat can be used to stabilize temperatures in specific parts of an industrial process, ensuring that products are consistently manufactured to the desired specifications. This is particularly beneficial in industries that require precise temperature control, such as pharmaceutical manufacturing or chemical production.
One key aspect to consider when implementing waste heat recovery systems is heat mapping. This process involves identifying all the potential sources of waste heat within a facility, from exhaust gases to cooling water, and determining the temperature and quantity of heat available for recovery. Once heat sources are identified, a thorough analysis can help determine the best recovery technologies and how to integrate them effectively into existing systems. Heat mapping enables industries to pinpoint the most cost-effective opportunities for waste heat recovery, ensuring that investments are optimized and yield the highest returns.
The scalability of waste heat recovery systems is another important factor. In some cases, industries may begin with small-scale heat recovery installations, particularly if their facilities are smaller or their energy needs are more modest. As energy demands grow, companies can scale up their systems to recover larger amounts of waste heat. This modularity ensures that companies can invest in heat recovery technologies incrementally without overwhelming their budgets or making too large a commitment upfront. Furthermore, as technology advances, newer, more efficient recovery systems can be added to existing setups, offering ongoing improvements in performance and cost-effectiveness.
Collaboration between different industries and sectors also plays a significant role in expanding the potential of waste heat recovery. For example, excess waste heat from one industrial process can be transferred to a neighboring facility that requires heat for its own operations. This type of industrial symbiosis, where companies share resources like heat, water, or energy, creates a more circular economy and reduces the total environmental impact. By working together, industries can optimize the use of available energy, reduce waste, and lower costs. This approach not only benefits individual companies but can also help create regional energy networks that improve energy resilience at the community or industrial park level.
The role of government incentives cannot be overlooked. In many regions, governments offer subsidies, tax breaks, or grants to encourage businesses to invest in energy-efficient technologies like waste heat recovery. These incentives can significantly offset the initial capital required to install heat recovery systems, making them more accessible for companies of all sizes. Governments also play an important role in setting energy efficiency standards and carbon reduction goals, which encourage industries to adopt sustainable practices. By creating a regulatory environment that supports energy efficiency, governments help ensure that the adoption of waste heat recovery becomes a long-term business strategy rather than a one-time project.
Another significant factor that supports the growth of waste heat recovery is the increased availability of energy storage solutions. Technologies like thermal storage systems can store recovered heat for later use, allowing companies to balance their energy supply with their demand more effectively. This is particularly useful for industries that experience fluctuations in energy needs throughout the day or year. For example, in seasonal manufacturing operations, where demand for energy is higher during certain months, thermal storage can help smooth out the peaks and valleys of energy usage, reducing reliance on external sources of power and lowering overall energy costs.
In parallel with traditional waste heat recovery methods, emerging technologies in heat transformation are opening up new possibilities for energy capture. For example, thermochemical processes, which use chemical reactions to capture and convert heat into usable forms of energy, are being explored as more efficient ways to harness low-temperature waste heat. These technologies are still in the research and development stages but hold the potential to revolutionize the way industries manage heat recovery.
As digitalization continues to transform industrial operations, the integration of smart sensors, real-time data analysis, and predictive maintenance systems into waste heat recovery setups will help companies maximize efficiency. These digital tools can provide insights into system performance, identify areas of inefficiency, and alert operators to potential issues before they become problems. This data-driven approach allows for continuous optimization of heat recovery systems, ensuring that they remain cost-effective and energy-efficient throughout their lifecycle.
In the long term, the successful integration of waste heat recovery systems will become a competitive advantage for industries. Companies that prioritize energy efficiency, sustainability, and cost reduction will not only improve their bottom line but also strengthen their market position. Investors and consumers are increasingly favoring businesses that demonstrate a commitment to environmental responsibility, and companies that leverage waste heat recovery will be better equipped to meet these expectations.
Ultimately, waste heat recovery is not just about reducing costs; it’s about contributing to a more sustainable and energy-efficient industrial ecosystem. By recovering and reusing heat, industries can lower their carbon footprints, reduce their reliance on fossil fuels, and make more responsible use of available resources. As technologies advance and the importance of sustainability becomes more ingrained in industrial practices, the potential for waste heat recovery will continue to grow, paving the way for greener, more efficient manufacturing processes across the globe. The widespread adoption of waste heat recovery is a fundamental step toward building a more sustainable industrial future, and its importance will only increase as the global economy shifts toward cleaner energy solutions.
Waste Heat Utilization
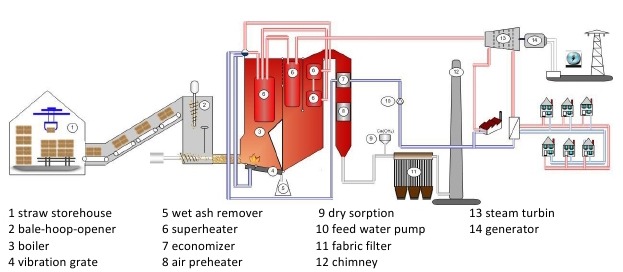
Waste heat utilization refers to the process of capturing and reusing thermal energy that would otherwise be lost during industrial or manufacturing processes. Instead of allowing this excess heat to be released into the environment, waste heat utilization focuses on converting it into useful energy or applying it in ways that contribute to the efficiency of operations. This practice is crucial in enhancing energy efficiency, lowering operating costs, and contributing to sustainability goals by reducing environmental impact.
Methods of Waste Heat Utilization
- Heat Recovery Systems: The most common approach to utilizing waste heat is through heat recovery systems. These systems can capture waste heat from hot gases, liquids, or surfaces and redirect it to useful purposes. Examples of heat recovery systems include:
- Heat exchangers: These devices transfer heat from one medium (e.g., exhaust gases) to another (e.g., water or air) without mixing them. The recovered heat can be used to preheat feedwater, combustion air, or other materials that require heating.
- Heat recovery steam generators (HRSGs): In co-generation or combined heat and power (CHP) systems, HRSGs capture heat from exhaust gases, typically from gas turbines or industrial furnaces, and use it to produce steam. This steam can then be used for power generation or process heating.
- Plate heat exchangers: These are compact devices that efficiently recover heat from both gaseous and liquid streams. They are widely used in industries like food processing, HVAC systems, and chemical manufacturing.
- Power Generation: One of the more advanced methods of utilizing waste heat is to convert it into electrical energy. There are several ways to do this:
- Organic Rankine Cycle (ORC): This technology uses a working fluid with a lower boiling point than water, making it ideal for low-temperature waste heat. The heat is used to vaporize the fluid, which then drives a turbine connected to a generator, producing electricity.
- Thermoelectric Generators (TEGs): TEGs convert heat directly into electricity using the Seebeck effect, which occurs when two different materials are joined together and subjected to a temperature difference. Although still relatively limited in industrial applications, TEGs have the potential to provide low-cost, small-scale power generation from waste heat.
- District Heating: Waste heat from industrial facilities can be captured and transported via a network of insulated pipes to nearby buildings or communities for space heating or hot water supply. This method is particularly useful in areas where industrial parks or clusters of manufacturing facilities are located near residential or commercial areas. By using recovered heat in this way, industries can reduce the demand for external heating sources, such as natural gas or electricity, while also reducing overall energy consumption in the community.
- Process Heat: Rather than converting waste heat into electricity, it can be utilized directly in various industrial processes. Some examples include:
- Preheating: Waste heat can be used to preheat incoming air, water, or raw materials. This reduces the need for additional heating, saving energy and lowering fuel consumption.
- Drying and Evaporation: In industries like food processing, paper manufacturing, and textiles, waste heat is often used in drying processes to remove moisture from products or materials. In chemical plants, it can also be used for evaporation purposes.
- Chemical Reactions: Some industries, especially in the chemical and petrochemical sectors, use waste heat to assist in endothermic reactions (reactions that require heat). For example, heat can be applied to distillation or absorption columns to help separate or purify materials.
- Absorption Cooling: This method uses waste heat to drive absorption chillers, which provide cooling. Absorption chillers use heat (instead of electricity) to drive a refrigeration cycle, making them particularly suited for industries that generate excess heat but also require cooling, such as in pharmaceutical production or HVAC systems. Absorption cooling systems can significantly reduce energy consumption compared to conventional compression refrigeration systems, as they do not rely on electricity to drive the cooling process.
- Thermal Storage: In cases where immediate utilization of waste heat is not necessary, thermal storage systems can be employed. These systems store heat for later use, enabling industries to recover waste heat during times of low demand and utilize it during peak demand periods. Molten salt storage systems are commonly used in this context, particularly in industries like metal smelting or power generation.
- Desalination: In water-scarce regions, waste heat can be utilized for desalination processes, which remove salts from seawater to produce potable water. Multi-effect distillation (MED) and multiple-effect desalination (MED) are common techniques that use waste heat to drive the evaporation and condensation processes necessary to purify seawater.
- Thermochemical Heat Storage: This innovative technology involves using chemical reactions to store heat. Thermochemical storage systems can store heat for extended periods, unlike traditional thermal storage systems, which are limited by heat loss. The stored heat can be released on demand, providing a continuous and reliable energy source. This is especially useful in industries where steady, on-demand heat is required, such as in the steel industry or for continuous chemical processes.
Benefits of Waste Heat Utilization
- Energy Efficiency: The most obvious benefit of utilizing waste heat is the improvement in overall energy efficiency. Rather than wasting energy by releasing it into the atmosphere or water bodies, industries can recover and reuse it for heating, cooling, or power generation. This helps reduce energy consumption and minimizes the need for external energy sources, which is both economically and environmentally beneficial.
- Cost Savings: Reusing waste heat can lead to significant cost savings by reducing the amount of fuel or energy required for manufacturing processes. Companies can also reduce their reliance on expensive electricity or gas, especially if they are located in regions with high energy costs. In some cases, recovered heat can be sold to neighboring facilities or communities, creating an additional revenue stream.
- Environmental Benefits: By recovering and reusing waste heat, industries can lower their carbon footprint and reduce greenhouse gas emissions. This is particularly important in industries that are large energy consumers, such as cement, steel, and petrochemical industries. The adoption of waste heat utilization helps businesses comply with environmental regulations and contribute to the broader effort to combat climate change.
- Reduced Fuel Consumption: Waste heat utilization reduces the need to burn additional fuel to generate heat or power. This is particularly beneficial in industries that rely on fossil fuels for heating or electricity generation. By making use of existing heat, industries can reduce their dependence on non-renewable energy sources and contribute to a more sustainable future.
- Improved Sustainability: By integrating waste heat recovery and utilization into their operations, companies can enhance their sustainability credentials. This is particularly important as consumers, investors, and governments increasingly favor businesses that take steps to reduce their environmental impact. Companies that adopt energy-efficient practices, such as waste heat utilization, often see improved reputation, stronger market positions, and increased access to green financing.
- Reduced Environmental Impact: By minimizing waste heat emissions to the atmosphere or water bodies, industries can reduce thermal pollution, which can have harmful effects on local ecosystems. Waste heat utilization systems can help minimize these impacts by either directly using the heat or converting it into useful energy, preventing it from contributing to environmental degradation.
Challenges and Considerations
- High Initial Investment: The installation of waste heat recovery and utilization systems often involves a significant upfront investment in equipment, infrastructure, and integration with existing systems. This can be a barrier for smaller companies or those with tight budgets, even though the long-term savings may outweigh these initial costs.
- Heat Quality and Temperature: The effectiveness of waste heat utilization depends on the temperature and quality of the waste heat. Low-temperature waste heat is harder to recover and use efficiently, which can require specialized technologies like organic Rankine cycles (ORC) or absorption chillers. Companies must carefully assess the temperature profile of waste heat sources to determine the most suitable recovery method.
- Integration with Existing Systems: Incorporating waste heat recovery and utilization systems into existing industrial operations requires careful planning and engineering. Retrofitting older plants with new heat recovery systems can be complex and may require significant modifications to infrastructure.
- Maintenance and Reliability: Like any energy recovery system, waste heat utilization equipment requires regular maintenance and monitoring to ensure that it continues to operate at peak efficiency. This can add to operational costs and require specialized personnel to manage.
- Space and Infrastructure Requirements: Installing waste heat recovery systems often requires additional space and infrastructure, which may not always be readily available in existing facilities. Industries may need to reconfigure their plant layouts or invest in new equipment to make use of recovered heat.
Conclusion
Waste heat utilization offers industries the opportunity to improve energy efficiency, reduce operational costs, and minimize environmental impact by converting waste heat into useful energy or directly applying it in manufacturing processes. With advancements in heat recovery technologies and increasing pressure on industries to reduce energy consumption and emissions, waste heat utilization is becoming an increasingly important strategy for achieving sustainability and cost competitiveness. However, challenges related to upfront costs, heat quality, and system integration must be carefully managed to fully realize the benefits of waste heat recovery. As technologies evolve and more industries adopt waste heat utilization practices, its role in improving energy efficiency and reducing industrial environmental footprints will continue to grow.
As industries continue to push for more sustainable and efficient operations, the role of waste heat utilization becomes even more critical. Beyond simply improving energy efficiency, harnessing waste heat helps industries meet their environmental goals, reduce their carbon footprint, and comply with energy efficiency regulations. The drive toward greater sustainability, combined with rising energy costs, makes waste heat recovery and reuse a fundamental strategy for companies looking to maintain competitiveness in a global market.
The ability to reuse waste heat not only cuts energy consumption but also provides a cost-effective way to reduce dependency on external energy sources. With energy prices fluctuating and concerns over energy security, companies that recover and reuse heat can provide a more resilient energy system for their operations. This increased energy independence can lead to more stable production processes, helping companies avoid disruptions due to energy shortages or price spikes.
The technology behind waste heat utilization continues to evolve, enabling industries to recover and use heat from lower temperature sources than previously possible. New heat exchange systems, organic Rankine cycles (ORC), and thermoelectric generators offer increasingly efficient ways to recover and convert waste heat into electricity or directly use it for heating purposes. These advancements are pushing the boundaries of what was once considered waste heat, allowing industries in a broader range of sectors to capture and utilize previously untapped energy.
One of the exciting developments in the field of waste heat utilization is the growth of smart technologies integrated into these systems. Sensors, data analytics, and predictive maintenance tools can optimize the performance of heat recovery units in real-time, allowing operators to fine-tune their operations and ensure maximum energy efficiency. These digital advancements are also reducing the risk of system downtime by alerting operators to potential issues before they result in costly repairs or production halts. This level of integration and automation is a game-changer for industries that rely on continuous, high-temperature processes such as cement production, metal smelting, and petrochemicals.
In addition to improving operational efficiency, waste heat utilization can open up new business models and revenue streams. For example, companies can sell excess heat to neighboring businesses or districts through district heating systems, turning waste heat into a source of income. This approach not only helps lower energy costs for recipients but also makes industrial operations more economically sustainable. Industries that engage in this practice may also benefit from collaborative initiatives between public and private sectors, such as energy sharing platforms, which promote the exchange of recovered energy within communities or industrial hubs.
Further, the integration of waste heat recovery into industrial ecosystems can lead to the creation of industrial symbiosis networks, where neighboring industries share not just energy but also resources like water or raw materials. By reducing waste and reusing heat across interconnected businesses, these networks contribute to a circular economy, where energy and materials flow more efficiently through the system. These types of collaborations are becoming more common, especially in regions with large industrial clusters, where the density of businesses offers significant opportunities for waste heat exchange.
The use of waste heat can also have a significant impact on resource conservation. By reducing the need for additional energy production, companies are not only conserving fuel but also contributing to the reduction of greenhouse gas emissions. This is particularly relevant as industries face increasing pressure to meet national and international climate goals. Reusing waste heat in manufacturing and industrial processes directly reduces emissions by reducing the need for external energy generation, particularly from fossil fuel-powered plants. Additionally, employing renewable technologies like solar thermal or geothermal systems alongside waste heat recovery further contributes to the decarbonization of industrial activities.
While the long-term benefits of waste heat utilization are substantial, there are still challenges that need to be addressed. The initial capital investment required to install and maintain waste heat recovery systems can be a significant barrier, particularly for small and medium-sized enterprises (SMEs). However, many governments offer financial incentives, grants, or subsidies to help offset the costs of implementing energy-saving technologies. These programs can make waste heat recovery technologies more accessible and reduce the financial burden on companies, encouraging more widespread adoption. Additionally, in some cases, the payback period for waste heat systems is relatively short, often just a few years, which makes the investment more attractive from a financial standpoint.
The success of waste heat utilization is also highly dependent on the specific needs and processes of each industry. For example, a steel plant may generate high-temperature waste heat that can be easily captured and used to produce electricity or preheat raw materials. In contrast, industries like food processing or textile manufacturing may generate lower-temperature waste heat that requires different technologies for effective recovery. Tailoring heat recovery systems to the unique characteristics of each industry ensures that the potential energy savings are maximized.
Looking forward, the ongoing development of low-temperature heat recovery technologies is likely to open up new possibilities for industries that generate relatively modest amounts of heat. As these technologies continue to improve, waste heat recovery will become a viable option for a wider range of sectors, including those that were previously unable to capture and reuse their waste heat efficiently. The growing emphasis on sustainability and energy efficiency is also likely to drive further innovation in heat recovery technologies, making them more accessible and cost-effective for all types of industrial operations.
The push for more energy-efficient and sustainable industries is not just a passing trend but a long-term shift toward a cleaner, more resilient global economy. Waste heat utilization is at the heart of this transformation, offering industries a tangible way to reduce their environmental impact while achieving substantial economic savings. Whether through heat recovery systems, power generation, or industrial symbiosis, the possibilities for utilizing waste heat are vast and varied. As industries continue to embrace these technologies, the future will see more energy-efficient, sustainable operations that rely less on external energy sources and contribute to a greener economy. The continued integration of waste heat utilization will be a key part of shaping a more sustainable industrial landscape for generations to come.
