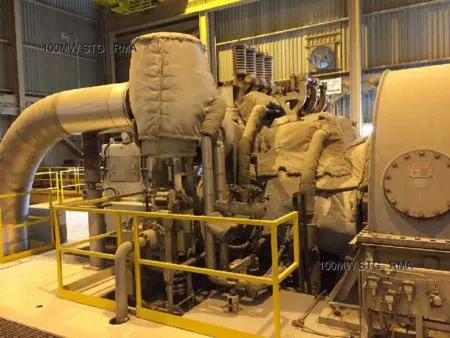
Most Efficient Way to Convert Heat to Electricity: Heat recovery steam generation (HRSG) is an essential process in industrial and power generation applications, aimed at enhancing energy efficiency and reducing waste. The HRSG system is typically used in conjunction with gas turbines or reciprocating engines, where the exhaust gases contain significant amounts of residual heat. Instead of letting this heat escape into the atmosphere, HRSG systems capture and utilize it to produce steam, which can be used for various purposes such as power generation, process heating, or driving turbines.
Waste Heat Recovery Technologies for Maritime Applications
An HRSG system consists of several key components:
- Heat Exchangers: The core of an HRSG, these exchangers transfer heat from the hot exhaust gases to the feedwater, converting it into steam. The main types include:
- Economizers: Preheat the feedwater using lower-temperature exhaust gases, improving overall efficiency.
- Evaporators: Facilitate the phase change of water to steam by transferring heat at the boiling point.
- Superheaters: Increase the temperature of the steam beyond its saturation point, which enhances its efficiency in power generation applications.
- Reheaters (optional): Reheat partially expanded steam in combined-cycle systems for increased efficiency.
- Ducting and Gas Flow System: Directs exhaust gases from the primary engine or turbine through the HRSG unit. The design ensures optimal heat transfer while minimizing pressure drops.
- Steam Drum: Collects steam generated in the evaporator section and separates it from the water. It also serves as a storage vessel to ensure a steady supply of steam during transient conditions.
- Control and Safety Systems: Includes valves, sensors, and controllers to regulate temperature, pressure, and flow rates. Safety mechanisms such as pressure relief valves and emergency shutdown systems are vital for operation.
- Stack: Discharges cooled exhaust gases to the atmosphere after heat recovery, often adhering to stringent environmental regulations to minimize emissions.
Applications of HRSG
Combined Cycle Power Plants
In combined cycle power plants (CCPPs), HRSG systems work alongside gas turbines to enhance overall plant efficiency. The high-temperature exhaust gases from the gas turbine are passed through the HRSG to generate steam, which is then used to drive a steam turbine. This dual-stage process significantly increases the energy extracted from the fuel, often achieving efficiencies above 60%.
Industrial Processes
In industries such as petrochemicals, pulp and paper, and steel manufacturing, HRSG systems are used to recover heat from processes involving high-temperature exhaust gases. The generated steam is used for tasks such as heating, distillation, or chemical reactions.
District Heating
HRSG units can also supply steam or hot water for district heating systems, distributing thermal energy to residential, commercial, and industrial facilities in a centralized manner.
Waste Heat Recovery
In smaller-scale applications, HRSGs are integrated with internal combustion engines or industrial furnaces to recover heat and provide steam for localized energy needs.
Advantages of Heat Recovery Steam Generation
- Improved Energy Efficiency: By utilizing waste heat, HRSG systems reduce the need for additional fuel, leading to significant energy savings.
- Reduced Emissions: Capturing and repurposing waste heat helps decrease greenhouse gas emissions and environmental impact.
- Cost Savings: Lower fuel consumption translates to reduced operational costs, making HRSG systems economically viable in the long term.
- Scalability and Flexibility: HRSG systems can be designed to accommodate various scales and configurations, from single-pressure to multi-pressure systems, depending on the application.
Design Considerations
The design of an HRSG system is influenced by several factors:
- Exhaust Gas Characteristics: Temperature, flow rate, and composition determine the heat recovery potential and material requirements.
- Steam Requirements: Pressure, temperature, and flow rate of the steam depend on the intended application.
- Footprint and Layout: Space constraints and integration with existing systems impact the design.
- Environmental Regulations: Compliance with emission standards and noise control measures must be factored in.
- Operational Flexibility: Modern HRSG systems are designed to handle variable loads and frequent start-stop cycles in response to fluctuating demand.
Challenges in HRSG Systems
While HRSG systems offer significant advantages, they also present challenges:
- Fouling and Corrosion: High-temperature exhaust gases can lead to fouling and corrosion in heat exchanger surfaces, reducing efficiency over time.
- Thermal Stresses: Rapid temperature changes during startup or shutdown can cause thermal fatigue in components.
- Maintenance Requirements: Regular inspection and maintenance are necessary to ensure long-term reliability.
- Capital Costs: The initial investment for HRSG systems can be high, particularly for advanced configurations.
Innovations in HRSG Technology
Recent advancements aim to address these challenges and further enhance the performance of HRSG systems:
- Enhanced Materials: Development of corrosion-resistant alloys and coatings for improved durability.
- Optimized Design Tools: Advanced simulation software for better heat exchanger design and gas flow optimization.
- Integration with Renewable Energy: Combining HRSG systems with solar thermal or biomass energy to create hybrid solutions.
Heat recovery steam generation represents a vital technology for sustainable energy utilization. By transforming waste heat into valuable steam, HRSG systems contribute to improving energy efficiency, reducing environmental impact, and supporting the transition to cleaner energy systems. As industries and power plants increasingly focus on energy conservation, the role of HRSG technology will continue to grow in importance.
Heat Exchangers: An Overview
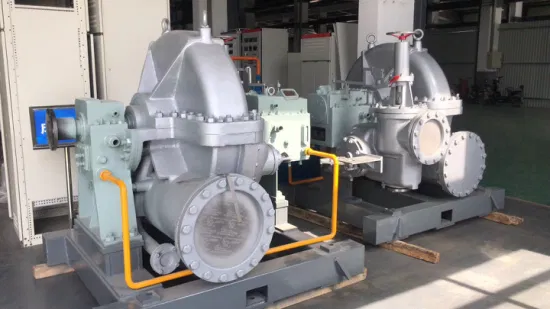
Heat exchangers are essential components in a wide range of industrial, commercial, and domestic applications. Their primary function is to transfer heat between two or more fluids—such as liquids, gases, or a combination of both—without allowing the fluids to mix. Heat exchangers play a critical role in improving energy efficiency, optimizing thermal management, and enabling various processes in industries such as power generation, chemical processing, HVAC, and automotive manufacturing.
Principles of Operation
The basic principle of a heat exchanger involves the transfer of heat from a hotter fluid to a cooler fluid, separated by a solid barrier or facilitated through direct contact. The heat transfer process occurs via conduction, convection, or a combination of these mechanisms. The performance of a heat exchanger depends on several factors, including the heat transfer area, fluid flow rates, temperature gradients, and material properties.
Types of Heat Exchangers
Heat exchangers are classified based on their design, flow arrangement, and application:
1. Based on Flow Arrangement:
- Parallel Flow Heat Exchangers: In this configuration, the hot and cold fluids flow in the same direction. While simple in design, the temperature difference decreases along the flow path, limiting heat transfer efficiency.
- Counterflow Heat Exchangers: Here, the fluids flow in opposite directions. This arrangement maximizes the temperature gradient and is more efficient than parallel flow designs.
- Crossflow Heat Exchangers: In this type, the fluids flow perpendicular to each other. They are commonly used in applications such as air conditioning and refrigeration.
2. Based on Construction:
- Shell-and-Tube Heat Exchangers: These consist of a bundle of tubes housed within a shell. One fluid flows through the tubes, while the other flows around them in the shell. They are widely used in industries due to their robustness and versatility.
- Plate Heat Exchangers: These use a series of thin, corrugated plates to facilitate heat transfer. The fluids flow in alternating channels created by the plates. Compact and efficient, plate heat exchangers are ideal for applications with space constraints.
- Air-Cooled Heat Exchangers: In these systems, air is used as the cooling medium. Fans force air across finned tubes or surfaces, dissipating heat into the atmosphere. They are common in situations where water is scarce.
- Spiral Heat Exchangers: These consist of two concentric spiral channels that allow fluids to flow in a compact and efficient manner. They are effective for handling viscous fluids or fluids with suspended particles.
3. Based on Heat Transfer Mechanism:
- Direct Contact Heat Exchangers: The fluids come into direct contact and exchange heat, often seen in processes like cooling towers or gas scrubbers.
- Indirect Contact Heat Exchangers: A solid barrier separates the fluids, preventing mixing while allowing heat transfer. This is the most common type in industrial settings.
Applications of Heat Exchangers
Heat exchangers are integral to numerous systems, including:
- Power Generation: In thermal and nuclear power plants, heat exchangers play a critical role in converting heat into mechanical energy and, ultimately, electrical energy. Condensers and economizers are examples of heat exchangers used in these plants.
- Chemical and Petrochemical Industry: Processes like distillation, cracking, and refining require precise thermal management, which is achieved using specialized heat exchangers.
- HVAC Systems: Heat exchangers are vital for heating, ventilation, and air conditioning systems, ensuring efficient temperature control in residential, commercial, and industrial buildings.
- Automotive Industry: Radiators and intercoolers are examples of heat exchangers used to maintain optimal engine temperatures and improve vehicle performance.
- Food and Beverage Processing: Pasteurization, sterilization, and other processes rely on heat exchangers for controlled heating and cooling.
- Cryogenic Applications: Heat exchangers are used in processes involving extremely low temperatures, such as liquefied natural gas (LNG) production.
Advantages of Heat Exchangers
- Energy Efficiency: By recovering and reusing waste heat, heat exchangers improve overall energy efficiency, reducing fuel consumption and operational costs.
- Customizability: Heat exchangers can be tailored to meet specific thermal and spatial requirements.
- Durability: Made from materials like stainless steel, copper, or titanium, heat exchangers are designed to withstand high pressures, temperatures, and corrosive environments.
- Environmental Benefits: Improved heat recovery and thermal management contribute to lower greenhouse gas emissions.
Challenges in Heat Exchanger Design and Operation
- Fouling and Scaling: Deposits from the fluids can accumulate on heat exchanger surfaces, reducing heat transfer efficiency and increasing maintenance costs.
- Corrosion: Prolonged exposure to corrosive fluids or environments can damage heat exchangers, necessitating the use of resistant materials or coatings.
- Thermal Stresses: Sudden temperature changes can cause material fatigue or failure, requiring careful design and operation.
- Pressure Drops: Improper flow design can lead to significant pressure losses, reducing overall system efficiency.
Advances in Heat Exchanger Technology
To address these challenges and improve performance, several innovations have been introduced:
- Additive Manufacturing: 3D printing enables the production of complex geometries, enhancing heat transfer efficiency and reducing material usage.
- Nanotechnology: Nano-coatings and nanofluids improve thermal conductivity and resistance to fouling and corrosion.
- Compact Heat Exchangers: Advances in design and materials have led to smaller, lighter heat exchangers with higher efficiency, ideal for space-constrained applications.
- Smart Monitoring Systems: Sensors and IoT-enabled systems provide real-time data on performance, enabling predictive maintenance and reducing downtime.
Conclusion
Heat exchangers are indispensable in modern technology, offering solutions to a wide array of thermal management challenges. By transferring heat efficiently, they enhance energy conservation, reduce costs, and support sustainable practices across industries. As technology advances, the continued development of heat exchangers will play a pivotal role in meeting the growing demands of energy efficiency and environmental stewardship.
Economizers: Enhancing Energy Efficiency in Heat Recovery Systems
Economizers are specialized heat exchangers designed to recover residual heat from exhaust gases or fluids and use it to preheat another fluid, typically water or air. They are widely employed in industrial and power plant settings to improve energy efficiency, reduce fuel consumption, and lower operational costs. By recovering waste heat from exhaust streams, economizers maximize the utilization of available energy and contribute to sustainable practices.
Principles of Operation
The primary function of an economizer is to transfer heat from a hot exhaust stream to a cooler fluid. In most cases, economizers are installed in the exhaust path of boilers, furnaces, or gas turbines. The recovered heat is used to preheat feedwater entering the boiler or air for combustion, thereby reducing the energy required to achieve desired temperatures.
Heat transfer in economizers typically occurs through conduction and convection. The design ensures a high heat transfer surface area, allowing efficient energy recovery while minimizing pressure losses.
Types of Economizers
Economizers are classified based on their design, the medium they heat, and their specific applications:
1. Boiler Economizers
- Found in steam power plants and industrial boilers, these economizers recover heat from flue gases to preheat boiler feedwater.
- This reduces the energy required to convert water into steam, enhancing boiler efficiency.
2. Air-Side Economizers
- These economizers transfer heat to incoming combustion air, making the combustion process more efficient.
- They are common in furnaces and gas turbines, where preheated air reduces the fuel required for combustion.
3. Condensing Economizers
- These economizers recover both sensible and latent heat from flue gases by cooling them below their dew point.
- The condensation of water vapor in the exhaust stream releases additional heat, which can be used for feedwater or space heating.
4. Non-Condensing Economizers
- These economizers recover only the sensible heat, operating above the dew point of the flue gases.
- They are simpler in design and are used in applications where condensation is undesirable.
5. Heat Recovery Steam Generator (HRSG) Economizers
- Integrated into HRSG systems in combined-cycle power plants, these economizers recover heat from gas turbine exhaust to preheat feedwater for steam generation.
Applications of Economizers
Economizers are utilized across a range of industries and processes:
- Power Generation: In thermal and combined-cycle power plants, economizers are integral to improving the efficiency of boilers and HRSGs by preheating feedwater with exhaust heat.