As industries push toward greater sustainability and energy efficiency, waste heat utilization will play an increasingly pivotal role. Beyond just economic and environmental benefits, utilizing waste heat will also help industries stay competitive in a rapidly evolving global market. With rising energy costs, stricter environmental regulations, and greater public demand for sustainability, waste heat recovery systems provide a viable solution for businesses to maintain cost-effectiveness while meeting their carbon reduction targets.
Innovative partnerships and collaborative efforts between industries, municipalities, and research institutions will further accelerate the adoption of waste heat utilization technologies. In some regions, industrial parks and clusters are already benefiting from shared waste heat infrastructure, where heat from one facility is transferred to others through district heating networks. This approach maximizes the value of waste heat by allowing multiple industries to benefit from it, rather than letting it go unused. This collaborative model can be particularly effective in dense industrial zones or regions where energy infrastructure is shared across multiple sectors, promoting local energy resilience and reducing dependency on external energy sources.
As the technology landscape evolves, advanced materials and nano-technologies are also emerging as key players in improving heat recovery systems. For example, nanostructured thermoelectric materials are being explored for their ability to capture low-grade heat and convert it into electricity with higher efficiency than traditional thermoelectrics. This would significantly expand the types of waste heat that could be harnessed, including lower-temperature sources like those found in industrial refrigeration, cooling systems, or certain chemical manufacturing processes. As these technologies mature, industries will have access to even more efficient and cost-effective ways to recover heat from processes that were previously considered too low-grade or inefficient for energy recovery.
Energy storage technologies, like thermal energy storage (TES) and phase change materials (PCMs), will also play a crucial role in enhancing the effectiveness of waste heat utilization. Thermal storage can store excess heat when it is available and release it when demand is high or when it is needed for peak-load heating. This can significantly increase the flexibility and reliability of energy systems, particularly in industries with fluctuating energy demands. Additionally, combining heat storage with renewable energy sources like solar or wind can create highly efficient, hybrid systems that integrate waste heat recovery with intermittent energy generation, further boosting energy independence and reliability.
Regulatory and policy frameworks are expected to evolve in parallel with technological advancements. Governments and international organizations are increasingly setting ambitious energy efficiency and climate goals, pushing industries to reduce emissions and waste. This creates both opportunities and pressures for industries to invest in waste heat recovery systems. Policies that incentivize the adoption of energy-saving technologies, such as grants, tax rebates, or favorable financing options, will continue to encourage companies to integrate waste heat recovery into their operations. Furthermore, companies that lead in adopting energy-efficient solutions will benefit from enhanced corporate reputations, which can increase their appeal to investors, customers, and regulatory bodies.
Looking forward, smart technologies will transform the way waste heat recovery systems operate. The integration of artificial intelligence (AI), machine learning, and internet of things (IoT) technologies into heat recovery units will enable real-time monitoring, predictive analytics, and adaptive control systems that optimize performance. These digital tools will help industries continuously adjust and improve their heat recovery processes, ensuring maximum efficiency and minimizing downtime. This approach, which combines data-driven insights with real-time system optimization, will help companies avoid operational inefficiencies and ensure the most effective use of recovered heat.
The importance of waste heat recovery will also continue to grow as industries face increased scrutiny over their environmental impacts. As consumer demand for green products rises and companies are held accountable for their sustainability efforts, waste heat recovery will become a critical component of corporate social responsibility (CSR) strategies. Businesses that adopt waste heat utilization technologies are not only reducing their operational costs but also positioning themselves as leaders in the green economy, which is becoming a key factor in gaining market share.
Furthermore, the concept of circular economy will be advanced by waste heat utilization. By effectively managing energy waste and reusing it in other parts of the production process, industries can close the loop on their resource use. This will help in minimizing resource depletion and environmental degradation while promoting more sustainable business models. Companies that embrace circular economy principles, including the recovery and reuse of waste heat, will be better prepared to meet both market demands and regulatory requirements for sustainability.
As we look ahead, the increasing availability of low-carbon technologies, coupled with sustainability-driven regulations, will drive industries towards more comprehensive energy management strategies. Waste heat recovery will be integrated into broader energy optimization programs that include renewable energy sources, energy storage, and efficiency measures. These comprehensive strategies will not only help industries reduce their energy consumption and costs but will also contribute significantly to the decarbonization of global industries.
The role of waste heat utilization will continue to evolve, providing industries with innovative solutions to reduce costs, improve sustainability, and achieve greater energy independence. As technology advances and industry-specific applications become more refined, the potential for waste heat recovery will only expand. Ultimately, the widespread adoption of waste heat utilization technologies will play a crucial role in transitioning to a more energy-efficient, circular, and sustainable industrial economy, where both businesses and the environment can thrive.
Waste Heat Recovery for Maritime Applications
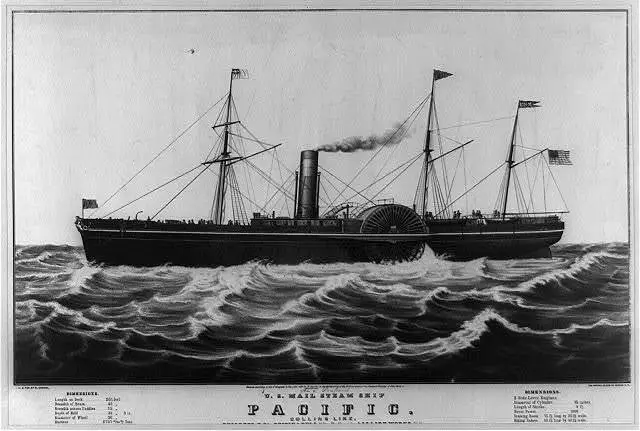
Waste heat recovery for maritime applications is an increasingly important aspect of improving the energy efficiency and environmental sustainability of the shipping industry. Ships are known to consume large amounts of fuel, and a significant portion of this fuel’s energy is lost as waste heat through exhaust gases, engine cooling, and other processes. Recovering and utilizing this waste heat can significantly improve the overall efficiency of vessels, reduce fuel consumption, lower greenhouse gas emissions, and help shipping companies meet regulatory standards.
Key Areas of Waste Heat Recovery in Maritime Applications:
- Exhaust Gas Heat Recovery Systems (EGHR): One of the most common applications of waste heat recovery on ships is the recovery of energy from the hot exhaust gases produced by the main engine. Exhaust gas heat recovery systems (EGHR) capture and transfer the heat from exhaust gases into a useful form, typically as steam or hot water. This recovered energy can be used for various onboard purposes:
- Preheating fuel or air entering the engine, reducing the need for additional fuel consumption.
- Generating steam for propulsion or auxiliary systems, such as heating, air conditioning, and even desalination.
- Power generation through steam turbines or organic Rankine cycle (ORC) systems, where the heat can drive a turbine to produce electricity.
- Heat Recovery Steam Generators (HRSGs): A more advanced version of exhaust gas heat recovery is the heat recovery steam generator (HRSG), which can recover waste heat from both the main engine and auxiliary engines. HRSGs generate steam, which can then be used for propulsion (in a steam turbine), electricity generation, or other applications such as heating and desalination. HRSGs are particularly useful for large vessels and are often integrated into more energy-efficient ship designs.
- Organic Rankine Cycle (ORC): The Organic Rankine Cycle is a technology that uses a low-boiling-point organic fluid instead of water. The fluid absorbs heat from exhaust gases or other waste heat sources, turning into vapor that drives a turbine to generate electricity. ORCs are well-suited to maritime applications because they can operate efficiently at the lower temperature waste heat levels often found on ships. By recovering waste heat in this way, vessels can reduce fuel consumption and emissions, while also generating power for auxiliary systems or propulsion.
- Exhaust Gas Economizers: Exhaust gas economizers are used to recover heat from the exhaust gases of large marine engines. They function as heat exchangers, transferring the heat from exhaust gases to feedwater, which is then used to generate steam. The economizer can preheat water before it enters the boiler, improving efficiency and reducing fuel consumption. Economizers are typically used on large vessels like tankers, container ships, and cruise ships, where fuel savings can be substantial.
- Waste Heat Recovery for Refrigeration: Waste heat recovery can also be used to support refrigeration on board ships, which is crucial for transporting perishable goods. The recovered heat can be used to run absorption refrigeration systems or other cooling technologies. By utilizing waste heat to drive the cooling process, vessels can reduce their reliance on traditional fuel-driven refrigeration systems, thereby reducing fuel consumption and operational costs.
- Combined Heat and Power (CHP): The integration of Combined Heat and Power (CHP) systems on ships allows for the simultaneous generation of electricity and heat from a single fuel source, typically using waste heat from the ship’s engine or exhaust. CHP systems are designed to capture waste heat and convert it into useful energy for the vessel, increasing overall energy efficiency. In maritime applications, this could involve using recovered heat to generate electricity for onboard systems, such as lighting, air conditioning, and desalination, as well as thermal energy for heating and hot water.
- Heat Recovery for Freshwater Production (Desalination): Onboard desalination systems, used to convert seawater into freshwater for consumption, can be powered by recovered waste heat. Specifically, multi-effect distillation (MED) or multi-stage flash (MSF) desalination processes can be driven by waste heat from the engine or exhaust gases. These methods allow vessels to produce freshwater with minimal additional energy input by utilizing the thermal energy already available. By using waste heat in this way, vessels can reduce their reliance on fuel for desalination, improving overall fuel efficiency and lowering operating costs.
- Thermal Energy Storage Systems: Ships are typically subject to varying heat demands depending on the operation conditions, and thermal energy storage (TES) systems can help manage this variability. Phase change materials (PCMs) or other storage solutions can be used to store excess waste heat generated during peak engine loads or when the vessel is operating at high speeds. This stored energy can then be used during periods of lower engine output or when additional heating is required, such as during overnight operations or when the ship is idle.
- Waste Heat Recovery for Auxiliary Power Generation: On many vessels, there are separate auxiliary engines that provide power for onboard systems like lighting, refrigeration, and air conditioning. Waste heat from these auxiliary engines can be captured and used to generate additional power, reducing the need for external fuel consumption. By integrating waste heat recovery with auxiliary systems, ships can achieve significant fuel savings, improve operational efficiency, and lower their environmental impact.
Environmental Benefits:
The maritime industry is under growing pressure to meet international environmental regulations, including the International Maritime Organization’s (IMO) Emission Control Areas (ECAs) and the IMO 2020 sulfur cap, which mandates a reduction in sulfur emissions from ship exhaust. Waste heat recovery systems help vessels comply with these regulations by improving energy efficiency, which in turn reduces the carbon emissions and air pollutants released into the atmosphere.
- Reduction in CO2 emissions: By recovering and utilizing waste heat, ships can reduce their overall fuel consumption, leading to lower carbon emissions.
- Reduction in particulate matter and sulfur emissions: Some waste heat recovery technologies, such as exhaust gas economizers and ORC systems, can help reduce the need for burning additional fuel, which in turn reduces the amount of harmful particulate matter and sulfur oxides released from ships.
- Compliance with emission regulations: Waste heat recovery systems can help vessels meet stringent emissions standards and avoid penalties, all while improving operational efficiency.
Economic Benefits:
The initial installation costs for waste heat recovery systems on ships can be high, but the long-term savings in fuel and operational costs make these systems an attractive investment.
- Fuel savings: By recovering waste heat and reusing it for auxiliary systems, propulsion, or power generation, ships can significantly reduce fuel consumption. These savings are especially valuable for vessels operating in energy-intensive sectors, such as bulk carriers, oil tankers, and container ships, where fuel costs are a significant portion of overall operating expenses.
- Lower operating costs: In addition to reducing fuel consumption, waste heat recovery systems can help reduce the wear and tear on engines and other mechanical systems, leading to fewer repairs and lower maintenance costs.
- Return on investment: Over time, the savings generated from reduced fuel consumption, lower emissions, and improved operational efficiency can provide a substantial return on investment for shipping companies.
Challenges and Considerations:
Despite the many benefits of waste heat recovery for maritime applications, there are several challenges and considerations to keep in mind:
- Space limitations: Ships have limited space, and the installation of waste heat recovery systems requires careful planning to ensure that they can be integrated into the existing ship design without compromising safety, efficiency, or cargo capacity.
- Capital costs: The initial investment in waste heat recovery technologies can be significant. Shipping companies must carefully evaluate the potential savings and payback period to determine whether the investment is worthwhile.
- Maintenance and operational complexity: Advanced waste heat recovery systems require ongoing maintenance and monitoring to ensure optimal performance. Ship crews must be trained to operate and maintain these systems properly, which can add to operational costs.
- Integration with existing systems: Integrating waste heat recovery systems into older vessels can be more challenging than installing them on new ships designed with these systems in mind. Retrofitting existing ships may require more extensive modifications to the vessel’s infrastructure, which can increase costs and downtime.
Conclusion:
Waste heat recovery for maritime applications is a key strategy for improving energy efficiency, reducing fuel consumption, and meeting environmental regulations. By harnessing the power of waste heat from exhaust gases, engine cooling, and auxiliary systems, ships can significantly reduce their reliance on external energy sources, lower operating costs, and contribute to a cleaner, more sustainable shipping industry. With advances in heat recovery technologies, such as organic Rankine cycles, combined heat and power systems, and exhaust gas economizers, the potential for energy savings in the maritime industry is vast. Although there are challenges related to space, installation costs, and maintenance, the long-term benefits of waste heat recovery make it an increasingly attractive option for shipping companies looking to improve operational efficiency, reduce environmental impact, and stay competitive in an evolving global market.
Waste heat recovery in maritime applications has become an essential strategy for improving energy efficiency, reducing fuel consumption, and minimizing the environmental impact of ships. As the shipping industry continues to face pressure to meet stringent environmental regulations, there is growing recognition of the value of harnessing waste heat to drive down emissions and improve operational performance. With advancements in technologies, vessels are now able to capture and reuse heat from various sources, such as exhaust gases, engine cooling, and auxiliary systems, which were previously wasted.
One of the most significant advantages of waste heat recovery is the potential for fuel savings. The process of capturing exhaust gases’ heat and converting it into useful energy allows ships to reduce their overall fuel consumption. This is particularly important in an industry where fuel costs are one of the largest operational expenses. By using the recovered heat to generate electricity, produce steam for propulsion, or run auxiliary systems, ships can operate more efficiently and significantly lower their fuel usage. These savings have a direct economic benefit, helping companies reduce operational costs and improve their bottom line.
In addition to the fuel savings, the reduction in fuel consumption leads to lower carbon emissions. With international regulations tightening on sulfur emissions, including the IMO 2020 sulfur cap, vessels equipped with waste heat recovery systems can reduce their reliance on high-sulfur fuels, thus meeting regulatory requirements. Moreover, waste heat recovery systems help to reduce greenhouse gas emissions and other pollutants, making ships more compliant with Emission Control Areas (ECAs) and other regional standards.
The technology involved in waste heat recovery is continually evolving, with innovations making it easier for ships to integrate these systems. For example, the Organic Rankine Cycle (ORC) technology is gaining traction in maritime applications because it can efficiently convert lower-temperature waste heat into electricity, making it well-suited for the relatively modest waste heat levels found on most ships. Similarly, Combined Heat and Power (CHP) systems offer dual benefits, generating electricity for onboard systems while using the recovered waste heat for heating, hot water, and even freshwater production.
Another growing trend is the use of exhaust gas economizers. These devices capture heat from exhaust gases and use it to preheat feedwater or generate steam. They are especially useful on large vessels that rely on both main and auxiliary engines. The integration of economizers into a ship’s existing systems can significantly enhance fuel efficiency, as they ensure that waste heat is put to good use rather than venting into the atmosphere.
In addition to generating power and reducing emissions, waste heat recovery can also support onboard cooling and refrigeration systems. For example, absorption chillers, which use heat to drive a cooling cycle, can be powered by recovered waste heat instead of additional fuel. This helps to reduce the amount of energy required for maintaining refrigerated cargo or for air conditioning in passenger vessels. In this way, waste heat recovery not only improves the efficiency of the vessel but also supports the operation of critical systems, such as temperature control for sensitive cargo.
The adoption of waste heat recovery technologies is also a step toward sustainable practices in the maritime industry. The industry is under increasing scrutiny from environmental groups and regulatory bodies, pushing for sustainability and reduced emissions. As a result, shipping companies are seeking solutions that help reduce their environmental impact and align with the growing demand for green shipping. Integrating waste heat recovery aligns with these goals by improving energy efficiency and lowering the carbon footprint of maritime operations.
However, the implementation of waste heat recovery systems is not without challenges. One of the primary concerns is the capital investment required to install and retrofit vessels with such technologies. While the long-term savings in fuel and operational costs can provide a solid return on investment, the upfront costs can be significant, particularly for older vessels. Moreover, the integration of these systems into existing ships requires careful planning, as retrofitting must not compromise the vessel’s safety or performance. Space limitations aboard ships can also be a barrier, as these systems often require specialized equipment and installations that may take up valuable space on board.
Additionally, maintenance and the technical complexity of these systems need to be considered. Waste heat recovery systems, such as organic Rankine cycles or steam generators, require ongoing maintenance and monitoring to ensure optimal performance. Regular servicing is needed to prevent malfunctions and ensure the systems continue to operate efficiently over time. Crews must be properly trained to operate and maintain these advanced technologies, which can lead to higher operational costs and resource requirements in the short term.
Despite these challenges, the benefits of waste heat recovery systems far outweigh the costs in the long run. Shipping companies that invest in these technologies not only reduce their environmental footprint but also improve the sustainability of their operations. Furthermore, the growing emphasis on green technologies and renewable energy in the shipping industry is likely to spur further innovations in waste heat recovery systems. As the industry continues to evolve and faces stricter environmental regulations, these technologies will become even more critical in helping companies stay competitive and meet their regulatory obligations.