- Industrial Boilers: Economizers are used to enhance the efficiency of industrial boilers in sectors like food processing, chemical manufacturing, and pulp and paper production.
- HVAC Systems: In heating, ventilation, and air conditioning (HVAC) systems, economizers preheat air or water, reducing the energy required for space heating.
- District Heating Systems: Economizers recover heat from power plants or industrial processes for distribution in district heating networks.
- Marine Applications: On ships, economizers recover heat from engine exhaust gases to generate steam or heat water, improving the overall energy efficiency of marine engines.
Benefits of Economizers
- Energy Savings: By recovering waste heat, economizers reduce the amount of fuel required for heating or steam generation.
- Lower Operating Costs: Reduced fuel consumption translates to significant cost savings over time.
- Environmental Benefits: Improved energy efficiency leads to lower greenhouse gas emissions and reduced environmental impact.
- Enhanced Equipment Lifespan: Preheating feedwater or air reduces thermal stresses on boilers and other equipment, extending their operational life.
- Increased System Efficiency: Economizers optimize the thermal performance of industrial and power generation systems.
Design Considerations
The effectiveness of an economizer depends on careful design and material selection:
- Heat Transfer Surface: Finned tubes or corrugated plates are often used to increase the heat transfer area and improve efficiency.
- Material Selection: Materials must withstand high temperatures, corrosive gases, and thermal cycling. Common choices include stainless steel and alloyed steels.
- Flow Arrangement: Counterflow designs are preferred for maximizing the temperature gradient and heat transfer efficiency.
- Dew Point Considerations: For condensing economizers, materials and coatings must resist corrosion caused by acidic condensates.
- Pressure Drop: Excessive pressure drops can reduce system performance, so the economizer must balance heat transfer with flow resistance.
Challenges in Economizer Operation
- Fouling and Scaling: Particulates and deposits from exhaust gases can accumulate on heat transfer surfaces, reducing efficiency and requiring regular cleaning.
- Corrosion: Condensing economizers are particularly susceptible to corrosion from acidic condensates, necessitating corrosion-resistant materials or coatings.
- Space Constraints: Economizers need to be compact enough to fit into existing systems without compromising performance.
- Maintenance Requirements: Regular inspection and maintenance are crucial to ensure long-term reliability and performance.
Innovations in Economizer Technology
Recent advancements have improved the performance, reliability, and versatility of economizers:
- Advanced Materials: Development of high-temperature alloys and anti-corrosion coatings extends the lifespan of economizers in harsh environments.
- Compact Designs: Modular and space-saving designs allow economizers to be integrated into systems with limited room.
- Smart Monitoring Systems: IoT-enabled sensors provide real-time performance data, enabling predictive maintenance and optimizing operation.
- Hybrid Systems: Integration with renewable energy systems, such as solar thermal or biomass, enhances overall energy efficiency.
Conclusion
Economizers are a critical component of energy recovery systems, playing a vital role in improving energy efficiency and reducing operational costs in various industries. By capturing waste heat and repurposing it for preheating, they contribute to sustainable energy practices and environmental conservation. With ongoing advancements in materials, design, and monitoring technologies, economizers are set to remain a cornerstone of energy-efficient systems for years to come.
Economizers are vital components in energy recovery systems, designed to enhance efficiency by capturing and utilizing residual heat from exhaust gases or fluids. Their primary purpose is to preheat a secondary fluid, such as feedwater or air, thereby reducing the energy required for subsequent heating processes. By recovering waste heat that would otherwise be lost to the environment, economizers contribute significantly to cost savings, energy conservation, and environmental sustainability. They are widely used in various industries, including power generation, manufacturing, HVAC systems, and marine applications, where thermal efficiency is a critical consideration.
The basic operation of an economizer revolves around heat exchange. Exhaust gases or fluids, often at high temperatures, pass through the economizer, transferring their heat to the incoming feedwater or air. This preheating process reduces the temperature differential required for heating the fluid in boilers or furnaces, thereby lowering the amount of fuel consumed. In power plants, economizers are typically installed in the flue gas path of boilers or heat recovery steam generators (HRSGs) to maximize the utilization of energy from combustion processes. Similarly, in industrial boilers, economizers preheat feedwater before it enters the boiler drum, enhancing overall efficiency.
One of the key advantages of economizers is their ability to reduce fuel consumption, which directly translates to cost savings. By recovering and reusing heat, less fuel is required to achieve the desired temperature, leading to lower operational expenses over time. Additionally, the use of economizers results in lower emissions of greenhouse gases and other pollutants, as the reduced fuel consumption leads to decreased combustion byproducts. This environmental benefit is especially significant in industries where stringent regulations on emissions are in place. Furthermore, economizers help extend the lifespan of equipment such as boilers and furnaces by minimizing thermal stresses caused by large temperature differences.
Despite their numerous advantages, the design and operation of economizers come with challenges that must be carefully managed. One common issue is fouling, which occurs when particulates or residues from exhaust gases accumulate on heat transfer surfaces, reducing the efficiency of heat exchange. Regular cleaning and maintenance are necessary to address this issue, although advancements in material coatings and self-cleaning designs have helped mitigate its impact. Corrosion is another concern, particularly in condensing economizers where exhaust gases are cooled below their dew point, leading to the formation of acidic condensates. The use of corrosion-resistant materials such as stainless steel or specially coated surfaces can reduce the risk of damage and prolong the economizer’s operational life.
The integration of economizers into existing systems requires careful consideration of several factors. The materials used must be able to withstand high temperatures and resist corrosion over extended periods. The design must also account for the pressure drop caused by the economizer, as excessive resistance can compromise overall system performance. Flow arrangements are optimized to maximize heat transfer efficiency, with counterflow designs often preferred for their ability to maintain a high temperature gradient. In some cases, economizers are custom-designed to meet specific operational and spatial constraints, ensuring they fit seamlessly into the overall system.
Advancements in economizer technology have further enhanced their performance and versatility. Compact designs, for instance, have allowed economizers to be integrated into systems with limited space without sacrificing efficiency. The adoption of advanced materials and coatings has improved resistance to fouling and corrosion, reducing maintenance requirements and extending service life. Smart monitoring systems, enabled by IoT technology, provide real-time data on economizer performance, enabling predictive maintenance and optimal operation. In hybrid energy systems, economizers have been successfully integrated with renewable energy sources, such as solar thermal or biomass systems, to create more sustainable and energy-efficient solutions.
In summary, economizers represent a critical innovation in the field of energy recovery, offering substantial benefits in terms of efficiency, cost savings, and environmental impact. By recovering waste heat and repurposing it for useful applications, they enable industries to maximize the use of available energy resources while reducing their carbon footprint. As technology continues to advance, economizers will play an increasingly important role in the drive toward energy-efficient and sustainable industrial practices. Their adaptability to diverse applications and ongoing improvements in design and materials ensure that they remain a cornerstone of modern energy management systems.
Evaporators
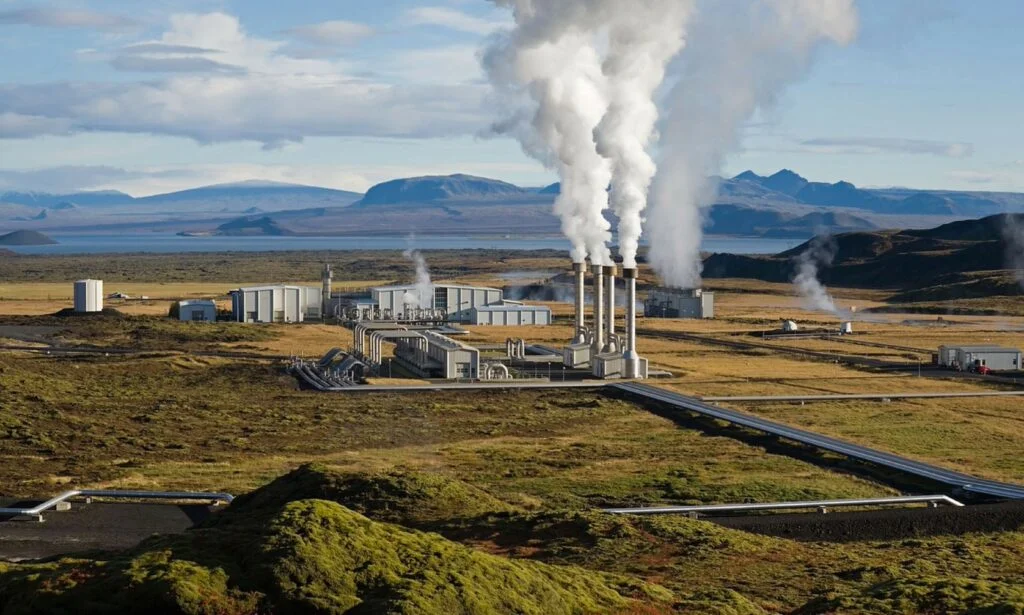
Evaporators are essential components in many industrial, commercial, and domestic applications, where their primary function is to separate a solvent, typically water, from a solution through the process of evaporation. By heating a liquid to its boiling point, evaporators cause the solvent to vaporize, leaving behind the concentrated solute or extract. This process is crucial in industries such as food and beverage, pharmaceuticals, chemicals, and desalination, where the efficient removal of water or other solvents is necessary to achieve product specifications or process requirements. The versatility of evaporators has made them indispensable in applications ranging from producing concentrated juices and milk powders to treating wastewater and generating fresh water from seawater.
The fundamental principle of an evaporator involves the transfer of heat to the liquid, raising its temperature to the boiling point. The heat source can vary, including steam, electricity, or combustion, depending on the specific design and operational needs. As the liquid reaches its boiling point, it transitions into vapor, which is then separated from the remaining liquid. In most evaporator designs, the vapor is directed to a condenser, where it is cooled and collected as a separate stream, often for reuse or further processing. The efficiency of an evaporator depends on several factors, including the heat transfer surface area, the temperature gradient, the properties of the liquid being processed, and the design of the evaporation chamber.
One of the most common types of evaporators is the single-effect evaporator, which consists of a single chamber where the liquid is heated and evaporated. While simple in design, single-effect evaporators are less energy-efficient because they utilize the heat only once before discharging it. To address this limitation, multiple-effect evaporators are often used in industrial applications. In a multiple-effect evaporator, the vapor generated in the first effect is used as the heating medium for the subsequent effects, allowing the heat to be reused multiple times. This cascading use of heat significantly improves energy efficiency, reducing operational costs and minimizing environmental impact. Depending on the number of effects, these systems can achieve substantial energy savings compared to single-effect designs.
In certain applications, falling film evaporators are employed to enhance efficiency and handle heat-sensitive materials. These evaporators use a thin film of liquid flowing over a heated surface, which promotes rapid evaporation with minimal thermal degradation of the product. Falling film designs are particularly useful in industries like dairy, where maintaining the quality of heat-sensitive components such as proteins and vitamins is critical. Similarly, rising film evaporators rely on natural circulation caused by vapor bubbles to lift the liquid upward along the heated surface, creating a high heat transfer rate and efficient evaporation. Other specialized designs, such as forced circulation evaporators and plate evaporators, cater to unique processing needs, including handling viscous liquids or ensuring compact system configurations.
Evaporators are also central to thermal desalination processes, where they are used to separate salt and other impurities from seawater to produce fresh water. In methods like multi-effect distillation (MED) and multi-stage flash distillation (MSF), evaporators play a pivotal role in converting saline water into potable water. These systems are widely implemented in arid regions and on ships, where access to freshwater resources is limited. The ability of evaporators to operate in closed-loop systems, recycling both the heat and the water, makes them a sustainable solution for freshwater production and industrial water reuse.
Despite their advantages, evaporators face several challenges that must be addressed to ensure reliable and efficient operation. Fouling and scaling are common issues, as dissolved salts, organic materials, and other impurities can accumulate on heat transfer surfaces, reducing efficiency and necessitating frequent cleaning. Selecting appropriate materials for construction, such as stainless steel or titanium, can mitigate corrosion and scaling issues, especially in harsh environments. Additionally, the design of evaporators must account for energy efficiency and minimize heat losses, as energy consumption is a significant cost factor in evaporation processes. Incorporating advanced insulation, optimizing heat exchangers, and employing heat recovery systems are strategies that enhance the performance and cost-effectiveness of evaporators.
The integration of advanced technologies has further improved the capabilities of evaporators. For instance, the use of mechanical vapor recompression (MVR) allows the vapor generated during evaporation to be compressed and reused as a heat source, drastically reducing energy consumption. Similarly, the application of smart monitoring systems equipped with sensors and IoT connectivity provides real-time data on evaporator performance, enabling predictive maintenance and process optimization. These innovations not only enhance the operational efficiency of evaporators but also reduce their environmental impact, aligning with the growing emphasis on sustainable industrial practices.
In summary, evaporators are vital in processes that require the concentration of solutions, the removal of solvents, or the generation of purified products. Their diverse applications, ranging from food production and pharmaceuticals to desalination and wastewater treatment, underscore their importance in modern industry. While challenges such as fouling, scaling, and energy consumption persist, advancements in design, materials, and technology continue to drive improvements in their efficiency and reliability. As industries prioritize sustainability and resource efficiency, evaporators will remain central to achieving these goals, ensuring their relevance in both traditional and emerging applications.