The future of waste heat recovery in maritime applications looks promising, especially with the continued development of more efficient systems and the increasing cost-effectiveness of these technologies. As digitalization and smart systems become more common in the maritime industry, the ability to monitor, control, and optimize the performance of waste heat recovery systems in real-time will become more prevalent. The use of data analytics, IoT, and artificial intelligence (AI) will allow operators to fine-tune these systems for maximum efficiency, minimizing fuel consumption and emissions while extending the lifespan of the systems themselves.
Ultimately, waste heat recovery in maritime applications not only improves the operational efficiency of vessels but also contributes to the greening of the shipping industry as a whole. The long-term impact will be significant, with substantial reductions in fuel consumption, emissions, and operating costs. As the shipping industry continues to evolve, waste heat recovery will undoubtedly play a pivotal role in achieving sustainability goals and improving energy efficiency, ensuring that ships can continue to meet the demands of global trade while reducing their environmental impact.
Looking ahead, the integration of waste heat recovery technologies will continue to advance as part of a broader trend towards energy-efficient and eco-friendly solutions in the maritime sector. The global push for decarbonization and sustainable practices will further accelerate the adoption of waste heat recovery systems. Vessels will increasingly rely on a mix of renewable energy sources, energy storage, and heat recovery systems to reduce their carbon footprint and reliance on fossil fuels.
One promising development is the hybridization of waste heat recovery systems with renewable energy sources. Ships equipped with solar panels or wind turbines could complement waste heat recovery systems, creating a hybrid energy solution. For instance, energy recovered from exhaust gases could be used to supplement the power generated by renewable sources, providing a more stable and reliable energy supply for onboard systems. This approach not only reduces the vessel’s environmental impact but also increases its energy independence, making it less reliant on external fuel sources.
Additionally, advanced materials and nano-technology are being explored to enhance the efficiency of waste heat recovery systems. New heat exchangers made from materials that better conduct heat or have superior thermal properties could increase the effectiveness of recovery systems. Thermoelectric materials, which convert heat into electricity, are also being researched for their ability to capture lower-temperature waste heat and convert it into usable power. These materials could potentially be used in combination with other heat recovery technologies, expanding the range of waste heat sources that can be utilized on vessels.
Fuel cells and battery-powered vessels are also gaining traction as alternatives to conventional marine engines. The combination of waste heat recovery with hydrogen fuel cells or battery storage could further improve the efficiency and sustainability of maritime operations. In this scenario, recovered heat could be used to drive fuel cell systems, or to assist with charging batteries for auxiliary power, further reducing the need for traditional fuels and enhancing overall vessel performance.
The concept of zero-emission ships is becoming more realistic with the integration of waste heat recovery and renewable energy technologies. As the maritime industry faces the challenge of meeting international emission reduction targets, such as those set by the Paris Agreement, waste heat recovery systems will play a crucial role in helping ships reduce their reliance on fossil fuels. This transition to zero-emission vessels may also be supported by stricter regulations and incentives for companies that adopt green technologies. For example, some countries and regions are already offering tax breaks, grants, or other incentives for companies investing in energy-efficient technologies or reducing emissions from their fleets.
As waste heat recovery becomes more common, collaborations between technology providers, shipbuilders, and maritime operators will foster innovation and drive the development of more efficient and cost-effective solutions. Research institutions, for example, could develop new heat recovery systems tailored to specific maritime needs, while shipyards will incorporate these systems into new vessel designs. In some cases, partnerships between shipping companies and energy providers could lead to the development of integrated waste heat recovery systems that share energy across fleets or ports, further boosting efficiency.
In the future, digitalization and smart technologies will play an increasingly significant role in waste heat recovery systems. Sensors, AI-driven analytics, and real-time monitoring will enable operators to track the performance of waste heat recovery systems more precisely. By using these technologies, ships can optimize the recovery and use of heat in real time, ensuring that energy is being used as efficiently as possible. Predictive maintenance powered by AI could help prevent system failures, reduce downtime, and extend the lifespan of the equipment.
Environmental and economic pressures will continue to shape the maritime industry’s shift towards waste heat recovery. With the demand for low-carbon transportation increasing across sectors, waste heat recovery will not only help reduce the environmental impact of shipping but will also provide shipping companies with a competitive advantage in a market that increasingly values sustainability. Vessels that can operate more efficiently and with a smaller environmental footprint will be better positioned to meet customer expectations, avoid penalties, and thrive in a decarbonized future.
The potential for waste heat recovery in maritime applications is vast, and its benefits will only continue to grow as technologies evolve. By reducing fuel consumption, lowering emissions, and improving operational efficiency, waste heat recovery systems will be integral to shaping the future of sustainable shipping.
Waste Heat Recovery Boiler Working Principle
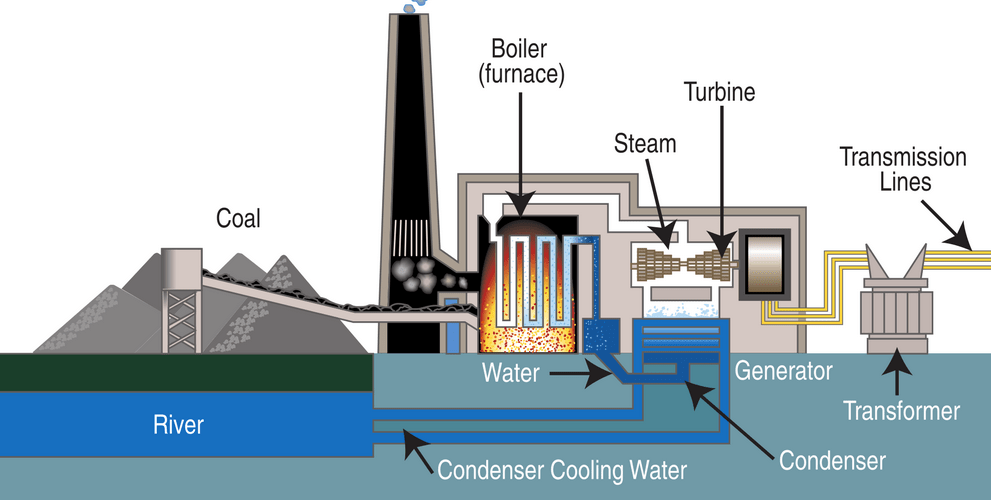
A Waste Heat Recovery Boiler (WHRB) is a system designed to capture and utilize heat that would otherwise be lost in industrial processes, such as exhaust gases from turbines, engines, or furnaces. This recovered heat is then used to generate steam or hot water, which can be utilized for power generation, heating, or other industrial applications.
Working Principle of a Waste Heat Recovery Boiler
- Heat Source Collection
- The waste heat recovery boiler is connected to an industrial process that produces hot exhaust gases, such as a gas turbine, diesel engine, incinerator, or furnace.
- These gases, which would normally be vented into the atmosphere, contain significant thermal energy that can be reused.
- Heat Transfer to Boiler Tubes
- The hot gases pass through a set of heat exchanger tubes in the boiler.
- Heat from the gases is transferred to the boiler tubes, which contain feedwater.
- Depending on the design, the WHRB can operate as a fire-tube boiler (hot gases pass through tubes surrounded by water) or a water-tube boiler (water flows through tubes heated externally by the hot gases).
- Steam Generation
- As heat is transferred from the hot exhaust gases to the water, the temperature of the water rises, eventually converting it into steam.
- The generated steam can then be used in various ways:
- Power generation: The steam is sent to a steam turbine to produce electricity.
- Process heating: The steam is used for industrial heating applications.
- District heating: It may supply heat to buildings or other facilities.
- Exhaust Gas Exit
- After transferring most of its heat to the boiler tubes, the cooled exhaust gas exits the boiler through a chimney or stack.
- In some systems, an economizer or air preheater is installed to extract even more heat before the gases are finally released.
- Condensate Return and Reuse
- The steam produced is utilized in the process, and the condensed steam (condensate) is returned to the boiler to be reheated, forming a closed-loop system for efficiency.
Advantages of Waste Heat Recovery Boilers
- Fuel Savings: By using waste heat, the need for additional fuel is reduced.
- Lower Emissions: Reduces carbon footprint by maximizing energy efficiency.
- Increased Energy Efficiency: Enhances overall plant efficiency by utilizing otherwise wasted energy.
- Cost Reduction: Reduces fuel costs by using available waste heat.
Waste heat recovery boilers are widely used in power plants, refineries, steel mills, cement plants, and marine applications, helping industries improve efficiency and sustainability while reducing operational costs.
A waste heat recovery boiler operates by capturing thermal energy from exhaust gases generated in industrial processes and using it to produce steam or hot water. The process begins with the collection of high-temperature exhaust gases from sources such as gas turbines, engines, furnaces, or incinerators. Instead of allowing these gases to dissipate into the atmosphere as waste, the boiler directs them through a series of heat exchanger tubes designed to transfer heat efficiently. As the hot gases flow over or through the boiler tubes, they transfer their heat to the water or feedwater circulating within the system.
The transfer of heat raises the temperature of the water, eventually generating steam. This steam can then be utilized for various applications, including power generation by driving a steam turbine, providing heat for industrial processes, or serving as a heating source in district heating systems. Depending on the system design, the waste heat recovery boiler can function as a fire-tube boiler, where hot gases pass through tubes surrounded by water, or a water-tube boiler, where water flows through tubes exposed to hot gases.
Once the heat is extracted, the now-cooled exhaust gases exit the boiler through a chimney or stack. To maximize efficiency, some systems incorporate additional heat recovery devices such as an economizer, which preheats incoming feedwater, or an air preheater, which warms combustion air before entering the main process. These enhancements further reduce energy losses and improve the overall efficiency of the system.
The condensed steam, after use in its designated application, is often returned to the boiler as feedwater in a closed-loop cycle, improving efficiency and minimizing water waste. The integration of waste heat recovery boilers offers significant advantages, including reduced fuel consumption, lower greenhouse gas emissions, and overall cost savings. By utilizing waste heat that would otherwise be lost, industries can improve their energy efficiency while reducing environmental impact. Waste heat recovery boilers are widely used in power plants, refineries, steel mills, cement plants, and maritime applications, playing a critical role in sustainable energy management.
Waste heat recovery boilers are designed to operate continuously, maximizing energy utilization from industrial processes. Their effectiveness depends on several factors, including the temperature and volume of the exhaust gases, the heat exchanger design, and the system’s overall integration with plant operations. In many industries, the recovered steam is used directly in production processes, helping to reduce dependence on primary fuel sources. This is particularly beneficial in energy-intensive sectors such as petrochemicals, steel manufacturing, and cement production, where high-temperature waste heat is abundant.
One of the key aspects of waste heat recovery boilers is their adaptability to different operating conditions. Some systems are designed to handle varying loads and fluctuating temperatures, ensuring stable and efficient steam generation even when waste heat availability changes. This flexibility makes them ideal for applications where process conditions are not constant, such as cogeneration plants, where both electricity and thermal energy are required. In these setups, the steam produced can either be used for direct heating or converted into electrical power using a steam turbine, improving overall plant efficiency.
Advanced waste heat recovery boilers incorporate features like modular construction, which allows for easier installation and scalability. Some modern systems use combined heat and power (CHP) technology, integrating waste heat recovery with additional energy conversion systems to optimize fuel usage. Additionally, the use of digital monitoring and automation enhances performance by continuously adjusting operations based on real-time data. Smart controls and predictive maintenance reduce downtime and improve efficiency by detecting potential issues before they cause significant disruptions.
As industries move toward greener energy solutions, waste heat recovery boilers are playing a vital role in reducing carbon emissions. Regulatory frameworks and sustainability goals are pushing companies to adopt energy-efficient technologies, making waste heat recovery a strategic investment for long-term operational savings and environmental benefits. With advancements in materials and heat transfer technology, future developments will continue to improve the efficiency and durability of these systems, further increasing their adoption across industries. The ongoing transition toward net-zero emissions and sustainable industrial practices ensures that waste heat recovery boilers will remain a critical component of energy-efficient plant operations.
Waste Heat Recovery Boiler
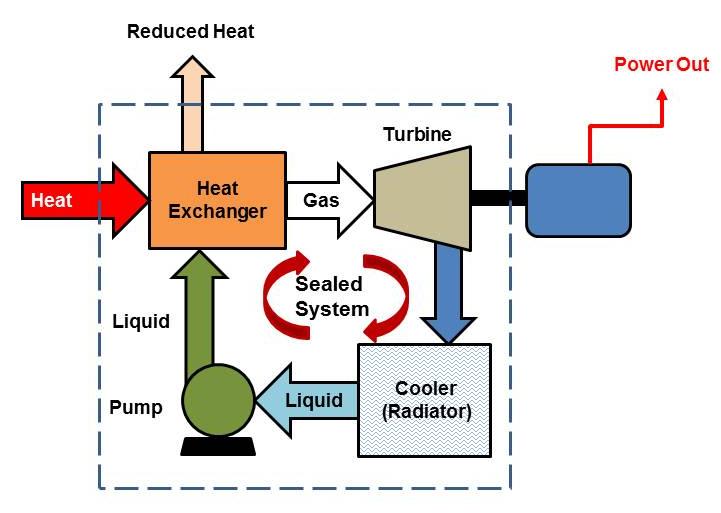
A Waste Heat Recovery Boiler (WHRB) is a specialized boiler that captures and utilizes the thermal energy from exhaust gases or other waste heat sources in industrial processes. Instead of allowing this heat to dissipate into the atmosphere, the WHRB transfers it to water, generating steam or hot water for further use. This process significantly improves energy efficiency, reduces fuel consumption, and lowers emissions, making it an essential component in energy-intensive industries such as power plants, refineries, steel mills, cement production, and maritime applications.
Working Principle of a Waste Heat Recovery Boiler
The operation of a WHRB revolves around heat exchange between hot exhaust gases and water within the boiler system. The process begins when waste heat from engines, turbines, or furnaces is directed through a series of heat exchanger tubes. As these hot gases flow over the tubes, they transfer thermal energy to the water inside, causing it to heat up and eventually convert into steam. This steam can then be utilized for various purposes, such as driving steam turbines for electricity generation, supplying heat for industrial processes, or contributing to district heating systems.
After the heat transfer process, the cooled exhaust gases exit the boiler through a stack or chimney. To maximize efficiency, many WHRB systems incorporate additional heat recovery components such as economizers (which preheat the feedwater) and air preheaters (which warm the combustion air before it enters the furnace). These enhancements further reduce energy losses and improve overall plant efficiency.
Types of Waste Heat Recovery Boilers
WHRBs can be classified based on their design and application:
- Fire-Tube WHRB: Hot exhaust gases pass through tubes surrounded by water, transferring heat to the water and generating steam.
- Water-Tube WHRB: Water flows through tubes while hot gases pass over them, allowing for efficient heat transfer, particularly in high-pressure applications.
- Once-Through WHRB: Used in power generation, these boilers operate without a drum, allowing continuous steam flow.
- Supplementary-Fired WHRB: Includes additional burners to increase steam production when waste heat alone is insufficient.
Advantages of Waste Heat Recovery Boilers
- Energy Efficiency: Utilizes otherwise wasted heat to generate steam, improving overall plant efficiency.
- Fuel Savings: Reduces the need for additional fuel consumption, leading to cost savings.
- Lower Emissions: By reducing fuel usage, WHRBs help lower greenhouse gas emissions.
- Versatility: Can be integrated into various industrial processes, power plants, and marine applications.
- Sustainability: Supports environmental regulations and sustainability initiatives by reducing energy waste.
Waste heat recovery boilers play a crucial role in optimizing energy use across industries, providing both economic and environmental benefits. As industries continue to focus on energy efficiency and emission reduction, the adoption of WHRBs is expected to grow, driven by technological advancements and regulatory requirements.
A Waste Heat Recovery Boiler (WHRB) is a key energy-saving technology that captures and utilizes heat energy from exhaust gases generated in industrial processes, reducing fuel consumption and increasing overall efficiency. Instead of releasing hot gases into the atmosphere, the system directs them through a heat exchanger, where they transfer thermal energy to water, producing steam or hot water that can be used for power generation, heating, or other industrial applications. The design of a WHRB depends on factors such as the temperature and volume of the waste heat source, process requirements, and available space, with common configurations including fire-tube, water-tube, once-through, and supplementary-fired designs.
The fundamental working principle of a WHRB involves heat exchange between high-temperature exhaust gases and water inside the boiler tubes. As the gases pass through, they transfer heat to the water, converting it into steam that can be used to drive a steam turbine for power generation, provide process heat, or supply district heating systems. To improve efficiency, many WHRBs incorporate additional components such as economizers, which preheat the feedwater using residual heat, and air preheaters, which warm the combustion air to enhance fuel efficiency. The exhaust gases, once they have transferred most of their heat, are released through a stack or chimney.
Industries such as power plants, steel manufacturing, cement production, petrochemicals, and refineries benefit significantly from WHRBs as they help lower fuel costs and reduce greenhouse gas emissions. The ability to recover waste heat makes these boilers an essential part of energy conservation strategies, particularly in industries with high-temperature exhaust streams. In cogeneration plants, WHRBs enable combined heat and power (CHP) applications, improving overall system efficiency by producing both electricity and useful thermal energy. In marine applications, they contribute to the energy efficiency of ships by utilizing heat from engine exhaust to generate steam, reducing the need for additional fuel consumption.
Advancements in WHRB technology continue to drive improvements in efficiency and durability, with modern systems incorporating digital monitoring and automation to optimize performance. Predictive maintenance, enabled by sensors and data analytics, helps reduce downtime and improve operational reliability. The adoption of waste heat recovery technology is also supported by stricter environmental regulations and sustainability goals, encouraging industries to minimize waste and reduce their carbon footprint. As companies seek to optimize energy use and comply with emission reduction targets, the role of WHRBs in sustainable industrial operations is becoming increasingly important.
As industries continue to prioritize energy efficiency and sustainability, Waste Heat Recovery Boilers (WHRBs) are becoming an integral part of modern industrial processes. These systems are designed to maximize energy utilization by capturing and repurposing waste heat that would otherwise be lost, significantly reducing operational costs and environmental impact. The effectiveness of a WHRB depends on factors such as the temperature and composition of exhaust gases, the design of the heat exchanger, and the integration with other energy systems within a plant. The ability to adapt to varying heat loads and different process conditions makes WHRBs highly versatile across a range of industries, from power generation to heavy manufacturing.
One of the most important applications of WHRBs is in power plants, where they recover heat from gas turbines or diesel engines to generate additional electricity. This is particularly beneficial in combined cycle power plants, where the steam produced by a WHRB is used to drive a steam turbine, increasing the overall efficiency of power generation. In industrial facilities, WHRBs are often used to recover heat from high-temperature processes such as metal smelting, cement kilns, and chemical refining, providing steam for internal use and reducing the need for external fuel sources. Marine applications also benefit from WHRBs, as they allow ships to harness heat from engine exhaust to power auxiliary systems, improving fuel efficiency and reducing emissions.
The continuous development of WHRB technology is leading to higher efficiency and better integration with digital control systems. Smart monitoring and automation enable real-time adjustments to optimize heat recovery and minimize energy waste. Predictive maintenance systems use sensors and analytics to detect potential issues before they lead to downtime, improving the reliability and longevity of the equipment. The combination of waste heat recovery with renewable energy technologies, such as solar thermal or biomass, further enhances the sustainability of industrial operations by creating hybrid energy solutions that maximize overall efficiency.
As environmental regulations become stricter and industries seek ways to meet carbon reduction targets, WHRBs are playing a crucial role in helping companies achieve their sustainability goals. Many governments and regulatory bodies offer incentives for adopting energy-efficient technologies, further driving the adoption of WHRB systems. By reducing dependence on fossil fuels and lowering greenhouse gas emissions, WHRBs contribute to a cleaner, more sustainable industrial landscape. Their widespread implementation will continue to shape the future of energy management, ensuring that waste heat is no longer seen as a byproduct but as a valuable resource for improving efficiency and reducing environmental impact.
Waste Heat Recovery Technology
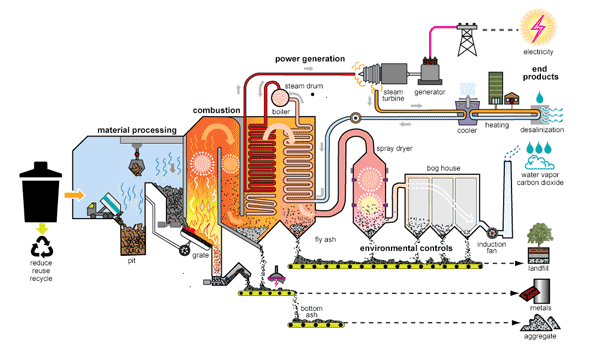
Waste Heat Recovery Technology refers to the process of capturing and reusing heat that would otherwise be lost from industrial processes, power generation, and other energy-intensive operations. This technology improves energy efficiency, reduces fuel consumption, lowers greenhouse gas emissions, and enhances overall sustainability. Waste heat is typically generated from high-temperature exhaust gases, cooling systems, combustion processes, and other thermal energy sources that are often released into the environment without being utilized. By recovering this waste heat, industries can repurpose it for power generation, process heating, or preheating combustion air, significantly improving energy utilization.
The most common waste heat recovery technologies include heat exchangers, waste heat boilers, thermoelectric generators, and organic Rankine cycle (ORC) systems. Heat exchangers, such as shell-and-tube, plate, and regenerative heat exchangers, transfer heat from hot exhaust gases to a secondary fluid, which can then be used in industrial applications. Waste Heat Recovery Boilers (WHRBs) use these hot gases to generate steam, which can drive turbines for electricity generation or provide heat for processes. The Organic Rankine Cycle (ORC) is an advanced technology that converts low-grade waste heat into electricity using an organic working fluid with a lower boiling point than water, making it suitable for industries with moderate-temperature waste heat sources.
Thermoelectric generators (TEGs) utilize the Seebeck effect, where a temperature difference across semiconductor materials generates electricity. While currently limited in efficiency, advancements in thermoelectric materials are improving their potential for small-scale power generation from waste heat. Additionally, absorption refrigeration systems use waste heat to drive cooling processes, improving efficiency in industries that require both heating and cooling. Other emerging technologies, such as phase-change materials and thermal energy storage, further enhance waste heat utilization by storing and redistributing excess heat for later use.
Industries such as power plants, steel manufacturing, cement production, petrochemical processing, and food production benefit significantly from waste heat recovery. In combined heat and power (CHP) plants, waste heat is used to generate electricity and provide heating simultaneously, maximizing energy efficiency. In the transportation sector, waste heat recovery systems in internal combustion engines and marine applications improve fuel efficiency by converting exhaust heat into useful energy. As industries work toward sustainability and regulatory compliance, waste heat recovery technology is becoming an essential tool for reducing energy waste and minimizing environmental impact. Continuous advancements in materials, automation, and energy storage will further enhance the effectiveness and economic viability of waste heat recovery systems, making them a key component of future energy strategies.
Waste Heat Recovery Technology is a critical innovation that enhances energy efficiency by capturing heat that would otherwise be lost from industrial processes, power generation systems, and transportation applications. This waste heat, typically found in exhaust gases from engines, furnaces, and other high-temperature processes, represents a valuable energy source that can be repurposed for electricity generation, process heating, or even cooling applications. The implementation of waste heat recovery systems reduces the need for additional fuel consumption, lowers greenhouse gas emissions, and improves overall sustainability across various industries.
Different technologies are used depending on the temperature and nature of the waste heat source. Heat exchangers, such as shell-and-tube, plate, and recuperative models, transfer heat from exhaust gases to other fluids, enabling preheating or direct utilization in industrial processes. Waste Heat Recovery Boilers (WHRBs) generate steam from high-temperature exhaust gases, which can then be used for process heating or to drive a steam turbine for power generation. The Organic Rankine Cycle (ORC) is particularly effective for converting low-to-medium-temperature waste heat into electricity by using an organic working fluid with a lower boiling point than water, making it suitable for industries such as cement, glass, and geothermal applications.
Advanced technologies such as thermoelectric generators (TEGs) use semiconductor materials to directly convert heat into electricity via the Seebeck effect. Although current efficiency levels are relatively low, research into high-performance thermoelectric materials is expanding their potential for commercial applications, particularly in the automotive and aerospace sectors. Absorption refrigeration systems also play a role in waste heat utilization by using recovered thermal energy to drive cooling cycles, which is beneficial in industries requiring simultaneous heating and cooling, such as food processing and petrochemical plants.
Industries with high energy consumption, such as steel production, cement manufacturing, and petrochemical refining, benefit significantly from waste heat recovery by reducing energy costs and improving process efficiency. Combined heat and power (CHP) systems integrate waste heat recovery with electricity generation, maximizing the efficiency of power plants by using residual heat for industrial applications or district heating. In transportation, waste heat recovery technologies are being explored for internal combustion engines and marine vessels, where exhaust heat can be converted into mechanical or electrical power, improving fuel efficiency.
The continued advancement of waste heat recovery is driven by stricter environmental regulations, economic incentives for energy efficiency, and technological improvements in heat exchangers, thermal storage, and digital monitoring systems. The integration of automation and predictive maintenance further enhances system performance by optimizing heat recovery based on real-time data, reducing downtime, and extending equipment lifespan. As industries transition toward lower carbon emissions and greater energy efficiency, waste heat recovery technology will play an increasingly vital role in sustainable energy strategies, helping to reduce reliance on fossil fuels and minimize environmental impact. The future of waste heat recovery will likely involve hybrid systems that combine multiple energy recovery methods, further improving efficiency and making waste heat a valuable resource rather than a byproduct of industrial processes.
The growing emphasis on sustainability and energy efficiency continues to drive advancements in waste heat recovery technology, making it a crucial component of modern industrial and power generation systems. With rising fuel costs and stricter environmental regulations, industries are increasingly adopting waste heat recovery solutions to optimize energy use, reduce operating expenses, and minimize carbon emissions. The versatility of these systems allows them to be integrated into a wide range of applications, from high-temperature exhaust gas recovery in heavy industries to low-grade waste heat utilization in commercial and residential settings. Emerging innovations are also enhancing the feasibility of capturing and reusing heat from previously untapped sources, further expanding the potential of waste heat recovery technology.
New developments in heat exchanger materials and designs are improving the efficiency of thermal energy transfer, allowing for better heat capture even in lower-temperature applications. High-performance alloys and advanced coatings are enhancing durability and resistance to corrosion, which is essential for maintaining efficiency in harsh industrial environments. In addition, compact heat exchanger designs are enabling more flexible integration into existing industrial setups, reducing the need for extensive modifications. This is particularly beneficial for industries that require retrofitting existing plants with energy recovery systems without disrupting operations.
In power generation, the integration of waste heat recovery with renewable energy sources is becoming an emerging trend. Hybrid systems that combine waste heat recovery with solar thermal, biomass, or geothermal energy are being explored to further improve efficiency and sustainability. These hybrid approaches allow industries to maximize energy utilization and reduce dependency on fossil fuels by leveraging multiple heat sources within a single system. The continued development of Organic Rankine Cycle (ORC) technology is also expanding the possibilities for converting lower-grade heat into usable power, with new working fluids being engineered to optimize performance and efficiency.
Automation and digitalization are playing an increasingly significant role in waste heat recovery, with smart monitoring systems and artificial intelligence-driven controls optimizing heat recovery operations. Predictive maintenance powered by sensors and real-time data analytics is helping industries minimize downtime, extend equipment lifespan, and ensure that heat recovery systems operate at peak efficiency. These advancements are reducing operational risks and making waste heat recovery more attractive for businesses looking to improve their energy management strategies.
The adoption of waste heat recovery technology is also being driven by government incentives and policy support aimed at reducing industrial carbon footprints. Many countries are implementing energy efficiency regulations and offering financial incentives such as tax credits and grants for companies that invest in waste heat recovery solutions. As global industries shift towards decarbonization and cleaner energy practices, waste heat recovery will remain a vital part of achieving net-zero emissions and sustainable industrial growth. The future of this technology will likely involve continued innovation in heat capture efficiency, new applications in emerging industries, and greater integration with digital energy management systems, ensuring that waste heat is no longer seen as an unavoidable loss but as a valuable resource for the circular economy.
Heat exchangers are essential components in waste heat recovery systems, facilitating the transfer of thermal energy between two or more fluids without mixing them. They are used in various industrial, power generation, and HVAC applications to improve energy efficiency by recovering and reusing heat. The choice of heat exchanger type depends on factors such as temperature, pressure, fluid properties, and space constraints.
The most common types of heat exchangers include shell-and-tube heat exchangers, which consist of a bundle of tubes enclosed within a cylindrical shell, making them ideal for high-pressure and high-temperature applications in power plants and chemical processing. Their design allows for easy maintenance and scalability. Plate heat exchangers use thin, corrugated plates to create multiple channels for efficient heat transfer and are widely used in compact systems such as HVAC and food processing. They provide a high surface area for heat exchange and are easy to clean, making them suitable for applications requiring hygiene and frequent maintenance.
Air-cooled heat exchangers, also known as finned-tube heat exchangers, dissipate heat into the surrounding air without the need for water as a cooling medium, making them suitable for industries where water scarcity is a concern. These are commonly found in refineries, gas processing plants, and power stations. Regenerative heat exchangers temporarily store heat in a thermal medium, such as a rotating matrix or packed bed, before transferring it to the incoming fluid, making them highly effective for cyclic operations, such as gas turbines and exhaust heat recovery.
Other specialized heat exchangers include spiral heat exchangers, which feature coiled flow paths for efficient heat transfer in compact spaces, making them suitable for sludge treatment and high-viscosity fluids, and plate-fin heat exchangers, which use multiple layers of fins and plates to maximize heat transfer while maintaining a lightweight and compact structure, often used in aerospace and cryogenic applications. Direct contact heat exchangers allow fluids to come into direct interaction, such as cooling towers, where heat is transferred between water and air through evaporation, commonly used in power plants and industrial cooling processes.
Each heat exchanger type is designed to optimize thermal performance based on specific process requirements. The selection of a heat exchanger depends on operational efficiency, cost-effectiveness, ease of maintenance, and the nature of the heat source. Continuous advancements in materials and design, including the use of corrosion-resistant coatings and enhanced heat transfer surfaces, are improving the durability and effectiveness of modern heat exchangers. As industries seek to optimize energy usage and reduce waste, innovative heat exchanger technologies will continue to play a crucial role in sustainable energy management and heat recovery applications.
Regenerative Heat Exchanger
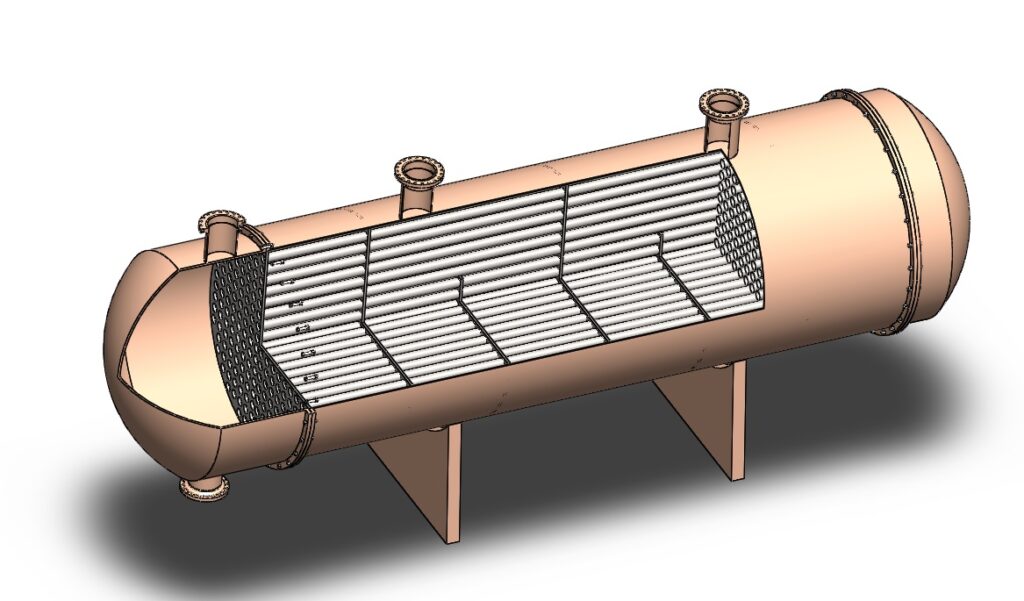
A Regenerative Heat Exchanger is a type of heat exchanger designed to recover heat from exhaust gases or hot fluids and transfer it to incoming fluids. Unlike traditional heat exchangers, which typically rely on two separate fluid streams for heat transfer, regenerative heat exchangers work by temporarily storing thermal energy in a heat-absorbing material, such as a rotating matrix, packed bed, or ceramic stones, before transferring it to the incoming fluid.
The basic principle of a regenerative heat exchanger is that hot gases or fluids are passed over or through a heat-absorbing medium, which captures and retains the thermal energy. This heat is then transferred to the incoming fluid during a subsequent cycle. This process typically occurs in a cyclical or intermittent manner, where the heat-absorbing material alternates between absorbing heat from the hot fluid and transferring it to the cooler fluid.
There are two primary types of regenerative heat exchangers:
- Rotary Regenerative Heat Exchanger: This design uses a rotating wheel or matrix that moves between the hot and cold fluid streams. The wheel or matrix captures heat from the exhaust gases (or hot fluid) and then rotates into the cooler fluid stream to release the stored heat. The process is continuous, with the wheel rotating in such a way that the hot and cold fluids never mix, and heat is transferred efficiently. This type of heat exchanger is commonly used in gas turbines and industrial furnaces, where the temperature difference between the exhaust and intake air is substantial.
- Fixed Matrix Regenerative Heat Exchanger: In this type, a stationary medium (like ceramic stones, metal blocks, or porous materials) is used to absorb heat from the hot fluid. The hot fluid passes over the material, transferring its heat, which is then stored within the medium. Afterward, the medium is exposed to the cooler fluid, releasing the captured heat. This type of heat exchanger is commonly used in smaller-scale applications or processes that involve cyclical heating and cooling, such as in some chemical processes.
Advantages of Regenerative Heat Exchangers include:
- Improved Efficiency: By recovering heat that would otherwise be lost, regenerative heat exchangers can significantly increase the overall thermal efficiency of a system, reducing the need for additional fuel consumption.
- Energy Savings: These heat exchangers are especially valuable in systems where a high temperature difference exists between the exhaust gases and the incoming fluids, allowing for significant energy recovery and cost savings.
- Compact Design: The design of regenerative heat exchangers is typically compact and can be integrated into systems with limited space, making them suitable for industries with space constraints.
- Reduced Environmental Impact: By recovering waste heat and reducing fuel consumption, regenerative heat exchangers help lower greenhouse gas emissions, contributing to sustainability goals.
However, regenerative heat exchangers also have certain limitations, such as the need for periodic maintenance and potential wear on the heat-absorbing medium, especially in systems with high temperatures and corrosive fluids. Despite these challenges, their ability to improve energy efficiency and reduce operational costs makes them a key technology for industries such as power generation, petrochemical processing, and cement manufacturing.