Superheaters
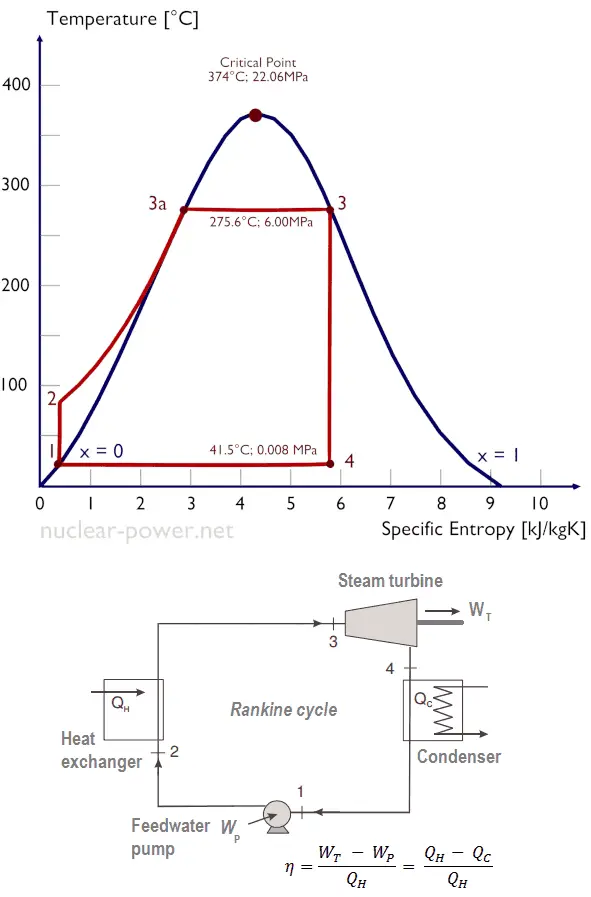
Superheaters are critical components in steam generation systems, designed to increase the thermal energy of steam by raising its temperature above its saturation point. Unlike saturated steam, which exists in equilibrium with water at its boiling point, superheated steam is completely free of moisture and possesses higher enthalpy, making it highly efficient for various industrial and power generation applications. The primary purpose of a superheater is to enhance the efficiency of steam turbines, engines, or industrial processes by providing dry, high-temperature steam, which minimizes energy losses and improves performance.
The operation of a superheater involves transferring heat to the saturated steam produced in the boiler, raising its temperature to a superheated state. This process occurs in the superheater tubes, which are exposed to high-temperature flue gases from the combustion process. The heat transfer mechanism is primarily convection and radiation, with the design and placement of the superheater optimized to ensure uniform heating and prevent overheating of the tubes. The degree of superheating required depends on the specific application, with higher temperatures often needed for power generation and lower temperatures for industrial processes.
Superheaters are typically categorized based on their design and placement within the boiler system. Convective superheaters rely on the flow of hot gases over the tube surfaces, where heat is transferred primarily through convection. These are usually located away from the combustion zone to prevent exposure to extreme temperatures. Radiant superheaters, on the other hand, are positioned closer to the combustion chamber and absorb heat through radiation, allowing for rapid and effective temperature increases. Many boiler systems use a combination of radiant and convective superheaters to achieve precise temperature control and maximize efficiency. Additionally, reheat superheaters, used in advanced power plants, reheat the exhaust steam from turbines to maintain high thermal efficiency during multiple expansion stages.
The primary advantage of using a superheater is the improved efficiency and performance of steam-driven systems. In power plants, superheated steam enables turbines to operate at higher thermal efficiency by reducing condensation losses and mechanical wear caused by wet steam. This results in greater energy output for the same amount of fuel consumed. Similarly, in industrial processes such as chemical manufacturing, petroleum refining, and food processing, the use of superheated steam ensures consistent and high-quality results by eliminating the presence of moisture that could interfere with the process.
Superheaters also contribute to the longevity and reliability of equipment. By providing dry steam, they prevent the erosion and corrosion of turbine blades, piping, and other components caused by the impact of water droplets. This reduces maintenance requirements and extends the service life of critical machinery. Additionally, superheated steam can achieve higher velocities and pressure differentials, making it ideal for applications requiring high kinetic energy or heat transfer rates, such as sterilization, drying, and power transmission.
However, the design and operation of superheaters present several challenges that must be carefully managed. One significant concern is overheating, which can lead to thermal stresses, material fatigue, and eventual failure of the superheater tubes. To prevent this, superheaters are constructed using high-temperature alloys and are equipped with temperature control mechanisms, such as attemperators or desuperheaters, to regulate steam temperature. Fouling and slagging, caused by deposits of ash or soot on the heat transfer surfaces, can also impair efficiency and require regular cleaning. Advanced cleaning systems, such as soot blowers, are often incorporated into boiler designs to address this issue.
Energy efficiency is another critical consideration in the operation of superheaters. Since the process of superheating requires additional fuel input, optimizing heat transfer and minimizing heat losses are essential to ensure cost-effective operation. Insulation of superheater tubes, efficient combustion control, and waste heat recovery systems are some strategies used to enhance energy efficiency. The placement of the superheater within the boiler system is also carefully designed to maximize exposure to the hottest flue gases without compromising tube integrity.
Technological advancements have significantly improved the performance and reliability of superheaters. The development of high-performance alloys and coatings has increased resistance to thermal stress, corrosion, and fouling, enabling superheaters to operate at higher temperatures and pressures. Smart monitoring systems, integrated with sensors and data analytics, provide real-time insights into superheater performance, allowing operators to detect potential issues and optimize operating conditions proactively. These innovations not only enhance the operational efficiency of superheaters but also reduce downtime and maintenance costs.
Superheaters are also integral to the ongoing transition to cleaner and more sustainable energy systems. In biomass and waste-to-energy plants, for example, superheaters enable the efficient conversion of renewable and waste-derived fuels into high-quality steam for power generation. In solar thermal power plants, superheaters are used to increase the temperature of steam produced by solar concentrators, improving the overall efficiency of the energy conversion process. The adaptability of superheaters to various fuel sources and applications highlights their importance in achieving energy efficiency and reducing carbon emissions.
In summary, superheaters are indispensable components in steam generation systems, enhancing the efficiency, reliability, and versatility of steam-driven processes. By producing high-temperature, moisture-free steam, they improve the performance of power plants, industrial machinery, and numerous other applications. While challenges such as overheating, fouling, and energy consumption persist, advancements in materials, design, and monitoring technology continue to drive improvements in their efficiency and sustainability. As industries strive for greater energy efficiency and environmental responsibility, superheaters will remain a cornerstone of modern steam generation systems, playing a crucial role in the advancement of both conventional and renewable energy technologies.
Reheaters
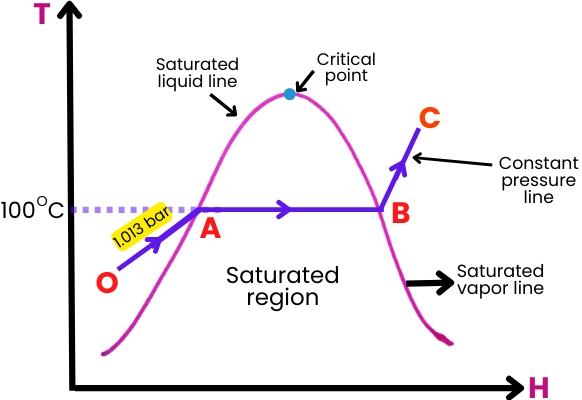
Reheaters are crucial components in modern power plants, particularly in thermal and combined-cycle systems, where they play a significant role in enhancing the efficiency and performance of steam turbines. Their primary function is to reheat partially expanded steam after it exits a high-pressure turbine stage, increasing its temperature before it is directed to subsequent turbine stages. This process reduces the moisture content of the steam, improves thermal efficiency, and minimizes mechanical wear on turbine components, making reheaters an essential element in large-scale power generation systems.
The operation of a reheater involves transferring heat to the low-pressure steam extracted from the high-pressure turbine. Typically, this heat is supplied by the combustion process in the boiler or heat recovery steam generator (HRSG). The reheater is composed of a network of tubes that are exposed to high-temperature flue gases, allowing the steam to absorb the necessary heat through convection and radiation. By raising the temperature of the steam, the reheater ensures that it retains sufficient energy for efficient expansion through the intermediate and low-pressure turbine stages. The amount of reheating required is carefully optimized to balance energy input with the desired thermal efficiency and output of the power plant.
Reheaters are generally classified based on their location and design. In radiant reheaters, the steam absorbs heat directly from the high-temperature radiation zone of the furnace, providing rapid and intense heating. These are typically positioned close to the combustion chamber to maximize exposure to radiant heat. Convective reheaters, on the other hand, rely on the flow of hot flue gases over the reheater tubes, transferring heat through convection. This type of reheater is usually placed farther from the combustion zone, where gas temperatures are lower but still sufficient for effective reheating. Many power plants use a combination of radiant and convective reheaters to achieve precise temperature control and maximize system efficiency.
One of the primary benefits of reheaters is their ability to improve the thermal efficiency of power generation systems. In a Rankine cycle, for example, reheating increases the average temperature at which heat is added to the steam, reducing the amount of fuel required to produce a given amount of electricity. This not only lowers operational costs but also reduces greenhouse gas emissions, making reheaters a vital component in efforts to improve the environmental sustainability of fossil-fuel-based power plants. By minimizing the moisture content of the steam, reheaters also prevent erosion and corrosion of turbine blades, reducing maintenance needs and extending the lifespan of critical equipment.
Reheaters are particularly advantageous in power plants that operate at high pressures and temperatures, where steam tends to lose significant energy during expansion. By reheating the steam, these systems maintain high energy availability throughout the turbine stages, enabling more efficient energy conversion and higher power outputs. This is especially important in supercritical and ultra-supercritical power plants, where the conditions of steam generation demand robust and efficient reheating systems. In combined-cycle power plants, reheaters contribute to the efficient integration of gas and steam turbines by optimizing the energy transfer between the two cycles.
However, the design and operation of reheaters present several challenges that must be carefully addressed to ensure reliable and efficient performance. Overheating is a critical concern, as the high temperatures involved can lead to thermal stresses and material degradation over time. To mitigate this, reheaters are constructed using advanced materials such as high-temperature alloys and are equipped with temperature control systems, such as attemperators, to regulate steam temperatures and prevent overheating. Fouling and slagging caused by ash and soot deposits on reheater tubes can reduce heat transfer efficiency, necessitating regular cleaning and maintenance. Many power plants incorporate soot blowers or other cleaning mechanisms to address this issue.
Energy efficiency is another critical consideration in the design of reheaters. Since reheating requires additional heat input, it is essential to optimize heat transfer and minimize energy losses to maintain cost-effectiveness. Insulation of reheater tubes, efficient combustion control, and waste heat recovery systems are some strategies employed to enhance the energy efficiency of reheaters. Additionally, the placement and arrangement of reheater tubes within the boiler are carefully designed to ensure uniform heating and minimize pressure drops, which can negatively impact turbine performance.
Technological advancements have significantly improved the reliability and efficiency of reheaters. The development of high-performance materials and coatings has increased resistance to thermal stresses, corrosion, and fouling, allowing reheaters to operate at higher temperatures and pressures. The integration of smart monitoring systems, equipped with sensors and data analytics, provides real-time insights into reheater performance, enabling predictive maintenance and optimal operation. These innovations not only enhance the operational efficiency of reheaters but also reduce downtime and maintenance costs, ensuring long-term reliability and sustainability.
Reheaters are also playing an important role in the transition to cleaner and more sustainable energy systems. In biomass and waste-to-energy plants, reheaters enable the efficient use of renewable and waste-derived fuels, contributing to lower carbon emissions and sustainable energy production. In concentrated solar power (CSP) plants, reheaters are used to increase the temperature of steam generated by solar collectors, enhancing the overall efficiency of the energy conversion process. The adaptability of reheaters to various fuel sources and energy systems highlights their importance in meeting the demands of modern power generation while addressing environmental concerns.
In conclusion, reheaters are indispensable components in steam power generation systems, providing essential benefits in terms of thermal efficiency, reliability, and equipment longevity. By reheating partially expanded steam, they enhance the performance of steam turbines, reduce fuel consumption, and minimize environmental impact. While challenges such as overheating, fouling, and energy efficiency persist, advancements in materials, design, and technology continue to drive improvements in reheater performance. As the energy industry evolves toward more efficient and sustainable practices, reheaters will remain a cornerstone of modern power plant design, supporting the efficient and reliable generation of electricity in both conventional and renewable energy systems.
Ducting and Gas Flow System
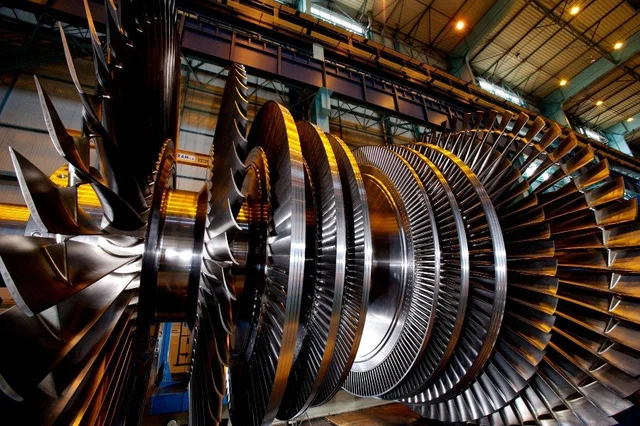
Ducting and gas flow systems are critical components in various industrial and power generation applications, ensuring the controlled movement and management of gases throughout a facility. These systems are designed to transport, regulate, and optimize the flow of gases, such as air, flue gas, or exhaust gases, between different equipment or processes. The performance of ducting and gas flow systems has a direct impact on the efficiency, safety, and environmental compliance of industrial operations, making their design and operation a cornerstone of effective plant management.
The primary purpose of ducting and gas flow systems is to facilitate the smooth and efficient transfer of gases between equipment like boilers, turbines, heat exchangers, scrubbers, and chimneys. In power plants, for example, ducting systems guide combustion air to the boiler, convey flue gases to emission control equipment, and ultimately discharge cleaned gases into the atmosphere through stacks. The design of these systems must ensure minimal pressure losses, uniform flow distribution, and resistance to high temperatures and corrosive substances, all of which are essential for maintaining system efficiency and longevity.