Regenerative heat exchangers are highly efficient devices designed to capture and reuse thermal energy that would otherwise be wasted. They achieve this by utilizing a heat-absorbing medium to store heat temporarily before transferring it to a cooler fluid. The efficiency of this heat recovery is enhanced by the cyclical nature of the process, where the heat-absorbing medium alternates between absorbing heat from the hot fluid and releasing it to the cooler fluid. This design makes regenerative heat exchangers especially suitable for processes where significant temperature gradients exist between the exhaust or waste heat source and the incoming fluid.
In rotary regenerative heat exchangers, the rotating matrix or wheel continuously moves between the hot and cold fluid streams, ensuring constant heat transfer without mixing the two fluids. This allows for the efficient capture and release of heat, making them ideal for use in applications such as gas turbines and industrial furnaces, where the exhaust gases are typically high-temperature and the incoming air or fluid is much cooler. Fixed matrix regenerative heat exchangers, on the other hand, use a stationary medium that collects and stores thermal energy before transferring it to the cooler fluid. Both types of regenerative heat exchangers are particularly effective in systems where the exhaust or waste heat is at a higher temperature and there is a need to recover and reuse this energy to improve overall system efficiency.
One of the primary benefits of regenerative heat exchangers is their ability to improve thermal efficiency, which leads to energy savings and reduced fuel consumption. By capturing waste heat and using it to preheat incoming fluids, these systems lower the demand for additional heating, resulting in a more energy-efficient process. This is particularly important in industries like power generation, cement production, and petrochemical refining, where high temperatures and large volumes of waste heat are common. In addition to energy savings, regenerative heat exchangers also reduce the environmental impact of industrial operations by decreasing the overall fuel use and lowering emissions associated with energy production.
Despite their advantages, regenerative heat exchangers require periodic maintenance, especially the rotating components in rotary systems, which can experience wear over time. The heat-absorbing media used in these systems can also degrade with prolonged exposure to high temperatures, which may require replacement or refurbishment. However, advancements in materials and design are addressing these challenges, improving the longevity and reliability of regenerative heat exchangers.
The continuous development of regenerative heat exchangers, including innovations in heat-absorbing materials, improved sealing technologies, and enhanced control systems, is making them increasingly viable for a wide range of applications. These advancements contribute to reducing operational costs, enhancing energy recovery, and improving sustainability in energy-intensive industries. As industries continue to prioritize energy efficiency and environmental responsibility, regenerative heat exchangers will remain an essential technology in achieving these goals.
Regenerative heat exchangers play a critical role in maximizing the energy efficiency of industrial systems, particularly in sectors where significant amounts of heat are wasted during manufacturing or power generation processes. Their function as energy recovery devices not only reduces operational costs but also supports sustainability initiatives by lowering the need for additional fuel and minimizing environmental impact. By capturing excess heat from exhaust gases or hot fluids and storing it temporarily, regenerative heat exchangers ensure that valuable thermal energy is not lost to the environment but instead reused to optimize the entire system.
In power generation, for example, regenerative heat exchangers can significantly improve the overall efficiency of combined-cycle power plants by recovering heat from exhaust gases of gas turbines and utilizing it to preheat the feedwater entering the steam boiler. This increases the amount of usable energy derived from the same amount of fuel, thereby enhancing the overall thermal efficiency of the plant. This type of energy recovery is especially important in industries with fluctuating energy demands, as it helps maintain system performance while reducing fuel consumption.
The use of regenerative heat exchangers is also becoming more prevalent in industries such as cement manufacturing, steel production, and petrochemical processing, where high-temperature processes produce substantial waste heat. The recovered heat can be repurposed to preheat air for combustion, provide process heat, or be used for other heating requirements, allowing these industries to operate more efficiently while lowering energy costs. In addition, regenerative heat exchangers contribute to better environmental outcomes by reducing the need for additional fuel, which leads to lower carbon emissions and other pollutants.
Advancements in regenerative heat exchanger technology are making these systems even more effective. New materials, such as high-performance ceramics and corrosion-resistant alloys, are being developed to withstand the extreme temperatures and corrosive environments commonly encountered in industrial applications. Moreover, the integration of advanced control systems and automation is allowing for more precise operation and optimized heat recovery cycles, leading to higher levels of energy efficiency and reduced wear on critical components.
Although regenerative heat exchangers come with some maintenance challenges, such as wear and tear on the heat-absorbing medium and moving parts, these systems have proven to be a cost-effective investment in the long term. Regular monitoring and predictive maintenance, aided by sensors and digital technologies, help minimize downtime and extend the lifespan of these systems. With industries facing increasing pressure to reduce their energy consumption and carbon footprints, regenerative heat exchangers are expected to remain a crucial component in achieving more sustainable and efficient industrial processes.
In the future, the continued evolution of regenerative heat exchangers, alongside improvements in heat recovery and integration with renewable energy systems, will enable industries to further optimize their energy consumption and contribute to a cleaner, more sustainable global economy. The growing adoption of these technologies aligns with the push for decarbonization and energy transition, making regenerative heat exchangers a pivotal technology in reducing waste and maximizing the utilization of available thermal energy.
The ongoing development of regenerative heat exchangers is focused on further improving their efficiency, lifespan, and applicability across a wider range of industries. One area of innovation is in the materials used for the heat-absorbing medium. New advanced materials, such as high-temperature resistant ceramics, composites, and durable alloys, are being engineered to withstand extreme thermal cycles and resist corrosion in high-temperature environments. This helps to increase the lifespan of regenerative heat exchangers, making them more cost-effective over time by reducing the frequency of maintenance and replacement of components.
Another area of focus is the design and optimization of the heat transfer process itself. Research is being conducted to enhance the thermal conductivity of the heat-absorbing media, allowing for more efficient heat storage and quicker transfer to the cooler fluid. Some designs incorporate improved surface geometries and enhanced flow dynamics that maximize the contact area between the hot fluid and the heat-absorbing medium, improving heat recovery and reducing losses. This helps to increase the overall efficiency of the heat recovery process, allowing industries to capture more waste heat and utilize it for various applications.
The integration of digital technologies such as sensors, IoT (Internet of Things), and AI-driven control systems is also revolutionizing the performance of regenerative heat exchangers. Real-time data collection from sensors installed throughout the system can provide valuable insights into the heat exchanger’s operating conditions, temperature gradients, and energy flow. AI algorithms can use this data to optimize the heat recovery process, adjusting operating parameters to ensure maximum energy capture while minimizing wear and tear on the components. Predictive maintenance, powered by machine learning algorithms, can forecast potential failures or maintenance needs before they occur, allowing operators to address issues proactively, which helps to reduce downtime and maintenance costs.
In addition, regenerative heat exchangers are increasingly being integrated into hybrid systems that combine waste heat recovery with renewable energy technologies. For example, coupling regenerative heat exchangers with solar thermal or biomass heating systems can create more energy-efficient solutions that use renewable sources to further enhance the performance of waste heat recovery. These hybrid systems can help industrial plants achieve energy independence, reduce reliance on fossil fuels, and meet regulatory emissions reduction targets.
Moreover, the use of regenerative heat exchangers is expanding beyond traditional industrial applications into new sectors. For example, they are being explored for use in HVAC (heating, ventilation, and air conditioning) systems for buildings, where they can help recover heat from exhaust air and use it to preheat incoming fresh air, improving energy efficiency in heating and cooling systems. In the automotive and transportation sectors, regenerative heat exchangers are also being integrated into systems such as waste heat recovery in exhaust systems to improve fuel efficiency and reduce emissions.
As industries continue to prioritize decarbonization and energy efficiency, regenerative heat exchangers will remain a vital component of energy-saving strategies. The continuous evolution of materials, designs, and integration with digital and renewable energy technologies will drive further improvements in their performance. These advancements will enhance the effectiveness of regenerative heat exchangers in reducing energy consumption, lowering emissions, and helping industries achieve their sustainability goals. As a result, regenerative heat exchangers will continue to play a key role in the transition toward a more sustainable and energy-efficient industrial landscape.
A Shell and Tube Heat Exchanger is one of the most widely used types of heat exchangers in industrial applications due to its durability, efficiency, and ability to handle high pressures and temperatures. It consists of a bundle of tubes enclosed within a cylindrical shell, allowing heat to be exchanged between two fluid streams—one flowing inside the tubes and the other around them within the shell. This design enables efficient thermal energy transfer while keeping the fluids separate to prevent contamination.
The working principle of a shell and tube heat exchanger is based on convection and conduction. One fluid, typically the hot fluid, flows through the tubes, while the cooler fluid flows around the outside of the tubes within the shell. Heat is transferred through the tube walls, either heating or cooling the secondary fluid, depending on the application. The flow arrangement can be parallel flow, where both fluids move in the same direction, counterflow, where fluids move in opposite directions for improved efficiency, or crossflow, where one fluid flows perpendicular to the other.
There are several types of shell and tube heat exchangers, including fixed tube sheet exchangers, which have tubes permanently attached to the shell, making them suitable for clean fluids but challenging to clean when fouling occurs, and floating head exchangers, which allow one end of the tube bundle to expand and contract, making maintenance and cleaning easier. Another variation is the U-tube heat exchanger, where tubes are bent into a U-shape, eliminating the need for expansion joints but making cleaning more difficult.
One of the key advantages of shell and tube heat exchangers is their ability to operate under high pressures and temperatures, making them ideal for power plants, chemical processing, and oil refineries. They also offer a large heat transfer surface area, making them efficient for large-scale heat exchange applications. Additionally, they are highly customizable, with variations in tube diameter, length, and shell design to optimize performance for specific processes.
Despite their advantages, shell and tube heat exchangers have some limitations, including a larger footprint compared to compact heat exchangers, potential fouling due to the accumulation of deposits inside the tubes, and the need for periodic maintenance to ensure efficient operation. However, advancements in materials, such as corrosion-resistant alloys and enhanced heat transfer coatings, are improving their longevity and performance. Additionally, modern computational fluid dynamics (CFD) and heat exchanger design software are helping engineers optimize the design for maximum efficiency.
The continued use of shell and tube heat exchangers in industries such as power generation, petrochemical refining, marine applications, and HVAC systems highlights their versatility and reliability. As energy efficiency becomes an increasing priority, ongoing innovations in heat exchanger design and materials will further enhance the performance and sustainability of shell and tube heat exchangers, ensuring their relevance in industrial heat transfer applications.
Shell and tube heat exchangers continue to be a fundamental component of industrial heat transfer systems due to their robustness, versatility, and ability to handle extreme operating conditions. Their efficiency depends on various design parameters, including the number of tube passes, shell configurations, and flow arrangements. Engineers optimize these factors to maximize heat transfer rates while minimizing pressure drops and energy consumption. The number of tube passes is particularly important in determining the overall effectiveness of the exchanger; single-pass designs are simpler and allow for easier maintenance, while multi-pass configurations enhance heat transfer by increasing the residence time of the fluids inside the exchanger.
Material selection also plays a critical role in the performance and longevity of shell and tube heat exchangers. The tubes are typically made of metals with high thermal conductivity, such as copper, stainless steel, or titanium, depending on the fluid properties and the risk of corrosion. For highly corrosive environments, exotic alloys or polymer-coated tubes may be used to extend service life. The shell is often constructed from carbon steel or stainless steel to withstand high pressures and mechanical stresses. Advanced manufacturing techniques, such as seamless tube fabrication and enhanced surface treatments, are continuously improving the durability and heat transfer efficiency of these exchangers.
The efficiency of a shell and tube heat exchanger is also influenced by flow patterns. Counterflow configurations, where hot and cold fluids move in opposite directions, are the most efficient because they maximize the temperature difference between the fluids along the length of the exchanger, leading to higher heat transfer rates. Parallel flow, where both fluids move in the same direction, is less effective because the temperature gradient decreases along the length of the exchanger, reducing the driving force for heat transfer. Crossflow designs, where one fluid moves perpendicular to the other, are often used in applications requiring compact designs and moderate heat transfer efficiency.
Maintenance and fouling prevention are critical for the long-term performance of shell and tube heat exchangers. Over time, deposits from scaling, corrosion, or biological growth can accumulate inside the tubes, reducing heat transfer efficiency and increasing pressure drops. Regular cleaning, either through chemical cleaning methods or mechanical techniques such as high-pressure water jetting, is necessary to maintain optimal performance. Some designs, such as floating head or removable tube bundle configurations, allow for easier cleaning and maintenance by enabling access to the tube bundle without dismantling the entire unit.
Despite being a traditional technology, shell and tube heat exchangers continue to evolve with advancements in design, materials, and computational modeling. Engineers use computational fluid dynamics (CFD) and advanced simulation tools to optimize tube layouts, baffle spacing, and flow distribution, improving overall performance while reducing material costs. Innovations such as twisted tube designs and dimpled tube surfaces enhance turbulence within the fluid streams, further increasing heat transfer efficiency.
The widespread use of shell and tube heat exchangers in industries such as oil and gas refining, power generation, chemical processing, food production, and marine applications highlights their enduring relevance. Their ability to handle phase changes, such as condensing steam or evaporating refrigerants, makes them indispensable in applications where thermal energy needs to be efficiently transferred between fluids of different phases.
As industries focus more on energy efficiency and sustainability, the optimization of heat exchanger designs plays a crucial role in reducing energy waste and improving process efficiency. Integrating these exchangers with waste heat recovery systems allows industries to capture and reuse thermal energy that would otherwise be lost, contributing to lower operational costs and reduced greenhouse gas emissions. With ongoing advancements in materials, manufacturing, and digital monitoring technologies, shell and tube heat exchangers will remain a key component of industrial heat transfer solutions, continuously adapting to meet the demands of modern energy-efficient systems.
The continuous advancement of shell and tube heat exchangers is driven by the need for greater energy efficiency, improved durability, and better adaptability to various industrial applications. One of the key areas of innovation is the enhancement of tube surfaces to improve heat transfer performance. Techniques such as finned tubes, grooved surfaces, and twisted tube designs increase the surface area and create turbulence, reducing the formation of boundary layers and enhancing heat exchange efficiency. These improvements allow for more compact designs while maintaining high thermal performance, which is particularly valuable in space-constrained applications such as marine systems and chemical processing plants.
Another significant development is the integration of advanced materials that offer higher thermal conductivity, better corrosion resistance, and greater mechanical strength. Composite materials, titanium alloys, and corrosion-resistant stainless steel are increasingly being used in heat exchanger construction to withstand aggressive chemical environments, extreme temperatures, and high pressures. In industries such as offshore oil and gas, where exposure to seawater leads to rapid corrosion, the use of these advanced materials significantly extends the lifespan of heat exchangers, reducing maintenance costs and downtime. Additionally, coatings and linings, such as polymer-based or ceramic coatings, are being developed to provide additional protection against corrosion and fouling, further improving long-term reliability.
Computational fluid dynamics (CFD) and artificial intelligence (AI) are playing an increasingly important role in optimizing heat exchanger design. CFD simulations allow engineers to model fluid flow patterns, heat transfer characteristics, and pressure drops to refine tube layouts, baffle placements, and flow distribution strategies. AI-driven predictive maintenance systems use real-time sensor data to detect potential issues such as fouling, leaks, or thermal inefficiencies before they lead to significant performance degradation or failure. By implementing machine learning algorithms, industries can optimize cleaning schedules, adjust operating conditions dynamically, and minimize unplanned downtime, resulting in more efficient and cost-effective operations.
The application of shell and tube heat exchangers is expanding beyond traditional industries into emerging fields such as renewable energy and sustainable heating and cooling solutions. In geothermal power plants, these exchangers play a crucial role in transferring heat from underground reservoirs to working fluids for electricity generation. In solar thermal systems, they are used to capture and distribute heat from solar collectors to storage tanks or process heating applications. Waste heat recovery systems in industrial plants are increasingly relying on shell and tube heat exchangers to capture excess heat from exhaust gases and reuse it for preheating, steam generation, or other thermal processes, improving overall energy efficiency and reducing carbon emissions.
Additionally, hybrid heat exchanger systems that combine shell and tube designs with other heat exchanger technologies, such as plate heat exchangers or air-cooled heat exchangers, are gaining popularity. These hybrid solutions optimize heat recovery and enhance process flexibility by allowing different heat transfer methods to be used based on specific operational needs. For instance, in applications where compactness is essential, plate heat exchangers can be integrated with shell and tube units to achieve a balance between space efficiency and thermal performance.
The push for energy efficiency regulations and environmental sustainability is also driving the development of more efficient shell and tube heat exchangers. Governments and regulatory bodies worldwide are imposing stricter energy efficiency standards, prompting industries to adopt advanced heat exchanger designs that minimize energy losses and reduce environmental impact. Innovations such as variable flow control, optimized baffle arrangements, and automated cleaning systems are being incorporated into modern designs to meet these requirements and enhance the overall performance of heat exchange systems.
Looking ahead, the future of shell and tube heat exchangers will likely involve greater integration with digital technologies, smarter materials with self-cleaning properties, and modular designs that offer greater flexibility in industrial applications. As industries continue to focus on reducing energy consumption and improving sustainability, the role of shell and tube heat exchangers in optimizing thermal processes will remain critical. With continuous improvements in efficiency, durability, and adaptability, these exchangers will continue to be a cornerstone of industrial heat transfer systems, contributing to more sustainable and cost-effective operations across various sectors.
Plate Heat Exchanger
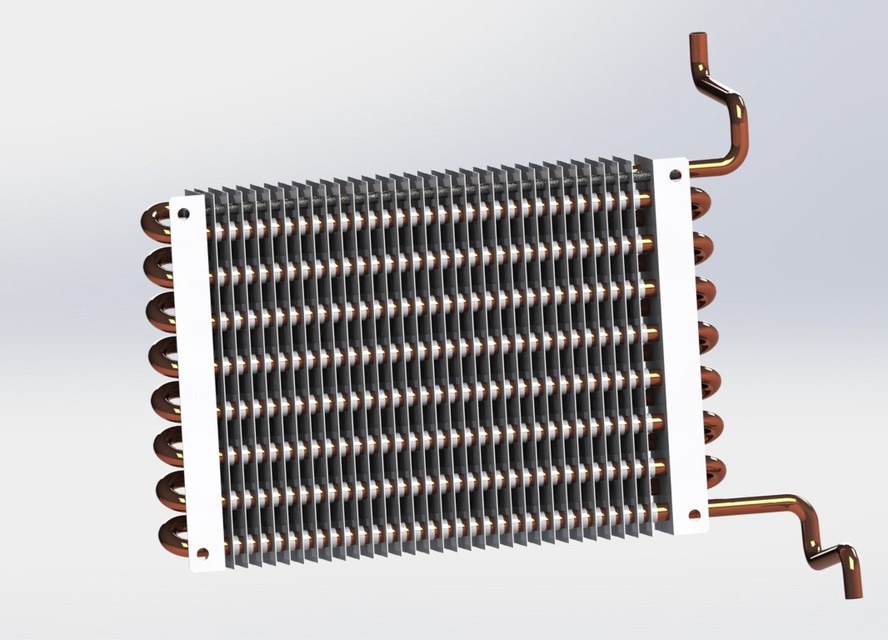
A Plate Heat Exchanger (PHE) is a highly efficient type of heat exchanger that consists of multiple thin, corrugated plates stacked together to facilitate heat transfer between two fluid streams. Unlike shell and tube heat exchangers, which use tubes to separate the fluids, plate heat exchangers rely on large surface areas created by the plates to maximize thermal efficiency. The design allows for compact construction, high heat transfer rates, and easy maintenance, making them popular in industries such as HVAC, food processing, pharmaceuticals, and power generation.
The working principle of a plate heat exchanger is based on thermal conduction and fluid convection. The hot and cold fluids flow through alternating channels between the plates, transferring heat as they pass by each other. The plates are typically arranged in a gasketed, brazed, or welded configuration, depending on the specific application and the need for maintenance access. The corrugated pattern of the plates creates turbulence, enhancing heat transfer efficiency while reducing fouling and deposits.
One of the major advantages of plate heat exchangers is their compact size. Because they offer a high heat transfer coefficient with a smaller footprint compared to shell and tube heat exchangers, they are widely used in applications where space is limited. Additionally, their modular design allows for easy expansion or modification by adding or removing plates to adjust capacity. This flexibility makes them particularly useful in processes where heat load requirements may change over time.
There are several types of plate heat exchangers, including gasketed plate heat exchangers (GPHE), which allow for easy disassembly and cleaning, brazed plate heat exchangers (BPHE), which are sealed permanently by brazing for high-pressure applications, and welded plate heat exchangers, which offer excellent resistance to high temperatures and aggressive fluids. Each type is designed to suit specific industry needs, balancing factors such as ease of maintenance, operational pressure, and resistance to corrosion.
Plate heat exchangers also offer superior energy efficiency due to their high heat transfer surface area and turbulent flow design. They can achieve close temperature approaches between the two fluid streams, allowing for more effective heat recovery and reduced energy consumption. This makes them ideal for waste heat recovery systems, district heating, refrigeration, and industrial cooling processes.
Despite their numerous advantages, plate heat exchangers do have some limitations. They are generally less suitable for applications with very high pressures and temperatures compared to shell and tube heat exchangers. Additionally, gasketed plate heat exchangers require regular maintenance to replace gaskets, which can degrade over time due to exposure to heat and chemicals. For heavily fouling fluids, such as those found in certain chemical and wastewater treatment processes, additional cleaning may be necessary to prevent clogging.
Advancements in plate heat exchanger technology continue to improve their performance and durability. New plate materials, such as titanium and stainless steel, are being used to enhance corrosion resistance and longevity. Computational fluid dynamics (CFD) and advanced manufacturing techniques have also led to more optimized plate geometries that further increase heat transfer efficiency while minimizing pressure drops.
The increasing focus on energy efficiency and sustainability is driving the widespread adoption of plate heat exchangers across various industries. Their ability to recover waste heat, reduce operational costs, and provide flexible thermal management solutions makes them a critical component in modern heat transfer systems. As industries seek to lower their carbon footprint and improve energy utilization, plate heat exchangers will continue to play a crucial role in optimizing thermal processes and enhancing overall system efficiency.
Plate heat exchangers continue to evolve as industries demand more efficient, compact, and durable solutions for heat transfer applications. Their design flexibility and high thermal efficiency make them indispensable in a wide range of sectors, including power generation, chemical processing, refrigeration, and food and beverage production. One of the key advantages of plate heat exchangers is their ability to achieve close temperature approaches, meaning they can transfer heat more effectively between two fluids with minimal temperature differences. This is particularly valuable in energy recovery systems, where maximizing heat utilization can lead to significant cost savings and reduced environmental impact.
The performance of plate heat exchangers is largely influenced by the design of their plates. Corrugated plate patterns are engineered to create turbulence in the fluid streams, which enhances heat transfer while reducing the likelihood of fouling. The choice of plate material is critical, especially for applications involving corrosive fluids or extreme temperatures. Stainless steel is commonly used due to its strength and corrosion resistance, while titanium is preferred in industries such as desalination and marine applications due to its ability to withstand aggressive saltwater environments. Advanced alloys and polymer-coated plates are also being developed to extend service life and improve compatibility with challenging fluid conditions.
Maintenance is a crucial consideration for plate heat exchangers, particularly for gasketed designs that require periodic gasket replacement and cleaning. The ease of disassembly makes them well-suited for industries where frequent cleaning is necessary, such as dairy processing and pharmaceuticals, where hygiene standards are stringent. In contrast, brazed and welded plate heat exchangers eliminate the need for gaskets, making them better suited for high-pressure and high-temperature applications, but at the cost of reduced serviceability. Innovations in self-cleaning designs and anti-fouling coatings are helping to reduce maintenance requirements and extend operational uptime.
Computational fluid dynamics (CFD) and artificial intelligence (AI) are playing an increasing role in optimizing plate heat exchanger performance. CFD simulations allow engineers to model fluid flow, pressure drop, and heat transfer characteristics to fine-tune plate geometries and flow distribution. AI-driven predictive maintenance systems analyze real-time sensor data to detect signs of fouling, leaks, or thermal inefficiencies, enabling proactive maintenance before performance degradation occurs. These advancements not only improve efficiency but also reduce operational costs by minimizing downtime and extending equipment lifespan.
Plate heat exchangers are also finding new applications in sustainable energy systems, where efficient heat recovery is essential for reducing waste and improving energy utilization. In district heating networks, they transfer excess heat from industrial processes or power plants to buildings, reducing reliance on fossil fuels. In renewable energy systems, they are used in geothermal heating, solar thermal power, and biomass energy production to optimize thermal exchange and increase overall system efficiency. The integration of plate heat exchangers into hybrid heating and cooling systems, such as those combining heat pumps with waste heat recovery, further enhances their role in reducing carbon emissions and improving energy sustainability.
As industries continue to prioritize energy efficiency and environmental sustainability, the demand for advanced plate heat exchangers will continue to grow. Research into new materials, enhanced plate designs, and smart monitoring systems is driving improvements in performance, durability, and adaptability. The ongoing evolution of these heat exchangers ensures that they remain a cornerstone of modern thermal management, enabling industries to optimize their processes, reduce energy costs, and minimize their environmental footprint. With continuous advancements in manufacturing techniques and digital integration, plate heat exchangers will play an increasingly vital role in the transition toward a more energy-efficient and sustainable future.
The ongoing advancements in plate heat exchanger technology are making them even more efficient, durable, and adaptable to a broader range of industrial applications. One of the most notable trends is the development of hybrid heat exchanger designs that combine the advantages of different heat exchanger types to optimize performance. For example, hybrid systems may integrate plate heat exchangers with shell and tube or air-cooled exchangers to balance efficiency, pressure resistance, and maintenance ease. These hybrid configurations are particularly valuable in industries such as power generation, chemical processing, and HVAC, where varying operating conditions require flexible thermal management solutions.
In parallel with design improvements, the materials used in plate heat exchangers are also evolving to meet the demands of increasingly harsh operating environments. While stainless steel remains a popular choice for its corrosion resistance and strength, newer materials such as duplex stainless steel, nickel-based alloys, and advanced polymers are gaining traction in applications involving highly aggressive fluids, extreme temperatures, or demanding sanitary requirements. In industries such as food and beverage, pharmaceuticals, and bioprocessing, where hygiene and contamination prevention are critical, non-metallic plate coatings and specialized gasket materials are being introduced to enhance safety and longevity.
Another key innovation is the integration of smart technologies into plate heat exchangers, enabling real-time monitoring and predictive maintenance. Sensors embedded within the exchanger can track parameters such as temperature, pressure, and flow rates, feeding data into AI-driven algorithms that detect anomalies and predict potential failures before they occur. By using machine learning models to analyze operational patterns, companies can optimize cleaning cycles, minimize downtime, and extend the lifespan of their heat exchangers. This level of intelligence is becoming increasingly valuable in industries that require continuous operation, such as power plants, chemical refineries, and large-scale manufacturing facilities.
Energy efficiency remains a primary focus in the development of plate heat exchangers, particularly in applications related to waste heat recovery and renewable energy systems. By capturing and reusing thermal energy that would otherwise be lost, plate heat exchangers contribute to lower energy consumption and reduced carbon emissions. In district heating and cooling systems, they help transfer heat between networks, improving overall system efficiency. In geothermal and solar thermal applications, they facilitate heat exchange between natural heat sources and working fluids, maximizing the potential of renewable energy. The increasing push for carbon neutrality and sustainability is driving industries to integrate these exchangers into innovative energy recovery solutions, making them a crucial component in modern energy infrastructure.
The future of plate heat exchangers is also being shaped by advancements in manufacturing techniques such as 3D printing and advanced laser welding. These technologies enable the creation of more complex plate geometries, reducing weight while increasing heat transfer efficiency. Additive manufacturing methods allow for rapid prototyping and customization of heat exchangers tailored to specific process requirements. Additionally, automation in the production process is improving precision, reducing material waste, and enhancing overall product quality.
As global industries continue to demand more efficient, reliable, and environmentally friendly heat exchange solutions, plate heat exchangers will remain a vital technology. Their compact size, high thermal efficiency, and adaptability to different applications make them indispensable across multiple sectors. With continuous innovation in materials, design, and digital integration, plate heat exchangers are set to play an even greater role in energy efficiency, sustainability, and industrial process optimization. The convergence of smart monitoring, advanced manufacturing, and hybrid heat exchanger systems will ensure that these devices remain at the forefront of thermal management technologies, contributing to a more energy-efficient and sustainable future.
The evolution of plate heat exchangers is not only driven by technological advancements but also by the increasing emphasis on sustainability, regulatory compliance, and operational efficiency. As industries strive to reduce carbon footprints and optimize energy usage, the role of plate heat exchangers in facilitating heat recovery and reducing energy losses is becoming more critical than ever. One area of significant progress is the enhancement of plate geometries to improve heat transfer efficiency while minimizing pressure drops. By refining the design of plate corrugations, manufacturers are able to achieve greater turbulence within the fluid streams, thereby maximizing thermal performance without increasing pumping costs.
The development of compact and high-efficiency plate heat exchangers is also enabling their use in applications that were previously dominated by bulkier heat exchanger types. For instance, in the oil and gas industry, high-temperature and high-pressure plate heat exchangers are being adopted to replace conventional shell and tube systems in certain processes, reducing space requirements and enhancing thermal performance. Similarly, in the maritime sector, plate heat exchangers are being integrated into ship engine cooling systems and waste heat recovery units, contributing to fuel savings and emissions reduction.
In the realm of industrial automation, the implementation of digital twin technology is allowing operators to simulate and optimize plate heat exchanger performance in real time. By creating a virtual replica of the physical system, engineers can analyze different operating scenarios, detect inefficiencies, and predict maintenance needs before issues arise. This predictive approach not only improves reliability but also extends the operational life of the equipment, reducing overall costs.
Another significant trend is the growing use of plate heat exchangers in refrigeration and cooling applications, where they help improve energy efficiency in air conditioning, heat pump, and industrial cooling systems. The ability of plate heat exchangers to operate with low-temperature differentials makes them well-suited for modern, energy-efficient refrigeration cycles. As global regulations push for the use of environmentally friendly refrigerants, plate heat exchanger designs are being optimized to accommodate new low-global-warming-potential (GWP) refrigerants, further supporting sustainability initiatives.
The rapid adoption of renewable energy technologies is also expanding the role of plate heat exchangers in power generation. In concentrated solar power (CSP) plants, they facilitate heat exchange between molten salts and working fluids, improving system efficiency. In hydrogen production and fuel cell systems, plate heat exchangers are used to manage thermal loads and optimize process efficiency, making them an integral part of the transition toward cleaner energy solutions.
Looking ahead, the continued advancement of materials, digital monitoring, and manufacturing processes will further enhance the performance and reliability of plate heat exchangers. With growing global emphasis on energy efficiency and sustainability, these exchangers will play an even more significant role in reducing waste, optimizing industrial processes, and supporting the shift toward greener technologies. The convergence of smart automation, enhanced materials, and hybrid heat exchanger solutions ensures that plate heat exchangers will remain a key technology in the future of heat transfer and thermal management across industries worldwide.
Plate Fin Heat Exchanger
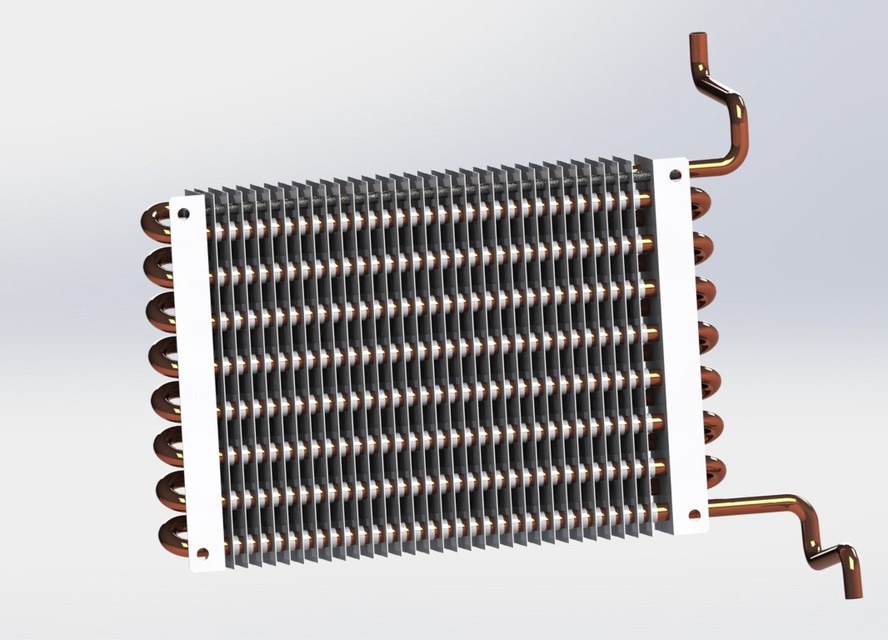
A plate fin heat exchanger (PFHE) is a highly efficient type of heat exchanger that uses thin, corrugated metal fins between parallel plates to facilitate heat transfer between multiple fluid streams. These exchangers are known for their compact design, high surface area-to-volume ratio, and ability to handle multiple fluid flows simultaneously. They are widely used in aerospace, cryogenics, automotive, chemical processing, and power generation due to their ability to provide efficient heat transfer while minimizing weight and size.
The working principle of a plate fin heat exchanger is based on conduction and convection. The plates separate the different fluid streams, while the fins enhance heat transfer by increasing the contact area and promoting turbulence. The exchanger typically consists of alternating layers of hot and cold fluid passages, with fins inserted between them. These fins come in different geometries, such as straight, offset, perforated, and wavy, each designed to optimize heat transfer and pressure drop characteristics. The choice of fin type depends on the application, as some designs prioritize high thermal performance while others minimize pressure loss or fouling.
One of the primary advantages of plate fin heat exchangers is their compact and lightweight design. Compared to traditional shell and tube heat exchangers, PFHEs can achieve the same heat exchange performance with a significantly smaller footprint, making them ideal for applications where space and weight are constraints, such as aircraft engines, satellite cooling, and fuel cell systems. Additionally, the use of aluminum or other lightweight alloys enhances their thermal conductivity while reducing overall system weight, which is particularly beneficial in aerospace and automotive applications.
Another key benefit of plate fin heat exchangers is their ability to handle multiple fluid streams within a single unit. This feature is especially valuable in cryogenic applications such as liquefied natural gas (LNG) production and industrial gas separation, where multiple gases need to be cooled or condensed simultaneously. The ability to integrate different heat transfer processes into a single exchanger improves system efficiency and reduces the need for additional equipment.
Despite their numerous advantages, plate fin heat exchangers also have some limitations. They are highly sensitive to fouling and contamination, as the narrow flow channels can become clogged with debris or deposits, reducing heat transfer efficiency. This makes them less suitable for applications involving heavily contaminated fluids or where regular cleaning is difficult. Additionally, PFHEs are typically more expensive to manufacture due to the complexity of their design and the need for specialized brazing or welding techniques to assemble the fins and plates securely.
Advancements in materials and manufacturing techniques are helping to address some of these challenges. For example, new coatings and surface treatments are being developed to improve corrosion resistance and reduce fouling. Additive manufacturing (3D printing) is also enabling the production of more intricate fin designs that further enhance heat transfer performance while minimizing pressure drop. Additionally, computational fluid dynamics (CFD) is being used to optimize fin geometries and flow patterns, ensuring maximum efficiency and durability.