A well-designed ducting system accounts for several critical factors, including gas velocity, temperature, pressure, and composition. The size and geometry of the ducts are carefully engineered to minimize pressure drops and turbulence, which can reduce system efficiency and increase energy consumption. Smooth transitions, rounded corners, and optimized cross-sectional areas are often incorporated into duct designs to achieve laminar flow and avoid hotspots or stagnation points. Additionally, ducting systems are often equipped with flow control devices such as dampers, diverters, and vanes, which regulate gas distribution and ensure consistent performance across varying operating conditions.
Material selection is a crucial aspect of ducting and gas flow system design, as these systems must withstand harsh operational environments. The materials used must be able to endure high temperatures, corrosive gases, and abrasive particles without degrading or failing. Stainless steel, carbon steel, and specialized alloys are commonly used in high-temperature and high-pressure applications, while coatings or linings may be applied to enhance corrosion resistance and extend the system’s service life. For ducts handling abrasive materials or particulates, wear-resistant linings are often employed to prevent erosion and maintain structural integrity.
The integration of advanced monitoring and control systems has greatly improved the efficiency and reliability of ducting and gas flow systems. Sensors installed within the ducts provide real-time data on parameters such as gas velocity, temperature, pressure, and composition, enabling operators to detect anomalies and optimize system performance. Smart controls, often integrated with plant automation systems, allow for precise adjustments to flow rates and distribution, ensuring that the system operates at peak efficiency under varying loads and process conditions. These advancements not only improve operational efficiency but also reduce maintenance costs and downtime by identifying potential issues before they escalate.
Ducting and gas flow systems are also essential in managing environmental compliance, particularly in industries subject to stringent emissions regulations. By ensuring efficient gas flow to pollution control equipment, such as scrubbers, baghouses, or electrostatic precipitators, these systems help minimize the release of harmful pollutants into the atmosphere. Properly designed ducting can prevent bypassing or uneven gas distribution, both of which can reduce the effectiveness of emission control technologies. Additionally, ducting systems often include features like expansion joints, baffles, and insulation to accommodate thermal expansion, reduce noise, and minimize heat losses, further contributing to their environmental performance.
Challenges in the design and operation of ducting and gas flow systems include managing pressure drops, controlling leakage, and minimizing energy losses. Pressure drops can occur due to friction, turbulence, or obstructions within the ducts, leading to higher energy consumption by fans or blowers. Addressing these issues requires careful attention to duct geometry, surface finish, and flow control devices. Leakage, whether caused by poor joint sealing or material failure, can compromise system performance and increase operational costs. The use of high-quality seals, precision fabrication, and regular inspections is essential to maintaining the integrity of the system.
Energy efficiency is a key consideration in the operation of ducting systems, as the movement of gases often requires significant energy input. Fans, blowers, and compressors used to drive gas flow can consume a substantial portion of a facility’s energy budget. Optimizing the design of ducting systems to reduce resistance and pressure drops can significantly lower energy consumption and associated costs. Advanced fan designs, variable frequency drives (VFDs), and energy recovery systems are commonly used to enhance the efficiency of gas flow systems.
The application of ducting and gas flow systems extends across a wide range of industries, including power generation, petrochemicals, cement, metals, and HVAC systems. In power plants, for example, ducting systems guide air and flue gases through boilers, heat recovery steam generators (HRSGs), and emission control equipment. In cement plants, these systems handle hot, dusty gases from kilns and preheaters, ensuring effective heat recovery and pollution control. In HVAC systems, ducting distributes conditioned air to maintain thermal comfort and indoor air quality in buildings and facilities.
In summary, ducting and gas flow systems are indispensable for the efficient and safe operation of industrial and power generation facilities. By facilitating the controlled movement and management of gases, they ensure optimal performance, environmental compliance, and energy efficiency. Challenges such as pressure drops, leakage, and material degradation are addressed through careful design, material selection, and the integration of advanced monitoring and control technologies. As industries continue to prioritize energy efficiency and environmental sustainability, ducting and gas flow systems will remain a critical focus, driving innovation and improvement across a wide range of applications.
Steam Drum
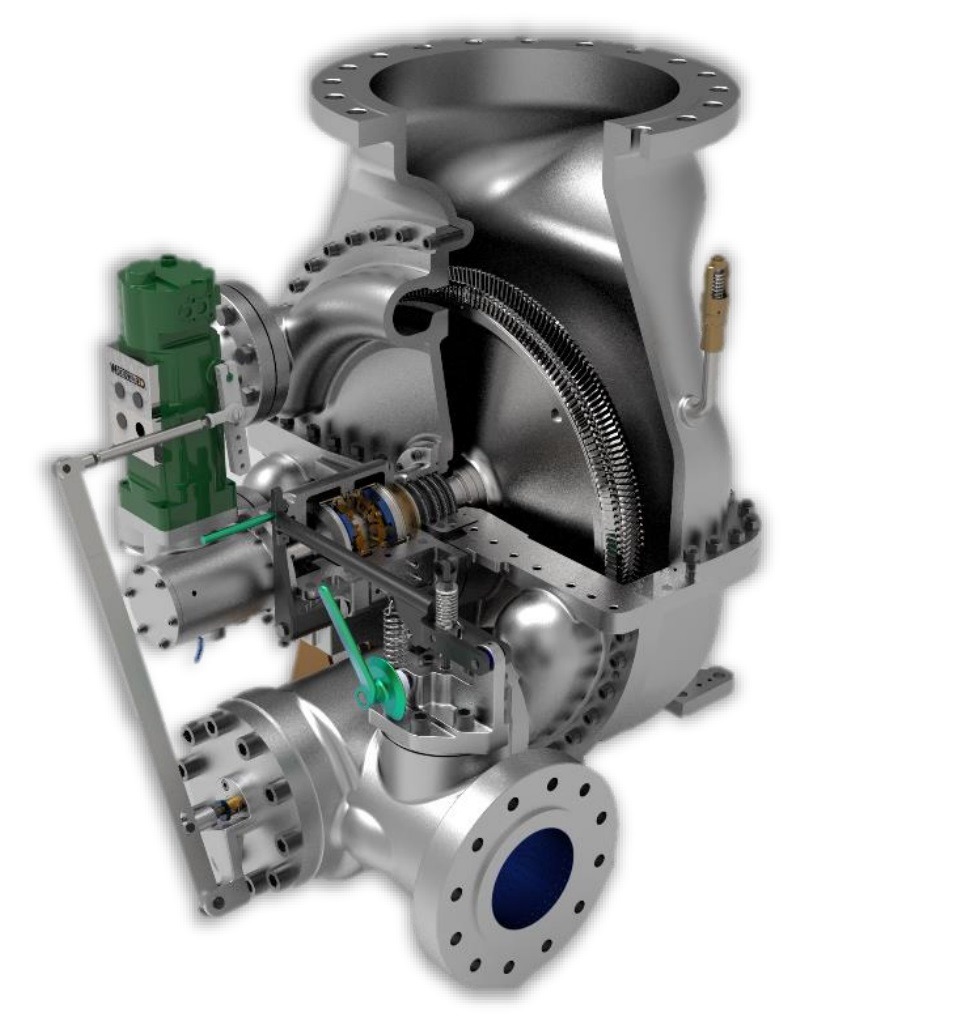
The steam drum is a critical component in steam generation systems, primarily found in water tube boilers, which are commonly used in power plants, industrial heating systems, and large-scale heating applications. The steam drum plays a vital role in separating steam from water, maintaining water levels, and regulating pressure within the boiler system. It is essentially a large cylindrical vessel located at the top of the boiler, where steam and water are separated, and steam is directed into the turbine or other downstream equipment.
The steam drum serves several essential functions. First, it acts as a separator for the steam-water mixture that is produced in the furnace. As water circulates through the tubes of the boiler, it absorbs heat from the combustion gases and begins to turn into steam. The steam and water mixture rises to the steam drum, where gravity and the design of the drum help to separate the steam from the water. The steam, being lighter, rises to the top of the drum, while the water, which is heavier, settles at the bottom. This separation is crucial because it ensures that only dry, saturated steam enters the turbine, reducing the risk of damage to turbine blades caused by water droplets, which can lead to erosion and corrosion.
Another critical function of the steam drum is to maintain water level control. The drum is typically equipped with level sensors that monitor the water level within the drum, ensuring that it remains within safe operational limits. If the water level drops too low, there is a risk of overheating and damaging the boiler tubes, while an excessively high water level can lead to water carryover, where liquid water is carried into the steam system, potentially causing damage to downstream equipment. The steam drum’s level control system is essential for maintaining the integrity and efficiency of the entire boiler system.
The steam drum is also responsible for the removal of impurities in the water. As water is heated and converted to steam, impurities in the water, such as salts, minerals, and other contaminants, tend to concentrate. The steam drum often contains a number of devices, such as blowdown valves and chemical dosing systems, which help remove these impurities from the water. Blowdown valves are used to discharge a portion of the concentrated water from the drum to prevent the buildup of harmful deposits, such as scale and sludge, which can impair heat transfer and reduce the boiler’s efficiency.
In modern steam generation systems, the steam drum is often part of a closed-loop system that works in conjunction with other components such as economizers, superheaters, and reheaters. These components work together to maximize the efficiency of the system by recovering and reusing heat, which helps to reduce fuel consumption and lower emissions. The steam drum’s interaction with these components is critical for maintaining optimal operating conditions within the boiler and ensuring that steam is produced at the desired pressure and temperature for the specific application.
The design of the steam drum takes into account several factors, including the size and capacity of the boiler, the pressure and temperature of the steam, and the characteristics of the fuel being used. Steam drums are typically constructed from high-strength steel alloys that can withstand the high pressures and temperatures involved in the steam generation process. The drum’s capacity must be sufficient to handle the volume of water and steam produced by the boiler, and its shape and dimensions are carefully engineered to allow for effective steam separation and water circulation.
To ensure safety and reliability, the steam drum is equipped with various safety features, including pressure relief valves, safety valves, and emergency shutoff systems. These devices help protect the boiler from overpressure, overheating, and other potentially hazardous conditions. Additionally, the steam drum is subjected to regular maintenance and inspection to detect any signs of wear, corrosion, or damage. Routine checks on the drum’s water level, pressure, and temperature are also conducted to ensure optimal performance and prevent any operational disruptions.
One of the challenges associated with the steam drum is managing the phenomenon of steam-water cycling, which refers to the continuous circulation of water and steam through the boiler system. This cycling process is essential for transferring heat from the combustion gases to the water, but it can also lead to issues such as the deposition of solids and the formation of bubbles that can affect the efficiency of heat transfer. To mitigate these challenges, modern steam drum designs often incorporate advanced technologies, such as advanced water treatment systems and enhanced drum internals, to improve separation efficiency and maintain water quality.
In summary, the steam drum is a vital component of steam generation systems, serving as the vessel where steam and water are separated, and playing an essential role in maintaining water levels, regulating pressure, and ensuring the efficient operation of the entire system. Its design, materials, and operational control systems are crucial for preventing damage to boiler components, maximizing efficiency, and ensuring safe and reliable operation. Through careful monitoring and maintenance, the steam drum helps ensure the long-term performance and reliability of steam boilers in various industrial and power generation applications.
Applications of HRSG
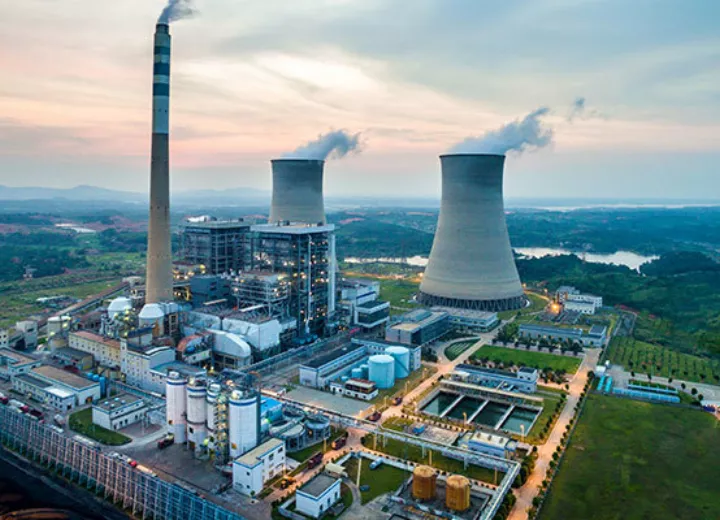
The Heat Recovery Steam Generator (HRSG) is a critical component in modern combined-cycle power plants and other industrial applications where waste heat recovery is essential for improving overall energy efficiency. The HRSG is designed to capture exhaust gases from gas turbines or other high-temperature processes and use this waste heat to produce steam, which can then be used for power generation, heating, or industrial processes. By efficiently recovering and utilizing waste heat, HRSGs contribute to reducing fuel consumption, lowering emissions, and increasing the overall efficiency of power generation systems.
In combined-cycle power plants, the HRSG plays a central role in maximizing the efficiency of the system by converting exhaust gases from the gas turbine into steam. In these plants, a gas turbine burns fuel to produce electricity, and the exhaust gases, which are still at a high temperature, are directed into the HRSG. The HRSG captures this waste heat and uses it to generate steam. This steam is then directed to a steam turbine, which produces additional electricity. By recovering the exhaust heat, HRSGs can significantly increase the overall efficiency of a combined-cycle plant, typically achieving thermal efficiencies of 50% to 60%, compared to around 33% for simple-cycle gas turbine plants. This improved efficiency results in reduced fuel consumption and a lower carbon footprint, making combined-cycle plants with HRSGs an attractive option for power generation.
HRSGs are also used in cogeneration or combined heat and power (CHP) systems, where both electricity and useful thermal energy are produced from the same fuel source. In these applications, the HRSG recovers waste heat from a gas turbine or other sources and produces steam, which can be used for industrial processes, district heating, or other applications requiring heat. Cogeneration systems with HRSGs are widely used in industries such as chemicals, petrochemicals, paper and pulp, and food processing, where both electrical power and thermal energy are needed. In these industries, the integration of an HRSG allows for efficient energy utilization, reducing the need for separate heating and power generation systems, and providing substantial cost savings.
In industrial applications, HRSGs can be integrated into various processes where waste heat is generated. For example, in petroleum refineries, HRSGs are used to recover heat from the flue gases of furnaces, catalytic crackers, or reformers. This recovered heat can then be used to generate steam for use in distillation columns, steam reforming, or other refinery processes. Similarly, in chemical plants, HRSGs are employed to recover waste heat from combustion processes or other high-temperature operations, providing steam for various reactions and separation processes. By recovering waste heat, HRSGs reduce fuel consumption and help refineries and chemical plants minimize energy costs, improve process efficiency, and reduce environmental impact.
In addition to power generation and industrial applications, HRSGs are also used in waste-to-energy plants, where they recover heat from the combustion of municipal solid waste (MSW) or other waste materials. In these plants, the HRSG captures the heat generated by burning waste and uses it to produce steam, which can then be used to drive a steam turbine for electricity generation or provide thermal energy for district heating. HRSGs in waste-to-energy systems contribute to a circular economy by helping to recover energy from waste, reducing the reliance on fossil fuels, and diverting waste from landfills.
HRSGs are also employed in geothermal power plants, where they can be used to capture heat from geothermal fluids. In these applications, the HRSG serves as a heat exchanger that transfers heat from the geothermal fluid to water, producing steam that can then be used to drive a steam turbine for power generation. The use of an HRSG in geothermal systems helps maximize the energy extracted from geothermal sources, improving the overall efficiency of the plant.
One of the key benefits of HRSGs is their ability to integrate with a wide range of heat sources and processes. Whether used in combined-cycle plants, cogeneration systems, waste-to-energy plants, or industrial facilities, HRSGs enable the efficient recovery and utilization of waste heat, improving energy efficiency, reducing emissions, and cutting operational costs. The versatility of HRSGs makes them a valuable tool in achieving more sustainable energy systems and supporting industries in their efforts to reduce energy consumption and environmental impact.
The design of HRSGs is highly adaptable, and they can be tailored to meet the specific needs of different applications. The configuration of the heat recovery system, including the number of pressure levels, the arrangement of heat exchangers, and the use of supplementary firing, is determined based on the temperature and flow characteristics of the exhaust gases, the steam requirements, and the overall system design. HRSGs can be designed for single-pressure, dual-pressure, or triple-pressure operation, depending on the steam demands of the turbine and the efficiency goals of the system. Additionally, the integration of supplemental burners or other heat sources into the HRSG can further enhance performance, allowing for the generation of superheated or reheated steam when required.
While HRSGs offer significant benefits, they also present several challenges. The high temperatures and corrosive nature of exhaust gases can lead to fouling, corrosion, and material degradation over time. To mitigate these issues, HRSGs are typically constructed using high-quality, heat-resistant alloys and are equipped with cleaning and maintenance systems to ensure reliable operation. Furthermore, HRSGs must be carefully designed to accommodate thermal expansion, minimize pressure losses, and ensure efficient heat transfer to maximize overall system efficiency.
In conclusion, the applications of HRSGs are diverse and widespread, contributing to the efficiency and sustainability of power plants, industrial processes, and waste-to-energy systems. By capturing and utilizing waste heat, HRSGs improve energy efficiency, reduce fuel consumption, lower emissions, and provide valuable thermal energy for industrial processes and district heating. Whether in combined-cycle power plants, cogeneration systems, or waste-to-energy facilities, HRSGs play a central role in optimizing energy recovery and supporting efforts to build more sustainable, low-carbon energy systems. As industries and power generation systems continue to evolve toward greater energy efficiency and environmental responsibility, the role of HRSGs will remain integral in achieving these goals.
Energy recovery systems
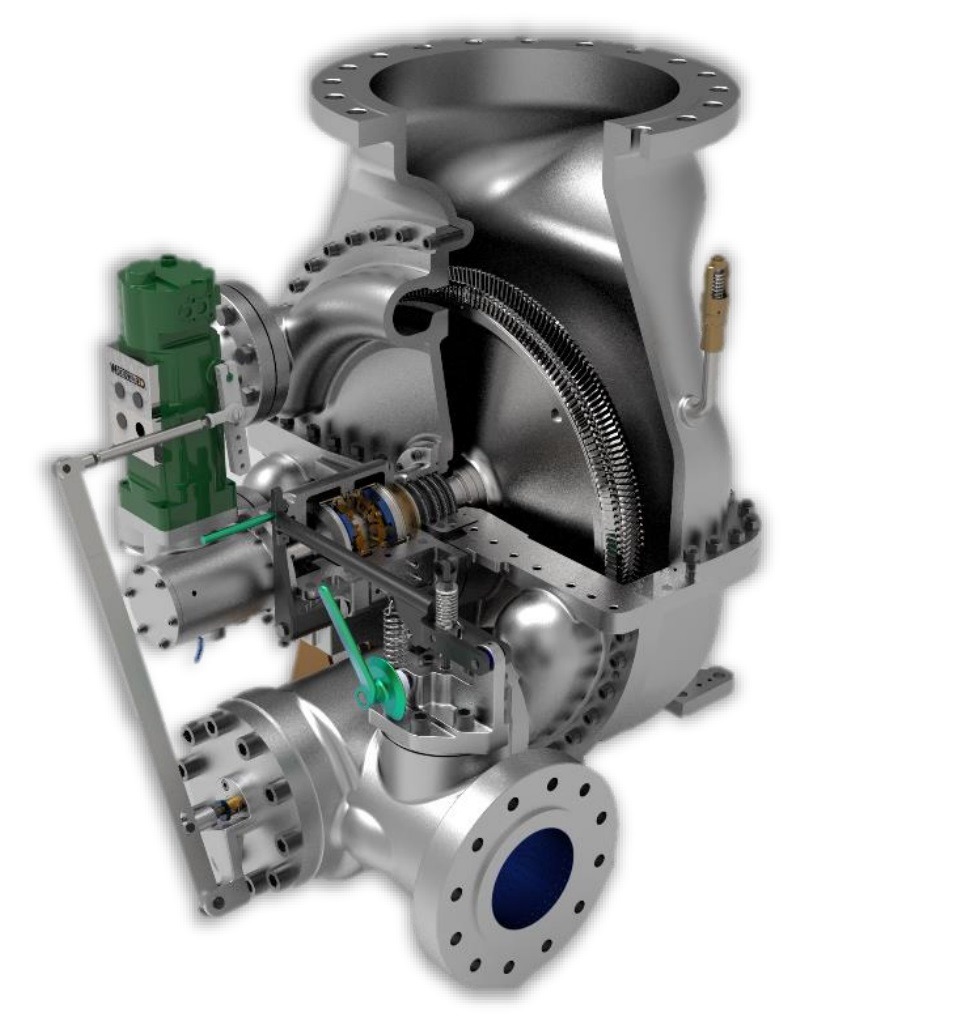
Energy recovery systems (ERS) are vital technologies used across various industries to capture waste energy from processes and convert it into usable forms of energy, such as electricity, heat, or mechanical power. By recovering energy that would otherwise be wasted, these systems contribute to increasing overall efficiency, reducing fuel consumption, and lowering carbon emissions. The widespread application of energy recovery systems helps industries meet environmental sustainability goals while improving their economic performance.
At their core, energy recovery systems are designed to harness energy from sources such as waste heat, exhaust gases, or mechanical energy that would typically be discarded. In many industrial processes, heat is a byproduct of combustion or other high-temperature operations. Instead of allowing this energy to dissipate into the environment, ERS capture it and repurpose it for useful applications. These systems can be found in power plants, manufacturing facilities, chemical plants, HVAC systems, and many other sectors where energy is produced or consumed.
In power plants, energy recovery systems are most commonly used in combined-cycle and cogeneration plants, where waste heat from gas turbines or engines is recovered and converted into steam to drive a steam turbine. Combined-cycle plants, in particular, have a high level of energy recovery by integrating gas turbines and steam turbines to produce more electricity from the same amount of fuel. The gas turbine generates electricity, and its exhaust gases are directed into a heat recovery steam generator (HRSG), which uses the waste heat to generate steam. This steam is then fed to a steam turbine to generate additional power, effectively increasing the plant’s efficiency.
Cogeneration or combined heat and power (CHP) systems are another prominent application of energy recovery systems. In CHP systems, energy recovery is employed to produce both electricity and thermal energy (heat) from the same fuel source. The recovered heat can be used for industrial processes, space heating, or district heating, allowing for efficient use of energy that would otherwise be wasted. This approach is widely used in industries such as chemical manufacturing, food processing, and paper production, where both electrical power and heat are necessary. By implementing ERS in CHP systems, industries can significantly reduce energy consumption, lower costs, and reduce their environmental impact.
Energy recovery systems are also essential in waste-to-energy (WTE) plants. These plants use ERS to convert waste materials, such as municipal solid waste (MSW), biomass, or industrial waste, into useful energy. In waste-to-energy applications, the waste is burned to produce heat, and the energy recovery system captures this heat to produce steam, which can then be used to generate electricity or provide thermal energy for district heating. This process not only helps reduce the volume of waste sent to landfills but also generates renewable energy from otherwise discarded materials, contributing to a more sustainable energy system.
In industrial applications, energy recovery systems are used to capture waste heat from furnaces, kilns, and other high-temperature processes. For example, in the cement industry, ERS capture heat from the exhaust gases of rotary kilns, which are then used to generate steam or provide thermal energy for other stages of the cement production process. In the petrochemical industry, waste heat recovery is common in refining processes, where it helps power distillation columns, reformers, and other units. By using energy recovery systems, industries can significantly reduce their reliance on external energy sources and decrease operational costs, all while reducing their carbon footprint.
In addition to thermal energy recovery, there are mechanical and electrical energy recovery systems, which focus on recovering energy from mechanical processes, such as rotating machinery or pressurized fluids. For example, in hydraulic systems, energy recovery devices such as pumps or turbines can capture energy from high-pressure fluids and convert it into electrical power or stored energy. Similarly, regenerative braking systems in electric vehicles and trains are another example of mechanical energy recovery, where kinetic energy is captured during braking and converted into electrical energy to recharge the battery.
HVAC systems, particularly in large buildings or industrial settings, also benefit from energy recovery systems. In these applications, heat recovery ventilators (HRVs) or energy recovery ventilators (ERVs) capture heat from exhaust air and transfer it to incoming fresh air. This process helps maintain indoor air quality while minimizing the energy required to heat or cool the incoming air. Energy recovery systems in HVAC applications contribute to reducing energy consumption for heating and cooling, improving system efficiency, and enhancing occupant comfort.
One of the most common forms of energy recovery systems is the heat exchanger. Heat exchangers are used in a variety of applications to recover waste heat from exhaust gases, fluids, or other sources. For example, in industrial processes, air-to-air, air-to-water, or liquid-to-liquid heat exchangers capture heat from exhaust streams and transfer it to the incoming air or fluids. The recovered heat can be used to preheat process fluids, reducing the need for additional heating energy. Similarly, in power generation plants, air preheaters and economizers use heat exchangers to recover heat from flue gases to preheat the combustion air, improving the combustion process and overall system efficiency.
Another important technology in energy recovery is the organic Rankine cycle (ORC), which allows for the recovery of low-grade waste heat and its conversion into electricity. The ORC operates similarly to a steam turbine system but uses an organic fluid instead of water, which has a lower boiling point. This allows the ORC to recover waste heat from lower-temperature sources, such as industrial processes, geothermal energy, or even solar thermal systems, converting the heat into useful electricity. ORC systems are increasingly being used in industries such as chemical manufacturing, food processing, and waste-to-energy plants.
Energy recovery systems are essential for improving overall energy efficiency, reducing costs, and minimizing environmental impacts. They help conserve energy, lower fuel consumption, and reduce greenhouse gas emissions by making better use of available resources. While the initial investment in energy recovery technologies can be substantial, the long-term benefits in terms of energy savings and operational efficiency make them a smart investment for industries looking to improve their sustainability profile.
Despite their many benefits, energy recovery systems present several challenges. The efficiency of energy recovery can be influenced by factors such as temperature differentials, system design, and the type of energy being recovered. Additionally, maintaining and optimizing energy recovery systems often requires specialized knowledge and technology, as well as careful monitoring and control systems to ensure maximum efficiency. Regular maintenance is essential to prevent issues such as fouling, corrosion, or wear, which can reduce the effectiveness of these systems over time.
In conclusion, energy recovery systems play an essential role in maximizing efficiency and sustainability across various industries and applications. Whether through waste heat recovery, mechanical energy capture, or electrical energy conversion, ERS contribute to reducing energy consumption, lowering emissions, and supporting the transition to more sustainable energy practices. As technology continues to evolve, the capabilities of energy recovery systems will expand, further driving improvements in energy efficiency and environmental responsibility across industrial, commercial, and power generation sectors.