As industries continue to demand more efficient and compact heat exchangers, plate fin heat exchangers will play an increasingly important role in applications ranging from renewable energy systems to high-performance cooling solutions. Their ability to provide efficient heat transfer in constrained spaces, handle multiple fluid streams, and support extreme temperature applications ensures that they remain a key technology in the future of thermal management. With ongoing advancements in materials, manufacturing, and digital monitoring, PFHEs are poised to become even more efficient and reliable, supporting a wide range of industrial and energy applications.
Plate fin heat exchangers continue to gain popularity due to their superior thermal efficiency, compact design, and ability to handle extreme operating conditions. Their lightweight aluminum construction makes them particularly useful in industries where minimizing weight is crucial, such as aerospace and automotive applications. In aircraft engines, they play a vital role in cooling lubricants, air, and hydraulic fluids, ensuring optimal performance and preventing overheating. Similarly, in automotive systems, they are used in intercoolers, radiators, and exhaust gas recirculation systems to improve fuel efficiency and reduce emissions. Their adaptability to high-temperature and high-pressure environments also makes them essential in power generation and chemical processing, where heat recovery and energy efficiency are critical.
One of the most notable applications of plate fin heat exchangers is in cryogenics, where they are used for gas liquefaction and separation processes. In liquefied natural gas production, they enable efficient heat exchange between multiple gas streams, helping to reduce energy consumption and improve overall system performance. Their ability to handle multiple fluids simultaneously within a single compact unit makes them ideal for industrial gas separation, where different gases such as oxygen, nitrogen, and argon need to be cooled and processed at various temperature levels. The precise thermal control offered by plate fin heat exchangers is a key factor in ensuring the efficiency and reliability of cryogenic processes.
Advancements in manufacturing and design are continuously improving the performance of plate fin heat exchangers. Computational fluid dynamics and finite element analysis are being used to optimize fin geometries, reducing pressure drop while maximizing heat transfer efficiency. The development of new fin configurations, such as louvered and vortex generator fins, is further enhancing heat exchange capabilities by increasing turbulence and improving thermal conductivity. Additionally, advanced brazing and welding techniques are enhancing the structural integrity of plate fin heat exchangers, allowing them to withstand more demanding operating conditions and extending their service life.
Another area of innovation is the integration of smart monitoring technologies, where sensors are embedded within the heat exchanger to provide real-time data on temperature, pressure, and flow rates. This data can be analyzed using artificial intelligence to detect potential issues such as fouling, leaks, or efficiency losses, enabling predictive maintenance and reducing the risk of unexpected failures. By implementing these smart monitoring solutions, industries can optimize the performance of plate fin heat exchangers, reduce downtime, and extend their operational lifespan.
Despite their numerous advantages, plate fin heat exchangers do have some challenges. Their narrow flow channels make them susceptible to fouling and blockages, particularly in applications where fluids contain particulates or contaminants. To address this issue, researchers are developing anti-fouling coatings and self-cleaning designs that reduce the accumulation of deposits and extend maintenance intervals. Additionally, while aluminum is the most commonly used material for these heat exchangers due to its lightweight and high thermal conductivity, alternative materials such as stainless steel and titanium are being explored for applications requiring greater corrosion resistance.
As industries continue to seek energy-efficient and space-saving heat transfer solutions, plate fin heat exchangers will remain a critical component in a wide range of applications. Their ability to handle multiple fluid streams, operate under extreme temperatures and pressures, and deliver superior heat transfer performance ensures their continued relevance in modern thermal management systems. Ongoing advancements in materials, design, and digital technologies will further enhance their efficiency, making them an indispensable technology for industries focused on improving energy utilization, reducing emissions, and optimizing process performance. With their versatility and efficiency, plate fin heat exchangers will continue to play a crucial role in advancing energy, transportation, and industrial systems worldwide.
As plate fin heat exchangers continue to evolve, new developments in materials and manufacturing processes are expanding their applications and improving their efficiency. The use of advanced alloys and composite materials is enhancing their durability and resistance to harsh operating environments, allowing them to function effectively in extreme temperatures and corrosive conditions. In sectors such as chemical processing and power generation, where exposure to aggressive fluids and high thermal loads is common, these innovations are increasing the lifespan and reliability of plate fin heat exchangers. Additionally, the development of ceramic-based heat exchangers is being explored for ultra-high-temperature applications, such as those found in concentrated solar power and advanced aerospace propulsion systems.
The role of additive manufacturing in the production of plate fin heat exchangers is becoming increasingly significant. By utilizing 3D printing technologies, manufacturers can create intricate fin structures that were previously impossible to achieve using traditional methods. These advanced designs improve thermal performance by optimizing surface area and flow distribution while also reducing material waste and production costs. Additive manufacturing also enables rapid prototyping and customization, allowing heat exchangers to be tailored to specific applications with greater precision. This is particularly beneficial in industries such as aerospace and defense, where optimizing heat exchanger geometry can lead to significant performance gains.
Digital twin technology and artificial intelligence are further enhancing the performance and maintenance of plate fin heat exchangers. By creating a virtual replica of an exchanger in operation, engineers can monitor performance metrics in real time and predict maintenance needs before efficiency declines. This predictive approach minimizes downtime, reduces maintenance costs, and extends the operational life of the heat exchanger. AI-driven optimizations also enable dynamic adjustments to operating conditions, ensuring maximum efficiency under varying loads and environmental conditions. As these digital solutions become more widespread, industries will be able to achieve higher levels of automation and energy efficiency, reducing overall operational costs.
Sustainability and energy efficiency remain key drivers in the continued development of plate fin heat exchangers. As governments and industries worldwide seek to reduce carbon emissions, these heat exchangers are playing an essential role in waste heat recovery and renewable energy systems. In industrial processes, they are used to capture and reuse excess heat, improving overall energy utilization and reducing the demand for primary energy sources. In district heating and cooling applications, plate fin heat exchangers enable efficient thermal energy transfer between different buildings and energy sources, contributing to lower energy consumption and enhanced system resilience.
The increasing adoption of hydrogen as a clean energy source is also expanding the use of plate fin heat exchangers in fuel cell technology. In hydrogen production, storage, and distribution systems, these exchangers help regulate temperatures and optimize energy efficiency. Their ability to handle multiple fluid streams and operate in extreme conditions makes them well-suited for managing the thermal requirements of hydrogen-based energy systems. As the hydrogen economy continues to grow, the demand for high-performance plate fin heat exchangers is expected to rise, driving further innovation in this field.
Looking ahead, continued advancements in manufacturing, materials, and digital integration will further enhance the capabilities of plate fin heat exchangers. Their compact design, high thermal efficiency, and adaptability to a wide range of applications ensure their continued importance in industries ranging from aerospace and cryogenics to renewable energy and industrial automation. As energy efficiency and sustainability remain global priorities, plate fin heat exchangers will play a crucial role in optimizing heat transfer processes and supporting the transition to cleaner, more efficient energy systems. With ongoing research and development efforts focused on improving performance and reducing environmental impact, plate fin heat exchangers are set to remain at the forefront of thermal management technologies for years to come.
What is Heat Exchanger
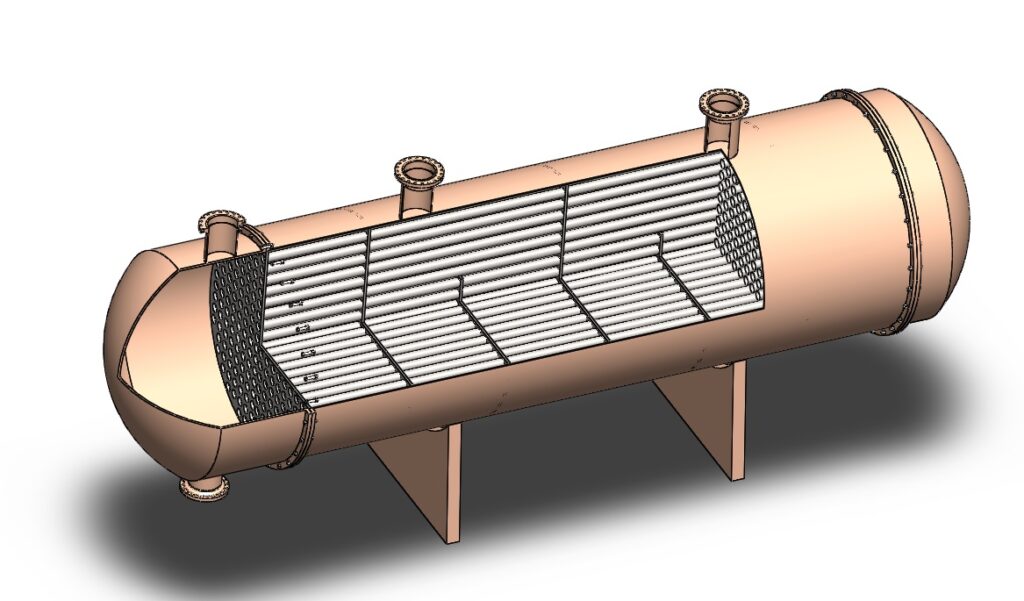
A heat exchanger is a device designed to transfer heat between two or more fluids without allowing them to mix. It is widely used in industrial, commercial, and residential applications to improve energy efficiency, recover waste heat, and regulate temperatures in various processes. Heat exchangers are essential components in power plants, HVAC systems, refrigeration, chemical processing, and many other industries.
The working principle of a heat exchanger is based on the fundamental concept of thermal energy transfer. When two fluids at different temperatures flow through the exchanger, heat naturally moves from the hotter fluid to the cooler one. This process occurs through conduction, convection, or a combination of both, depending on the heat exchanger design. The effectiveness of heat transfer is influenced by factors such as surface area, temperature difference, flow rates, and the properties of the fluids involved.
There are several types of heat exchangers, each suited for specific applications. One of the most common types is the shell and tube heat exchanger, which consists of a series of tubes enclosed within a cylindrical shell. One fluid flows inside the tubes while the other flows around them in the shell, allowing heat exchange through the tube walls. This type is commonly used in power plants, chemical processing, and oil refineries due to its high efficiency and ability to handle large temperature and pressure differences.
Another widely used type is the plate heat exchanger, which consists of thin metal plates stacked together with small gaps between them. The hot and cold fluids flow through alternating channels, and heat is transferred through the plates. Plate heat exchangers provide a high surface area for efficient heat transfer while maintaining a compact design, making them ideal for applications in food processing, HVAC systems, and heat recovery.
For applications requiring highly efficient and compact heat transfer, plate fin heat exchangers are used. These consist of thin metal plates separated by corrugated fins, which enhance heat transfer by increasing the contact surface area. Plate fin heat exchangers are commonly found in aerospace, cryogenics, and automotive industries due to their lightweight structure and ability to handle extreme temperatures.
Heat exchangers also play a crucial role in waste heat recovery, where they capture and reuse thermal energy that would otherwise be lost. For example, in industrial processes, exhaust gases from boilers or turbines can be used to preheat incoming fluids, reducing energy consumption and improving overall efficiency. This principle is widely applied in cogeneration (combined heat and power) systems, where waste heat from electricity generation is repurposed for heating applications.
In HVAC systems, heat exchangers help regulate indoor temperatures by transferring heat between air streams or refrigerants. Air-to-air heat exchangers improve ventilation efficiency by recovering heat from outgoing air, while evaporator and condenser coils in refrigeration systems facilitate cooling by absorbing and releasing heat.
Despite their advantages, heat exchangers require regular maintenance to ensure optimal performance. Over time, fouling—caused by the buildup of scale, corrosion, or biological growth—can reduce heat transfer efficiency and increase energy consumption. Cleaning methods such as chemical cleaning, mechanical brushing, or high-pressure water jetting help maintain performance and prolong the lifespan of the equipment.
Advancements in heat exchanger technology are focused on improving efficiency, reducing size, and enhancing durability. The use of advanced materials, such as corrosion-resistant alloys and ceramic composites, is extending the operating life of heat exchangers in harsh environments. Digital monitoring systems equipped with sensors and predictive analytics are also being integrated to detect performance issues and optimize maintenance schedules.
As industries continue to prioritize energy efficiency and sustainability, heat exchangers will remain a critical component in optimizing thermal processes. Their ability to improve energy utilization, reduce emissions, and enhance system performance ensures their continued importance in modern engineering and industrial applications. With ongoing innovation in design and materials, heat exchangers will play an even greater role in the future of energy management and environmental conservation.
Heat exchangers have evolved significantly over the years to meet the increasing demands for efficiency, compactness, and reliability in various industries. The development of new materials and manufacturing techniques has led to the creation of more efficient and durable heat exchangers, capable of operating in extreme temperatures and pressures. Advances in computational fluid dynamics (CFD) and finite element analysis (FEA) have allowed engineers to optimize heat exchanger designs by simulating heat transfer and fluid flow under different conditions, leading to better performance and lower energy consumption.
One of the key trends in heat exchanger technology is the push for miniaturization without compromising efficiency. Compact heat exchangers, such as microchannel heat exchangers, use small-diameter channels to enhance heat transfer while reducing weight and space requirements. These are widely used in applications such as automotive cooling, electronics thermal management, and air conditioning systems. In power generation, heat exchangers play a vital role in improving energy efficiency by recovering waste heat from exhaust gases in combined-cycle power plants, thereby increasing overall system performance.
The role of heat exchangers in renewable energy systems is also growing. In geothermal energy, heat exchangers extract heat from underground sources to generate electricity or provide heating. In solar thermal power plants, they transfer heat from solar collectors to working fluids, enabling efficient energy conversion. Wind turbines use heat exchangers to cool their electronic components and maintain optimal performance. The transition to hydrogen as a clean fuel has further increased the demand for efficient heat exchangers in hydrogen production, storage, and fuel cell applications, where precise temperature control is essential for safety and efficiency.
Industrial automation and the Internet of Things (IoT) are transforming how heat exchangers are monitored and maintained. Smart sensors embedded within heat exchangers provide real-time data on temperature, pressure, and flow rates, enabling predictive maintenance and reducing downtime. Artificial intelligence and machine learning algorithms analyze this data to detect anomalies, optimize operating conditions, and extend equipment lifespan. By integrating digital monitoring systems, industries can improve reliability, reduce energy waste, and minimize maintenance costs.
Despite their advantages, heat exchangers face challenges such as fouling, corrosion, and thermal stress, which can reduce efficiency and increase operational costs. Researchers are developing new materials and coatings that resist fouling and corrosion, improving durability and reducing maintenance requirements. For example, nanocoatings and self-cleaning surfaces are being explored to prevent the accumulation of deposits that can impair heat transfer. Advanced manufacturing techniques such as 3D printing allow for the production of complex heat exchanger geometries that enhance performance while minimizing material usage.
The environmental impact of heat exchangers is also a growing concern, driving the development of more sustainable and energy-efficient designs. Heat recovery systems are increasingly used to reduce greenhouse gas emissions by capturing and repurposing waste heat in industrial processes. District heating and cooling networks utilize heat exchangers to efficiently distribute thermal energy across buildings and urban areas, reducing overall energy consumption. In refrigeration and air conditioning, the shift toward low-global-warming-potential (GWP) refrigerants is influencing heat exchanger design, requiring compatibility with new working fluids that offer improved environmental performance.
Looking to the future, the continued advancement of heat exchanger technology will play a crucial role in energy efficiency, sustainability, and industrial optimization. As global energy demands rise, the need for more effective heat transfer solutions will drive innovation in materials, design, and digital integration. The convergence of smart automation, advanced manufacturing, and sustainable engineering practices ensures that heat exchangers will remain a fundamental component in a wide range of applications, from power generation and transportation to healthcare and data centers. With ongoing research and technological progress, heat exchangers will continue to evolve, providing more efficient and environmentally friendly solutions for heat management across industries.
As the demand for energy-efficient solutions grows, heat exchangers will continue to play a vital role in optimizing industrial processes, reducing energy consumption, and supporting sustainability initiatives. The integration of heat exchangers in waste heat recovery systems is becoming more widespread across industries, including manufacturing, oil and gas, and chemical processing, where significant amounts of thermal energy are traditionally lost. By capturing and repurposing waste heat, businesses can lower operational costs, reduce reliance on fossil fuels, and decrease greenhouse gas emissions, contributing to more sustainable production methods. This trend is especially important in industries with high energy consumption, such as steel production, cement manufacturing, and glassmaking, where heat exchangers are used to recover energy from exhaust gases, hot water, or steam.
Another emerging application of heat exchangers is in energy storage and advanced thermal management systems. With the rise of renewable energy sources such as solar and wind power, effective energy storage solutions are needed to manage fluctuations in power generation. Heat exchangers are being used in thermal energy storage systems to store excess heat during periods of high energy production and release it when needed, helping to balance supply and demand. In concentrated solar power plants, for example, heat exchangers transfer heat from solar collectors to molten salt storage tanks, allowing for continuous energy generation even after sunset. Similarly, in battery cooling applications, heat exchangers help regulate the temperature of lithium-ion batteries used in electric vehicles and grid-scale energy storage, ensuring safe and efficient operation.
The increasing adoption of heat exchangers in the transportation sector is another key area of development. In automotive applications, advanced heat exchangers improve fuel efficiency and reduce emissions by optimizing the cooling of engines, transmissions, and exhaust gases. Hybrid and electric vehicles rely on high-performance heat exchangers to manage battery temperature, preventing overheating and extending battery life. Aircraft engines and aerospace applications benefit from lightweight, compact heat exchangers that enhance cooling efficiency while minimizing weight, improving overall fuel efficiency and performance. In marine transportation, waste heat recovery systems utilizing heat exchangers are being deployed on ships to improve fuel economy and reduce environmental impact by capturing heat from engine exhaust and using it for onboard power generation or heating systems.