Introduction to Energy Recovery Systems
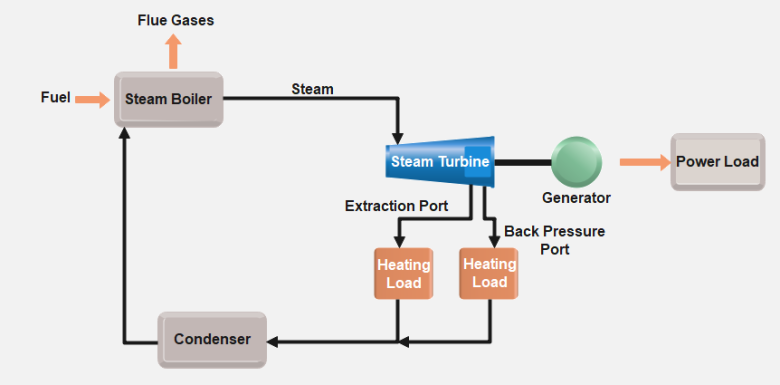
Energy Recovery Systems (ERS) are systems designed to capture waste energy from various industrial and power generation processes and convert it into useful forms of energy, such as electricity, heat, or mechanical power. These systems are essential for improving overall energy efficiency, reducing fuel consumption, and lowering environmental impacts. By recovering energy that would otherwise be wasted, ERS help industries and power plants achieve more sustainable operations, lower operating costs, and comply with increasingly stringent environmental regulations.
Types of Energy Recovery Systems
Energy recovery systems can be categorized based on the type of energy they recover and the method used to convert this energy into a usable form. These include thermal energy recovery, mechanical energy recovery, and electrical energy recovery systems.
- Thermal Energy Recovery: This is the most common form of energy recovery, where waste heat from exhaust gases, industrial processes, or combustion is captured and used for heating, steam generation, or preheating. Examples include heat recovery steam generators (HRSGs), economizers, and heat exchangers.
- Mechanical Energy Recovery: Mechanical energy recovery involves capturing energy from moving mechanical systems, such as rotating machinery, pumps, or turbines. An example of this is regenerative braking systems, which recover kinetic energy during braking and convert it into electrical energy.
- Electrical Energy Recovery: Electrical energy recovery systems capture energy from electrical systems or processes and convert it back into usable electrical power. These systems can include devices such as flywheels, which store mechanical energy for later conversion into electricity.
Applications of Energy Recovery Systems
Energy recovery systems are applied in a wide range of industries and energy systems to optimize energy use and reduce waste. Common applications include:
- Combined-Cycle Power Plants: In combined-cycle power plants, energy recovery systems such as Heat Recovery Steam Generators (HRSGs) capture waste heat from gas turbines and convert it into steam to drive a steam turbine, thereby improving the overall efficiency of the power generation process.
- Cogeneration and Combined Heat and Power (CHP) Systems: These systems generate both electricity and useful thermal energy from a single fuel source. The energy recovery system captures waste heat and uses it for heating, district heating, or industrial processes, contributing to significant energy savings and cost reduction.
- Waste-to-Energy Plants: In waste-to-energy plants, energy recovery systems capture heat from the combustion of waste materials such as municipal solid waste (MSW) or biomass. This recovered heat is used to generate electricity or provide thermal energy for district heating.
- Industrial Applications: Industries such as cement, petrochemical, and steel manufacturing use energy recovery systems to capture heat from high-temperature processes like furnaces or kilns. This recovered energy can be used for preheating, steam generation, or other applications, reducing energy consumption and operating costs.
- Geothermal and Biomass Energy Systems: In geothermal plants, energy recovery systems such as Organic Rankine Cycles (ORC) are used to convert low-grade waste heat into electricity. Biomass plants also use energy recovery systems to capture and convert heat from biomass combustion into electricity or thermal energy.
- HVAC Systems: Energy recovery ventilators (ERVs) and heat recovery ventilators (HRVs) in HVAC systems capture and reuse heat from exhaust air to preheat or precool incoming fresh air, reducing the energy needed for heating or cooling and improving system efficiency.
Benefits of Energy Recovery Systems
The integration of energy recovery systems provides numerous benefits, both economically and environmentally:
- Increased Energy Efficiency: By recovering and utilizing waste energy, ERS significantly boost the overall efficiency of industrial processes, power generation, and other energy systems. This leads to less fuel consumption and reduced operational costs.
- Reduced Environmental Impact: Energy recovery systems reduce the reliance on fossil fuels and help lower greenhouse gas emissions. By converting waste energy into usable forms, ERS contribute to cleaner, more sustainable energy production.
- Cost Savings: By capturing waste heat and converting it into electricity or thermal energy, ERS help businesses reduce their reliance on external energy sources, leading to long-term cost savings in energy bills.
- Sustainability: Energy recovery systems are key to the transition to more sustainable energy practices, reducing the need for new fuel sources and minimizing waste. This contributes to both economic sustainability and environmental stewardship.
Challenges of Energy Recovery Systems
While energy recovery systems provide substantial benefits, they also present several challenges:
- System Complexity: The design, integration, and maintenance of ERS can be complex, requiring specialized knowledge and technology. Optimizing ERS for a specific application or process often involves extensive engineering and system integration.
- Initial Costs: The installation of energy recovery systems can be expensive, especially in large-scale applications like power plants or industrial facilities. However, the long-term energy savings and operational efficiencies typically outweigh the initial investment.
- Efficiency Limitations: The efficiency of energy recovery systems can be influenced by factors such as temperature gradients, process variability, and the type of waste energy being captured. Some systems may not recover enough energy to justify the investment if not properly designed.
- Maintenance and Operation: Energy recovery systems require regular maintenance to ensure optimal performance. Fouling, corrosion, and wear can degrade system performance over time, necessitating frequent cleaning, inspections, and component replacements.
Technologies Used in Energy Recovery Systems
Several technologies are employed to capture and convert waste energy, each suited to different applications:
- Heat Recovery Steam Generators (HRSGs): HRSGs are commonly used in combined-cycle power plants and cogeneration systems. They capture waste heat from gas turbines or other sources and convert it into steam, which can be used for electricity generation or industrial processes.
- Organic Rankine Cycle (ORC): The ORC is used to recover waste heat from lower-temperature sources (such as industrial processes or geothermal fluids) and convert it into electricity. It uses an organic fluid with a lower boiling point than water, enabling it to operate at lower temperatures.
- Heat Exchangers: Heat exchangers are widely used in energy recovery applications to transfer heat from exhaust gases or other fluids to a secondary fluid, which can then be used for heating or steam generation. These devices are essential in many industrial applications and power plants.
- Regenerative Braking Systems: In mechanical energy recovery, regenerative braking systems capture kinetic energy during braking and convert it into electrical energy, which can be stored and reused. This technology is common in electric vehicles and rail systems.
- Flywheels and Batteries: Flywheels and advanced battery systems are used to store recovered mechanical or electrical energy for later use. These systems are employed in applications where energy needs to be captured and stored for future demand.
Future of Energy Recovery Systems
The future of energy recovery systems looks promising as industries continue to prioritize energy efficiency and sustainability. Emerging technologies such as advanced thermoelectric generators, improved ORC systems, and smart energy recovery networks are expected to further enhance the capabilities of ERS. Additionally, the increasing focus on renewable energy sources, such as wind, solar, and biomass, will drive the development of more efficient energy recovery solutions.
As regulatory frameworks become more stringent and the demand for sustainable energy solutions grows, the adoption of energy recovery systems will likely become more widespread. Industries will increasingly seek ways to recover and utilize waste energy to minimize costs, reduce emissions, and contribute to a more sustainable global energy system.
Conclusion
Energy recovery systems are essential tools for improving the energy efficiency, sustainability, and economic performance of industrial, commercial, and power generation systems. By capturing and repurposing waste energy, ERS help reduce fuel consumption, minimize environmental impact, and provide valuable thermal or electrical energy for various applications. While challenges such as system complexity and maintenance exist, the long-term benefits of energy recovery systems make them a smart investment for industries aiming to improve energy efficiency, reduce operating costs, and contribute to environmental sustainability. As technology advances and energy needs evolve, energy recovery systems will play a crucial role in shaping the future of energy management across sectors.
Introduction to Thermal Energy Recovery
Thermal energy recovery refers to the process of capturing waste heat that is typically lost during industrial processes, power generation, or other high-temperature operations, and converting it into usable forms of energy, such as steam, hot water, or electricity. By utilizing this waste heat, thermal energy recovery systems help improve the overall energy efficiency of systems and reduce reliance on external energy sources. The integration of thermal energy recovery is a key strategy for reducing energy costs, lowering emissions, and improving sustainability in various sectors, including manufacturing, power generation, and industrial processing.
Types of Thermal Energy Recovery Systems
Thermal energy recovery systems vary depending on the application and the type of heat being recovered. These systems are designed to capture heat from exhaust gases, hot liquids, or other sources of thermal waste. The most common types of thermal energy recovery systems include:
- Heat Recovery Steam Generators (HRSGs): HRSGs are used in combined-cycle power plants to recover waste heat from gas turbines. The exhaust gases from the turbine are passed through the HRSG, where heat is transferred to water or steam, which can then be used to drive a steam turbine for additional power generation.
- Heat Exchangers: Heat exchangers are devices that transfer heat from one fluid to another without mixing the fluids. These are widely used in industrial processes to capture heat from exhaust gases or fluids and transfer it to a secondary fluid for use in heating, preheating, or steam generation.
- Economizers: Economizers are heat exchangers designed to capture waste heat from flue gases or exhaust air in industrial or power generation systems. The recovered heat is typically used to preheat the combustion air, which improves the efficiency of the combustion process.
- Organic Rankine Cycle (ORC): The ORC is a technology used to recover low-grade waste heat and convert it into electricity. The process uses an organic fluid with a low boiling point, making it ideal for capturing heat from industrial processes or renewable energy sources like geothermal or biomass.
- Thermal Storage Systems: Thermal energy storage systems allow heat to be stored and used at a later time. These systems capture and store excess heat during periods of low demand and release it during periods of high demand, ensuring a consistent supply of thermal energy.
- Regenerative Heat Exchangers: These systems are designed to capture heat from exhaust gases and store it temporarily before transferring it to incoming air or fluids. This process is often used in industrial and HVAC applications to improve overall system efficiency.
Applications of Thermal Energy Recovery
Thermal energy recovery systems are used across a wide range of industries and applications, with the goal of improving energy efficiency and reducing operational costs. Some common applications include:
- Power Generation: In power plants, thermal energy recovery is most commonly implemented through the use of Heat Recovery Steam Generators (HRSGs) and economizers. HRSGs capture waste heat from gas turbines, converting it into steam to drive a steam turbine for additional power generation. This improves the efficiency of combined-cycle power plants, where both gas and steam turbines are used in tandem to generate electricity.
- Industrial Manufacturing: Many industries, such as cement, steel, and chemical manufacturing, produce large amounts of waste heat during production processes. Thermal energy recovery systems capture this heat from furnaces, kilns, and other high-temperature equipment and use it to preheat materials or generate steam for industrial processes. This reduces the need for external energy sources, lowering operating costs and improving process efficiency.
- District Heating: Thermal energy recovery systems are widely used in district heating systems, where heat is recovered from power plants or industrial processes and distributed to residential and commercial buildings for space heating or hot water. This system helps optimize the use of available energy by providing both electricity and thermal energy from the same fuel source.
- Waste-to-Energy (WTE) Plants: Waste-to-energy plants use thermal energy recovery systems to capture heat from the combustion of municipal solid waste (MSW) or biomass. The recovered heat is used to generate steam, which can be used to produce electricity or provide thermal energy for district heating or industrial processes.
- Renewable Energy Systems: Thermal energy recovery is also applied in renewable energy systems, such as geothermal power plants and solar thermal systems. In geothermal plants, thermal energy recovery systems capture heat from geothermal fluids and use it to generate electricity or provide direct heating. Similarly, solar thermal systems capture heat from the sun and convert it into usable thermal energy.
- HVAC and Building Systems: In heating, ventilation, and air conditioning (HVAC) systems, thermal energy recovery is used to capture waste heat from exhaust air and transfer it to incoming fresh air, reducing the energy required for heating or cooling. This helps reduce energy consumption in large buildings, industrial facilities, and commercial spaces.
Benefits of Thermal Energy Recovery
Thermal energy recovery offers several significant benefits that contribute to both economic and environmental goals:
- Improved Energy Efficiency: By recovering and reusing waste heat, thermal energy recovery systems significantly improve the overall efficiency of industrial processes, power generation, and building systems. This helps reduce energy consumption, lower operational costs, and optimize resource use.
- Cost Reduction: The integration of thermal energy recovery systems reduces the need for external heating and cooling sources, leading to substantial cost savings over time. For example, by preheating combustion air or process materials, energy recovery systems reduce fuel consumption and energy costs in manufacturing operations.
- Reduced Environmental Impact: The use of thermal energy recovery systems helps reduce greenhouse gas emissions by minimizing the need for additional fuel consumption. By recovering heat that would otherwise be wasted, these systems contribute to lower carbon footprints and more sustainable energy practices.
- Increased Reliability: Thermal energy recovery systems help improve the reliability of energy systems by ensuring a more consistent supply of heat or steam for industrial processes and power generation. In district heating systems, for instance, thermal storage systems can store excess heat for later use, ensuring a steady energy supply during peak demand periods.
- Sustainability: By reducing waste and making more efficient use of available resources, thermal energy recovery contributes to a more sustainable energy system. The ability to reuse heat reduces the need for new energy production and supports the transition to more sustainable, low-carbon energy solutions.
Challenges of Thermal Energy Recovery
While thermal energy recovery offers significant advantages, several challenges must be addressed for successful implementation:
- Capital Investment: The initial cost of installing thermal energy recovery systems can be high, particularly in large-scale industrial or power generation applications. However, the long-term energy savings and operational efficiencies typically outweigh the initial investment.
- Heat Source Temperature and Availability: The effectiveness of thermal energy recovery systems is often dependent on the temperature and availability of the waste heat source. Low-grade waste heat may require more advanced technologies, such as Organic Rankine Cycles (ORC), to recover efficiently.
- System Complexity and Integration: Integrating thermal energy recovery systems into existing industrial or power generation infrastructure can be complex. These systems often require specialized knowledge and engineering to ensure proper design, operation, and integration with other systems.
- Maintenance and Operation: Thermal energy recovery systems require regular maintenance to ensure optimal performance. Fouling, corrosion, and wear of heat exchangers or other components can reduce the efficiency of the system over time, requiring frequent cleaning, inspections, and repairs.
Technologies Used in Thermal Energy Recovery
Several technologies are employed to recover and utilize thermal energy efficiently:
- Heat Recovery Steam Generators (HRSGs): HRSGs are commonly used in combined-cycle power plants to recover heat from exhaust gases and generate steam. This steam can then be used to power a steam turbine or for industrial processes.
- Heat Exchangers: Heat exchangers are versatile devices used in a variety of applications to capture and transfer heat from exhaust gases or liquids to other fluids, enabling preheating, steam generation, or thermal energy use in industrial processes.
- Economizers: Economizers are heat exchangers that recover heat from flue gases and use it to preheat combustion air or process fluids, improving the efficiency of industrial and power generation systems.
- Organic Rankine Cycle (ORC): The ORC technology recovers low-grade waste heat and converts it into electricity by using organic fluids that have lower boiling points than water. This technology is well-suited for capturing heat from industrial processes, geothermal systems, or biomass.
- Thermal Storage Systems: Thermal storage systems allow excess heat to be stored and used when demand is higher. These systems can use molten salts, phase-change materials, or other mediums to store and release heat efficiently.
Conclusion
Thermal energy recovery plays a crucial role in improving the energy efficiency, sustainability, and cost-effectiveness of industrial processes, power generation systems, and buildings. By capturing and reusing waste heat, these systems reduce energy consumption, lower operational costs, and help industries meet environmental sustainability goals. As technology advances and energy efficiency becomes an even greater priority, the role of thermal energy recovery systems will continue to grow, driving further improvements in energy management and reducing the environmental impact of industrial and power generation activities. With its broad range of applications and significant benefits, thermal energy recovery is a key component in the transition to a more sustainable and efficient energy future.
Introduction to Combined-Cycle Power Plants
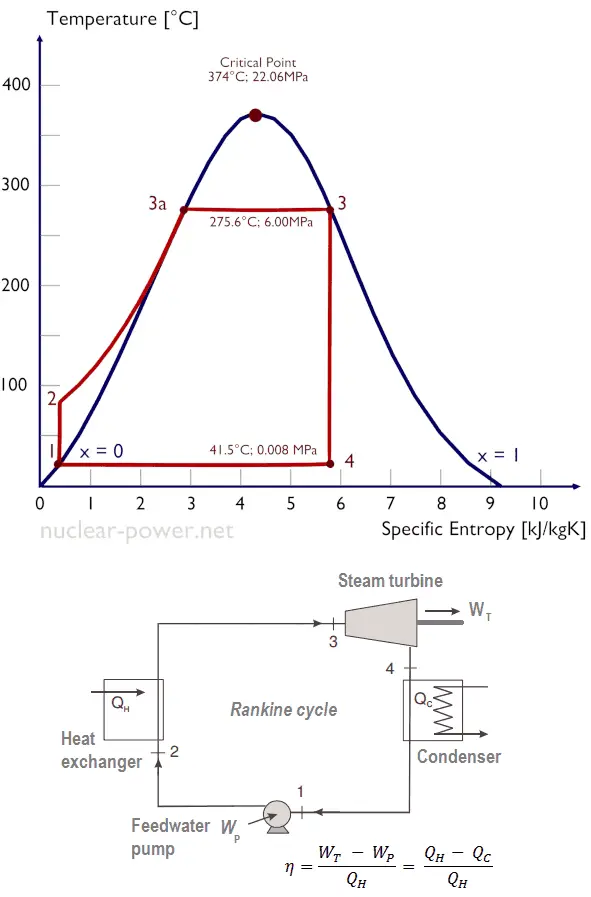
Combined-cycle power plants (CCPPs) are highly efficient power generation systems that combine two or more thermodynamic cycles to generate electricity. These plants integrate both gas and steam turbines, using the waste heat from the gas turbine to generate steam for a steam turbine, thereby improving overall efficiency compared to traditional single-cycle power plants. By utilizing both the Brayton cycle (for the gas turbine) and the Rankine cycle (for the steam turbine), combined-cycle plants can achieve much higher thermal efficiency, typically between 50% and 60%, compared to the 30% to 40% efficiency of conventional thermal power plants. This makes them one of the most efficient and cost-effective ways of generating electricity, with a reduced environmental footprint.
Components of Combined-Cycle Power Plants
Combined-cycle power plants typically consist of the following key components:
- Gas Turbine: The primary source of power in a combined-cycle plant, the gas turbine operates on the Brayton cycle. It burns natural gas or another fuel to produce high-temperature exhaust gases, which are then used to drive the turbine and generate electricity. The gas turbine’s exhaust gases contain substantial waste heat, which is captured and used to produce steam for the steam turbine.
- Heat Recovery Steam Generator (HRSG): The HRSG is a heat exchanger that captures waste heat from the gas turbine exhaust gases and uses it to convert water into steam. The steam produced in the HRSG is then sent to the steam turbine. The HRSG plays a crucial role in improving the efficiency of the plant by utilizing waste heat that would otherwise be lost.
- Steam Turbine: The steam turbine operates on the Rankine cycle, where steam produced by the HRSG is used to drive the turbine and generate additional electricity. The steam turbine is typically designed to operate at lower temperatures and pressures than the gas turbine but can still contribute significantly to the plant’s overall power output.
- Generator: Both the gas and steam turbines are connected to electrical generators, which convert the mechanical energy of the turbines into electrical energy. The combined output from the two turbines is added together to produce a higher overall power output.
- Condenser: After the steam passes through the steam turbine, it is condensed back into water in the condenser. This water is then pumped back into the HRSG for reheating, completing the Rankine cycle. The condenser typically uses cooling water or air to cool the steam.
- Cooling System: The cooling system, which may include cooling towers or air-cooled heat exchangers, is used to reject the heat absorbed by the condenser and maintain the necessary temperature for efficient operation of the Rankine cycle.
Operational Process of Combined-Cycle Power Plants
The operation of a combined-cycle power plant is designed to maximize efficiency by making use of both the gas and steam turbines in a complementary manner:
- Gas Turbine Operation: The gas turbine burns fuel (usually natural gas) to produce high-temperature exhaust gases. These gases are used to spin the turbine, which drives a generator to produce electricity. While the gas turbine is highly efficient in converting fuel to mechanical energy, it still produces a significant amount of waste heat in the exhaust gases.
- Heat Recovery: The waste heat from the gas turbine exhaust gases is directed into the HRSG, where it is used to convert water into steam. The HRSG recovers this heat, which would otherwise be lost to the environment, and uses it to create steam at a high pressure and temperature.
- Steam Turbine Operation: The high-pressure steam produced in the HRSG is sent to the steam turbine, which spins and drives a second generator to produce additional electricity. This effectively increases the overall efficiency of the plant by utilizing the waste heat from the gas turbine.
- Condensation and Recirculation: After passing through the steam turbine, the steam is condensed back into water in the condenser. This water is then pumped back into the HRSG to be reheated, completing the cycle. The continuous operation of the gas turbine and steam turbine maximizes the use of available fuel.
Advantages of Combined-Cycle Power Plants
- High Efficiency: Combined-cycle power plants are much more efficient than conventional single-cycle plants. By recovering waste heat from the gas turbine and using it to produce steam for the steam turbine, CCPPs can achieve efficiencies of 50-60%, which is significantly higher than the 30-40% efficiency of conventional thermal plants. This results in more electricity generated per unit of fuel consumed.
- Reduced Fuel Consumption: Because combined-cycle plants operate more efficiently, they require less fuel to produce the same amount of electricity. This translates to lower operational costs and a reduction in fuel consumption, which is especially beneficial in an era of rising fuel prices.
- Lower Environmental Impact: Combined-cycle power plants produce fewer emissions compared to conventional plants. The higher efficiency means that less fuel is burned for the same amount of electricity, leading to a reduction in greenhouse gas emissions. Additionally, the use of natural gas, which produces fewer carbon emissions than coal or oil, further enhances the environmental benefits.
- Flexibility: Combined-cycle power plants can be designed for both base-load and peaking operations. They are capable of quickly adjusting to changes in power demand, making them suitable for grid stabilization and meeting fluctuating energy needs. This makes CCPPs an ideal complement to intermittent renewable energy sources like wind and solar power.
- Fuel Flexibility: While natural gas is the most common fuel for combined-cycle power plants, these systems can also operate on alternative fuels, such as biogas, hydrogen, or synthetic fuels, making them adaptable to changing fuel availability and supporting the transition to cleaner energy sources.
- Cost-Effective: Due to their high efficiency and relatively low operational and fuel costs, combined-cycle power plants are a cost-effective option for power generation. They are particularly attractive for utilities seeking to optimize their energy mix and reduce overall energy production costs.
Challenges of Combined-Cycle Power Plants
- Capital Costs: Although combined-cycle plants are more efficient and cost-effective in the long run, they tend to have higher initial capital costs compared to single-cycle plants. The complexity of the system, with the integration of both gas and steam turbines, HRSGs, and additional components, requires significant investment upfront.
- Water Consumption: The steam turbine cycle in combined-cycle plants typically requires large amounts of water for cooling in the condenser. This can be a challenge in regions with limited water resources or in areas subject to environmental regulations regarding water usage.
- Maintenance and Reliability: Combined-cycle plants, with their multiple turbine systems and associated components, require regular maintenance to ensure optimal performance. The operation of both gas and steam turbines increases the complexity of maintenance, and unplanned downtime can result in higher operational costs.
- Startup Time: While combined-cycle plants are flexible in terms of adjusting to changes in demand, they may still require a longer time to start up compared to simple-cycle plants. This can be a limitation in systems that need to rapidly respond to demand spikes.
Technologies Used in Combined-Cycle Power Plants
- Heat Recovery Steam Generators (HRSGs): HRSGs are a core technology in combined-cycle plants, as they capture and utilize waste heat from the gas turbine exhaust. These systems vary in design based on the temperature and pressure of the exhaust gases, with designs ranging from simple to complex depending on the level of heat recovery required.
- Organic Rankine Cycle (ORC): In some combined-cycle plants, the Organic Rankine Cycle (ORC) is used to capture lower-temperature waste heat from the exhaust gases. ORC technology uses organic fluids with lower boiling points than water to generate electricity from waste heat at lower temperatures, making it an ideal complement to combined-cycle plants.
- Advanced Gas Turbines: Gas turbines used in combined-cycle plants have become more advanced, with improvements in materials, combustion processes, and turbine design. Modern gas turbines operate at higher temperatures and pressures, increasing efficiency and reducing fuel consumption.
- Combined Heat and Power (CHP): In some applications, combined-cycle plants are integrated with combined heat and power (CHP) systems, which provide both electricity and useful thermal energy for industrial processes, district heating, or other applications. CHP systems further increase the efficiency and versatility of combined-cycle power plants.
Future of Combined-Cycle Power Plants
The future of combined-cycle power plants is closely tied to advancements in technology and the global transition to cleaner energy. With a growing emphasis on reducing greenhouse gas emissions, combined-cycle plants are likely to play an essential role in the decarbonization of the power generation sector, especially when fueled by natural gas or hydrogen.
New technologies such as carbon capture and storage (CCS), improved gas turbine designs, and hybrid systems combining renewable energy sources with natural gas will enhance the performance of combined-cycle plants. Furthermore, with the increasing interest in using hydrogen as a fuel source, there is potential for combined-cycle plants to adapt to hydrogen combustion, significantly reducing their carbon footprint and contributing to a more sustainable energy future.
Conclusion
Combined-cycle power plants are among the most efficient and cost-effective means of generating electricity today. By integrating both gas and steam turbines, combined-cycle plants utilize waste heat from the gas turbine to generate additional power, leading to higher overall efficiency. With their ability to reduce fuel consumption, lower emissions, and provide flexibility for power generation, combined-cycle plants are poised to remain a cornerstone of modern power generation. Despite challenges such as high capital costs and water usage, the benefits of combined-cycle plants make them an attractive option for utilities and industries seeking to optimize their energy production and reduce their environmental impact.
Introduction to Cogeneration and Combined Heat and Power (CHP) Systems

Cogeneration, also known as combined heat and power (CHP), is the simultaneous production of electricity and useful heat from a single energy source. Unlike conventional power plants that only produce electricity and discard excess heat as waste, CHP systems are designed to capture this waste heat and use it for heating, cooling, or industrial processes, significantly improving energy efficiency. This approach maximizes the use of fuel by providing two valuable outputs—electricity and thermal energy—thereby reducing overall energy consumption and environmental impact. Cogeneration systems are used across a variety of sectors, including industrial, commercial, residential, and district energy applications.
Components of Cogeneration and CHP Systems
Cogeneration and CHP systems can be implemented using different technologies, but they generally include a few core components that enable the simultaneous production of electricity and heat:
- Prime Mover: The prime mover is the engine or turbine that drives the generator to produce electricity. Common types of prime movers in CHP systems include:
- Gas Turbines: Often used in larger-scale industrial CHP applications, where natural gas or other fuels are burned to generate high-temperature exhaust gases that drive a turbine and produce electricity.