Advancements in heat exchanger materials and coatings are enhancing their durability and efficiency, particularly in harsh environments where corrosion and fouling are major concerns. The development of corrosion-resistant alloys, such as titanium and stainless steel, has expanded the use of heat exchangers in seawater cooling systems, desalination plants, and offshore oil and gas platforms. Nanotechnology-based coatings and self-cleaning surfaces are being introduced to reduce fouling and improve heat transfer efficiency, leading to lower maintenance requirements and longer service life. In high-temperature applications, ceramic heat exchangers are gaining attention for their ability to withstand extreme thermal conditions while maintaining excellent heat transfer properties, making them suitable for applications in gas turbines, nuclear reactors, and advanced manufacturing processes.
The digital transformation of heat exchanger operations is reshaping maintenance and efficiency optimization through the use of real-time monitoring and predictive analytics. Smart heat exchangers equipped with IoT-enabled sensors continuously collect data on fluid temperatures, flow rates, and pressure levels, allowing operators to monitor performance remotely and detect potential issues before they escalate. Artificial intelligence and machine learning algorithms analyze this data to optimize operational parameters, reduce energy waste, and schedule predictive maintenance, minimizing downtime and extending equipment lifespan. These digital innovations are particularly valuable in large-scale industrial facilities, where even small improvements in heat exchanger performance can lead to significant energy savings and cost reductions.
As the global focus on sustainability and energy conservation intensifies, heat exchanger technology will continue to evolve to meet the growing demands for efficiency, reliability, and environmental responsibility. The integration of heat exchangers in carbon capture and utilization (CCU) systems is an area of growing interest, where heat exchangers help manage the thermal processes involved in capturing and converting carbon dioxide emissions into useful products such as synthetic fuels, chemicals, or construction materials. The role of heat exchangers in district heating and cooling networks is also expanding, providing energy-efficient solutions for urban areas by transferring excess heat from industrial sources, data centers, or renewable energy plants to residential and commercial buildings.
The future of heat exchangers will be shaped by ongoing research in advanced materials, manufacturing techniques, and digital intelligence. With continued advancements in additive manufacturing, new heat exchanger designs with optimized geometries and enhanced thermal performance will become increasingly feasible, offering unprecedented efficiency gains. As industries strive to achieve net-zero emissions and improve energy efficiency, heat exchangers will remain an indispensable tool in thermal management, ensuring sustainable and cost-effective energy utilization across diverse applications. Their versatility, adaptability, and continuous innovation will keep them at the forefront of engineering solutions for decades to come.
Waste Heat Recovery
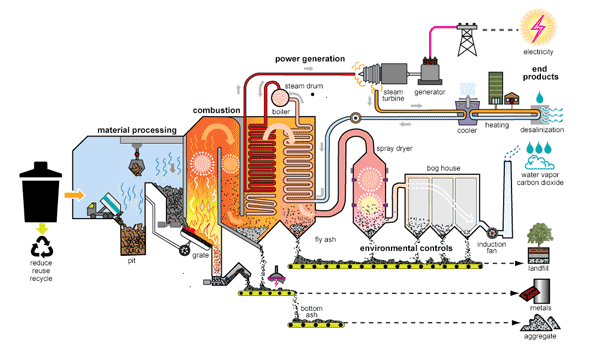
Waste heat recovery is the process of capturing and reusing heat that would otherwise be lost in industrial and commercial operations. This technique improves energy efficiency, reduces fuel consumption, and lowers greenhouse gas emissions. Industries such as power generation, manufacturing, chemical processing, and transportation generate large amounts of waste heat through exhaust gases, cooling systems, and high-temperature industrial processes. By implementing waste heat recovery systems, companies can convert this excess thermal energy into useful work, such as power generation, space heating, or process heating, thereby reducing overall energy demand and improving sustainability.
There are several methods for recovering waste heat, depending on the temperature and quality of the heat source. High-temperature waste heat, typically found in exhaust gases from combustion processes, can be recovered using heat exchangers, economizers, or waste heat boilers. These systems transfer the heat to another medium, such as water or air, to generate steam, hot water, or preheated air for industrial applications. Medium-temperature waste heat can be used in absorption cooling systems, where excess heat is converted into cooling energy for air conditioning or refrigeration. Low-temperature waste heat, often found in cooling water or warm air streams, can be utilized in heat pumps to provide heating for buildings, district heating systems, or preheating process fluids.
One of the most efficient ways to utilize waste heat is through cogeneration, also known as combined heat and power (CHP). In a cogeneration system, waste heat from electricity generation is used to produce steam or hot water for industrial processes or heating applications. This approach significantly improves energy efficiency, as it makes use of both the electrical and thermal outputs from a single fuel source. Trigeneration extends this concept by adding cooling capabilities, using absorption chillers to convert waste heat into cooling energy for air conditioning or refrigeration. These systems are widely used in large industrial plants, hospitals, commercial buildings, and district energy systems.
Waste heat recovery technologies also play a crucial role in improving fuel efficiency and reducing emissions in transportation. In the automotive industry, exhaust gas heat recovery systems are used to capture heat from vehicle engines and convert it into electrical power, reducing fuel consumption and improving overall efficiency. In marine applications, waste heat from ship engines is used to generate steam for auxiliary power or heating, reducing fuel use and operational costs. Similarly, aircraft use waste heat recovery systems to improve cabin heating and reduce fuel requirements.
Advancements in thermoelectric materials and organic Rankine cycle (ORC) technology are expanding the possibilities for waste heat recovery in various industries. Thermoelectric generators (TEGs) convert waste heat directly into electricity using semiconductor materials that generate an electric current when exposed to a temperature gradient. This technology is particularly useful in remote locations where conventional power generation may not be feasible. ORC systems operate similarly to traditional steam power cycles but use an organic working fluid with a lower boiling point, making them suitable for recovering low- and medium-temperature waste heat from industrial processes, geothermal sources, or biomass energy systems.
Despite its many benefits, waste heat recovery faces challenges such as the cost of implementation, space constraints, and efficiency limitations for low-temperature heat sources. However, ongoing research and development efforts are improving the feasibility and economic viability of these systems. Advances in compact heat exchangers, high-efficiency thermal storage, and smart control systems are making waste heat recovery more practical for a wider range of applications. Digital monitoring and predictive maintenance technologies are also helping industries optimize waste heat recovery performance by detecting inefficiencies and preventing system failures.
As industries and governments focus on reducing energy consumption and carbon emissions, waste heat recovery is becoming an essential strategy for improving energy efficiency and sustainability. Many countries are implementing policies and incentives to encourage the adoption of waste heat recovery technologies in industrial and commercial sectors. By integrating waste heat recovery into their operations, businesses can achieve significant cost savings, reduce environmental impact, and enhance overall energy security. With continued technological advancements and increasing awareness of energy conservation, waste heat recovery will play a critical role in the transition toward a more sustainable and energy-efficient future.
Waste heat recovery continues to evolve as industries seek ways to improve energy efficiency and reduce operational costs. The implementation of advanced heat exchangers, regenerative systems, and waste heat boilers has allowed for more effective capture and utilization of thermal energy that would otherwise be lost. In many industrial processes, such as steelmaking, cement production, and chemical manufacturing, significant amounts of heat are generated but not fully utilized. By integrating heat recovery systems, these industries can preheat combustion air, generate steam, or provide direct heating for various applications, leading to substantial energy savings and lower carbon emissions.
The role of waste heat recovery in power generation is expanding, particularly through combined cycle power plants, where gas turbines produce electricity while their exhaust heat generates steam for additional power production. This approach significantly improves overall plant efficiency compared to conventional power generation methods. In distributed energy systems, small-scale cogeneration units are increasingly used in commercial buildings, hospitals, and industrial facilities to provide both electricity and heating, reducing reliance on external power sources and lowering energy costs.
Innovations in organic Rankine cycle technology have made it possible to convert low-grade waste heat into useful energy. These systems use organic working fluids with lower boiling points than water, enabling the conversion of waste heat from sources such as industrial cooling systems, geothermal energy, and biomass combustion into electricity. This makes ORC technology particularly valuable for industries where high-temperature waste heat is not readily available. The integration of thermoelectric generators is also gaining traction, particularly in automotive and small-scale industrial applications, where heat from exhaust systems can be converted directly into electrical power, improving fuel efficiency and reducing emissions.
Waste heat recovery is playing an increasing role in the decarbonization of industries by helping reduce fossil fuel consumption and integrating renewable energy sources. In district heating systems, excess heat from industrial plants, power stations, or data centers is redistributed to residential and commercial buildings, reducing the need for separate heating systems and improving overall energy utilization. Similarly, in greenhouse agriculture, waste heat is used to regulate temperatures, reducing dependence on conventional heating methods and lowering greenhouse gas emissions.
Advances in materials and manufacturing techniques are enhancing the efficiency and durability of waste heat recovery systems. The use of corrosion-resistant alloys, high-performance coatings, and self-cleaning heat exchanger surfaces has improved system longevity and reduced maintenance costs. The adoption of compact and modular heat recovery units has made integration easier, particularly in space-constrained environments such as marine vessels and urban industrial sites. Digitalization is further transforming the field, with smart sensors and artificial intelligence optimizing heat recovery processes, enabling real-time monitoring, and reducing system downtime.
Despite the benefits, some challenges remain, including the need for high initial investment, technical complexity, and limitations in recovering low-temperature waste heat efficiently. However, ongoing research in phase-change materials, advanced heat exchangers, and hybrid energy systems is helping to overcome these barriers. Governments and industries are also introducing policies and incentives to encourage wider adoption, recognizing the role of waste heat recovery in achieving energy efficiency targets and reducing environmental impact.
Looking ahead, waste heat recovery will continue to be a crucial component of industrial energy strategies. As energy prices fluctuate and regulations on carbon emissions become stricter, businesses will increasingly turn to waste heat recovery as a cost-effective and sustainable solution. The integration of emerging technologies such as supercritical CO2 cycles, hydrogen production from waste heat, and AI-driven heat management will further enhance the potential of waste heat recovery systems. With continuous advancements, waste heat recovery is set to play an essential role in improving energy efficiency, lowering emissions, and driving industrial sustainability in the years to come.
The growing importance of waste heat recovery is driving continuous innovation across various industries, leading to more efficient and cost-effective solutions for harnessing and repurposing lost thermal energy. As industries strive to reduce their carbon footprint and comply with stricter environmental regulations, the adoption of waste heat recovery technologies is becoming increasingly essential. The integration of advanced heat exchangers, thermoelectric systems, and energy storage solutions is transforming the way waste heat is utilized, making it possible to recover and convert even low-temperature heat sources that were previously considered uneconomical.
One of the key advancements in waste heat recovery is the increasing use of phase-change materials (PCMs) for thermal energy storage. These materials absorb and release heat at specific temperatures, allowing excess waste heat to be stored and later used when needed. This is particularly useful in applications such as district heating, industrial process optimization, and power generation, where energy demand fluctuates over time. The ability to store and redistribute waste heat enhances overall system efficiency and ensures a more stable energy supply, reducing reliance on fossil fuels and lowering operational costs.
The role of artificial intelligence and machine learning in optimizing waste heat recovery is also expanding. By analyzing real-time data from sensors embedded in heat recovery systems, AI algorithms can predict energy demand, detect inefficiencies, and automatically adjust system parameters to maximize heat utilization. Predictive maintenance enabled by AI helps prevent equipment failures and reduces downtime, improving the overall reliability and lifespan of waste heat recovery installations. These digital innovations are particularly beneficial in large-scale industrial facilities, where even small efficiency improvements can result in significant cost savings and energy reductions.
Another area of development is the use of supercritical CO2 (sCO2) cycles in waste heat recovery power generation. Unlike traditional steam-based systems, sCO2 operates at higher pressures and temperatures, allowing for more efficient heat transfer and power generation from waste heat sources. This technology is gaining interest in industries such as power plants, refineries, and aerospace applications, where high-efficiency energy conversion is critical. The compact size and improved thermal properties of sCO2 systems make them an attractive alternative to conventional steam turbines, paving the way for more advanced waste heat recovery solutions.
In the transportation sector, waste heat recovery continues to improve fuel efficiency and reduce emissions in automotive, marine, and aviation applications. In hybrid and electric vehicles, thermal management systems recover heat from batteries and power electronics to enhance performance and extend battery life. Aircraft are utilizing heat exchangers to recover engine waste heat for cabin heating and power generation, reducing fuel consumption. In the maritime industry, waste heat recovery boilers are becoming standard on large vessels, capturing exhaust heat from engines and using it for steam production, auxiliary power, or onboard heating, leading to significant fuel savings.
The use of waste heat for hydrogen production is another promising avenue, as industries seek cleaner energy alternatives. High-temperature waste heat can be used in thermochemical water-splitting processes or solid oxide electrolyzer cells (SOECs) to produce hydrogen with lower energy input compared to conventional electrolysis. This approach aligns with global efforts to transition to a hydrogen-based economy and decarbonize industrial sectors such as steelmaking, refining, and ammonia production.
Despite these advancements, some challenges remain in fully implementing waste heat recovery systems, particularly in industries with dispersed or intermittent heat sources. Developing cost-effective solutions for recovering low-grade waste heat, integrating heat recovery into existing infrastructure, and ensuring economic feasibility in smaller-scale operations are key areas of focus for future research. Governments and organizations are introducing policies and incentives to encourage investment in waste heat recovery, recognizing its role in improving energy security and reducing greenhouse gas emissions.
Looking forward, waste heat recovery will continue to play a critical role in global energy efficiency strategies. The ongoing convergence of advanced materials, digital optimization, and innovative energy conversion technologies will enable more industries to harness waste heat effectively. As sustainability goals drive the transition toward cleaner energy solutions, waste heat recovery will remain an indispensable tool for reducing energy waste, lowering operational costs, and contributing to a more sustainable and resilient industrial landscape.
EMS Power Machines
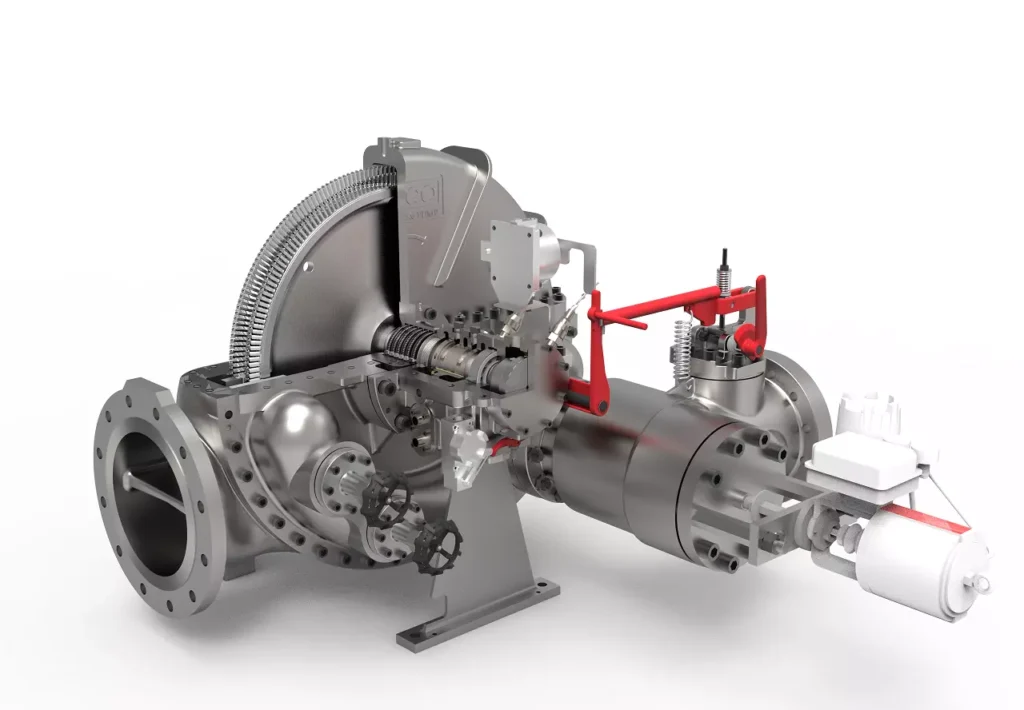
We design, manufacture and assembly Power Machines such as – diesel generators, electric motors, vibration motors, pumps, steam engines and steam turbines
EMS Power Machines is a global power engineering company, one of the five world leaders in the industry in terms of installed equipment. The companies included in the company have been operating in the energy market for more than 60 years.
EMS Power Machines manufactures steam turbines, gas turbines, hydroelectric turbines, generators, and other power equipment for thermal, nuclear, and hydroelectric power plants, as well as for various industries, transport, and marine energy.
EMS Power Machines is a major player in the global power industry, and its equipment is used in power plants all over the world. The company has a strong track record of innovation, and it is constantly developing new and improved technologies.
Here are some examples of Power Machines’ products and services:
- Steam turbines for thermal and nuclear power plants
- Gas turbines for combined cycle power plants and industrial applications
- Hydroelectric turbines for hydroelectric power plants
- Generators for all types of power plants
- Boilers for thermal power plants
- Condensers for thermal power plants
- Reheaters for thermal power plants
- Air preheaters for thermal power plants
- Feedwater pumps for thermal power plants
- Control systems for power plants
- Maintenance and repair services for power plants
EMS Power Machines is committed to providing its customers with high-quality products and services. The company has a strong reputation for reliability and innovation. Power Machines is a leading provider of power equipment and services, and it plays a vital role in the global power industry.
EMS Power Machines, which began in 1961 as a small factory of electric motors, has become a leading global supplier of electronic products for different segments. The search for excellence has resulted in the diversification of the business, adding to the electric motors products which provide from power generation to more efficient means of use.