- Internal Combustion Engines: These engines are typically used in smaller CHP systems, such as in residential or commercial buildings. They burn fuel (usually natural gas, diesel, or biogas) to generate electricity.
- Steam Turbines: In some CHP systems, steam turbines are used to generate electricity from steam produced by burning fuel or recovering waste heat.
- Microturbines: Small-scale turbines that generate electricity from natural gas or other fuels, often used in smaller CHP systems for industrial or commercial purposes.
- Generator: The generator is connected to the prime mover and converts mechanical energy into electrical energy. In a CHP system, the generator produces electricity, which can be used on-site or sold to the grid.
- Heat Recovery System: The heat recovery system captures waste heat from the exhaust gases, cooling system, or the engine’s own combustion process and converts it into usable thermal energy. This heat can be used for space heating, hot water, or industrial processes. The system typically includes:
- Heat Exchanger: A device that transfers heat from the exhaust gases or engine cooling system to water or another heat-carrying fluid.
- Thermal Storage: In some CHP systems, thermal energy is stored in tanks or other media for later use, which helps smooth out fluctuations in heat demand.
- Distribution System: The distribution system carries both electricity and heat to the areas where they are needed. In large industrial or district energy applications, this may include a network of pipes for hot water or steam and electrical transmission lines for power.
- Control System: The control system monitors and manages the operation of the entire CHP plant. It ensures that both power generation and heat recovery occur efficiently and that the system adapts to fluctuations in energy demand.
Operational Process of Cogeneration and CHP Systems
Cogeneration and CHP systems work by capturing and using waste heat that would otherwise be discarded in a conventional power generation process. Here’s how they typically operate:
- Energy Input: Fuel (typically natural gas, biomass, or even waste heat) is supplied to the prime mover, which is either a turbine, engine, or other mechanical device that converts fuel into mechanical energy.
- Power Generation: The prime mover drives the generator, producing electricity that can be used on-site to power industrial processes, buildings, or facilities. In some cases, excess electricity is fed back into the grid.
- Heat Recovery: The waste heat from the prime mover (from exhaust gases, cooling systems, or combustion processes) is captured by the heat recovery system. This heat is then transferred to water or another medium, which can be used for heating buildings, providing hot water, or supporting industrial processes.
- Distribution of Heat: The recovered heat is distributed through a network of pipes or ducts to areas that require heating or hot water. In larger industrial applications, the thermal energy may be used for process heating or for drying, steam generation, or chemical reactions.
- Energy Use: The system can be optimized to meet both electricity and heating demands simultaneously, improving overall energy efficiency. The integration of electricity and heat generation reduces fuel consumption and improves operational efficiency.
Advantages of Cogeneration and CHP Systems
- High Efficiency: Cogeneration and CHP systems can achieve efficiencies of 60-80%, depending on the size and type of system. This is significantly higher than the typical efficiency of conventional power plants, which may only achieve 30-40% efficiency. By capturing and utilizing waste heat, CHP systems reduce the amount of fuel needed to generate electricity and heat, making them highly efficient.
- Cost Savings: By using the waste heat for useful purposes such as space heating or industrial processes, CHP systems can dramatically reduce energy costs. Facilities can reduce their reliance on external heating sources (such as boilers or electric heaters), which leads to lower fuel and energy bills.
- Environmental Benefits: CHP systems are more environmentally friendly than conventional systems because they use fuel more efficiently. The reduction in energy consumption also leads to a decrease in greenhouse gas emissions. For facilities using natural gas as a fuel, CHP systems can help reduce carbon emissions by as much as 40% compared to traditional electricity and heating methods.
- Flexibility: Cogeneration and CHP systems are flexible and can be used in a wide range of applications, from residential buildings to large industrial complexes. CHP plants can be scaled to meet the specific energy needs of the facility, making them suitable for both small and large operations.
- Grid Reliability: CHP systems can improve the reliability and stability of the grid. By producing electricity locally, CHP systems reduce the need for transmission and distribution from centralized power plants, decreasing the risks of power outages. In some cases, CHP systems can operate in “island mode,” providing energy even when the grid is down.
- Fuel Flexibility: Many CHP systems can run on a variety of fuels, including natural gas, biomass, coal, or even waste products like municipal solid waste (MSW). This provides operational flexibility and allows facilities to take advantage of lower-cost or more sustainable fuel sources.
- Improved Energy Security: By producing both electricity and heat on-site, CHP systems reduce dependence on external energy sources. This is particularly important in areas where energy supply is unreliable or expensive.
Applications of Cogeneration and CHP Systems
- Industrial Manufacturing: CHP systems are widely used in industrial manufacturing, where both electricity and thermal energy are required. Examples include chemical manufacturing, food processing, paper mills, and refineries. In these applications, CHP systems can capture waste heat from industrial processes and reuse it for heating or steam generation, significantly improving energy efficiency.
- District Heating and Cooling: In district energy systems, CHP plants generate both electricity and heat, which are then distributed to nearby buildings or communities. The heat is typically used for space heating, domestic hot water, or industrial processes. CHP systems are an effective solution for urban areas or large campuses where centralized heating and cooling are needed.
- Commercial Buildings: Smaller-scale CHP systems are used in commercial buildings, such as hotels, hospitals, and shopping centers, to provide both electricity and heating. In these applications, CHP systems can improve energy efficiency, reduce energy costs, and provide a reliable backup power source.
- Residential Buildings: In residential applications, micro-CHP systems are becoming increasingly popular. These small-scale systems can be used to provide heat and electricity to individual homes, helping homeowners reduce energy bills and improve energy efficiency.
- Waste-to-Energy Plants: Some CHP systems are integrated with waste-to-energy (WTE) plants, where the heat from the combustion of municipal solid waste (MSW) or biomass is used to generate both electricity and thermal energy. These systems help reduce landfill waste while providing valuable energy.
- Renewable Energy Integration: CHP systems can also be integrated with renewable energy sources such as biomass or biogas. These systems contribute to reducing carbon footprints and improving the sustainability of the energy supply.
Challenges of Cogeneration and CHP Systems
- High Initial Capital Costs: The initial investment required to install a cogeneration or CHP system can be significant. This includes the cost of the prime mover, heat recovery equipment, generators, and distribution systems. While these systems often offer substantial long-term savings, the upfront costs can be a barrier to adoption for some organizations.
- Space Requirements: Cogeneration and CHP systems, particularly larger installations, require significant space for installation. This can be a limitation in urban areas or facilities with limited available space.
- System Complexity: CHP systems are more complex than traditional heating or power systems due to the integration of multiple energy-generation and heat-recovery components. This can lead to higher maintenance requirements and operational challenges.
- Fuel Availability and Cost: The cost and availability of fuel can impact the overall performance and cost-effectiveness of a CHP system. Fluctuations in fuel prices or fuel supply can influence the economic viability of CHP operations.
- Regulatory and Environmental Considerations: Depending on the location, CHP systems may face regulatory hurdles, including emissions standards or permitting requirements. This can add complexity and cost to the installation and operation of these systems.
Conclusion
Cogeneration and combined heat and power (CHP) systems provide a highly efficient and sustainable solution for generating electricity and heat simultaneously. These systems are widely used in industrial, commercial, residential, and district energy applications, offering substantial energy savings, environmental benefits, and cost reductions. By improving fuel efficiency, reducing emissions, and providing a reliable source of energy, CHP systems play a vital role in advancing energy efficiency and sustainability goals. While they present challenges such as high capital costs and system complexity, the long-term economic and environmental benefits make them a compelling choice for many organizations looking to optimize their energy consumption.
Introduction to Waste-to-Energy Plants
Waste-to-energy (WTE) plants are facilities that use municipal solid waste (MSW) or other types of waste as a fuel source to generate electricity, heat, or both. These plants play a key role in waste management and energy production by diverting waste from landfills while producing valuable energy. The waste material is incinerated or processed to release heat, which is then converted into electricity or used for heating. WTE plants contribute to reducing the environmental impact of waste disposal and provide a renewable energy source.
By recovering energy from waste, these plants address two significant challenges: waste disposal and the need for cleaner, more sustainable energy sources. WTE plants are particularly useful in urban areas where waste generation is high, and landfill space is limited. They are also part of a circular economy, as they help manage waste while producing energy, often reducing dependence on fossil fuels and contributing to waste reduction efforts.
Components of Waste-to-Energy Plants
Waste-to-energy plants generally consist of several key components designed to efficiently convert waste into usable energy:
- Waste Reception and Handling System: The first step in the WTE process is the reception and preparation of waste. Waste is delivered to the plant where it is sorted and processed to remove non-combustible materials such as metals, glass, or hazardous waste. In some plants, pre-treatment processes like shredding or drying are used to reduce the volume and improve the quality of the waste.
- Incinerator or Combustion Chamber: The waste is then fed into an incinerator or combustion chamber, where it is burned at high temperatures. The incineration process releases heat energy, which is then captured and used to generate steam or hot gases. Modern WTE plants are equipped with advanced combustion systems designed to achieve efficient burning, minimizing the release of pollutants.
- Heat Recovery Steam Generator (HRSG): In most WTE plants, the heat produced from the burning of waste is captured in a Heat Recovery Steam Generator (HRSG), where water is converted into steam. This steam can either be used directly for industrial heating or to drive a steam turbine connected to a generator for electricity production.
- Electricity Generator: The steam produced in the HRSG is used to drive a steam turbine, which powers a generator to produce electricity. In some plants, combined-cycle systems are used, where both gas turbines and steam turbines are employed to maximize energy production.
- Flue Gas Treatment System: Incineration produces emissions that must be carefully managed to meet environmental standards. The flue gas treatment system filters and treats the exhaust gases to remove harmful pollutants, including particulate matter, sulfur dioxide (SO2), nitrogen oxides (NOx), and heavy metals. Technologies such as electrostatic precipitators, scrubbers, and selective catalytic reduction are often used in this stage.
- Ash Handling System: After the waste is burned, the remaining residue, or ash, is collected and processed. There are two types of ash produced: bottom ash (which remains at the bottom of the furnace) and fly ash (which is carried up with the flue gases). The ash is typically disposed of in landfills, but in some cases, it can be recycled or used in construction materials.
- Cooling System: Like most thermal power plants, WTE plants require a cooling system to condense steam back into water after it has passed through the turbine. This is typically done through cooling towers or air-cooled condensers.
Operational Process of Waste-to-Energy Plants
The operation of a WTE plant follows a specific sequence of steps that converts waste materials into electricity or heat:
- Waste Collection and Sorting: Waste is collected from municipal or industrial sources and delivered to the WTE plant. Upon arrival, the waste is sorted to remove recyclable or non-combustible materials that may pose a risk to the incineration process.
- Waste Incineration: The sorted waste is loaded into an incinerator, where it is burned at high temperatures. The combustion process breaks down the organic materials in the waste, releasing heat energy in the form of hot gases or steam.
- Heat Recovery and Power Generation: The heat from the incinerator is captured by the HRSG, which heats water to produce steam. The steam drives a turbine that is connected to a generator, producing electricity. In some systems, the steam may be directly used for heating applications, such as district heating or industrial processes.
- Flue Gas Treatment: The exhaust gases from the combustion process are directed through a flue gas treatment system, where pollutants are removed. This ensures that the emissions comply with environmental standards and reduces the plant’s impact on air quality.
- Ash Disposal and Management: The ash produced from the incineration process is removed and managed. Bottom ash is typically collected at the base of the furnace and may be used in construction or disposed of in landfills. Fly ash, which is captured in the flue gas treatment system, is carefully managed due to its potential toxicity and may require specialized disposal or treatment.
- Energy Distribution: The electricity generated by the plant is sent to the grid, while the heat can be used on-site for industrial purposes, district heating, or other applications. In some cases, thermal storage systems are used to store excess heat for later use.
Advantages of Waste-to-Energy Plants
- Waste Reduction: WTE plants help reduce the volume of waste that would otherwise be sent to landfills. Incineration can reduce the waste volume by up to 90%, significantly decreasing the need for landfill space and minimizing the environmental impacts of waste disposal.
- Renewable Energy Generation: WTE plants generate renewable energy from waste, which is considered a sustainable fuel source. The energy produced can be used to power homes, businesses, and industries, reducing reliance on fossil fuels.
- Reduction in Greenhouse Gas Emissions: By diverting waste from landfills, WTE plants help reduce methane emissions, which are a potent greenhouse gas. Additionally, WTE plants generally emit fewer greenhouse gases compared to landfills and can offset emissions from conventional power plants by providing a renewable energy source.
- Energy Efficiency: Modern WTE plants are highly efficient, with combined heat and power systems that can use both electricity and heat for various applications. The integration of energy recovery technologies maximizes the use of available resources.
- Energy Security: WTE plants contribute to energy security by providing a local and consistent source of energy. As waste is produced continuously, WTE plants offer a reliable energy generation option, unlike some renewable sources such as wind or solar, which depend on weather conditions.
- Environmental Management: WTE plants offer an effective means of managing municipal solid waste and other waste streams, reducing the negative impacts of uncontrolled waste disposal, such as pollution and contamination of soil and groundwater.
Challenges of Waste-to-Energy Plants
- High Capital Costs: Building a waste-to-energy plant requires a significant upfront investment, including the costs of constructing the plant, purchasing equipment, and meeting regulatory requirements. These costs can be a barrier, particularly in regions with limited financial resources.
- Emissions and Pollution Control: Although modern WTE plants are equipped with advanced emission control technologies, the incineration process still produces pollutants such as nitrogen oxides (NOx), sulfur dioxide (SO2), and particulate matter. These emissions must be carefully managed to meet environmental standards and prevent air quality degradation.
- Waste Quality and Consistency: The quality and composition of waste can vary widely, which can affect the efficiency of the incineration process. High moisture content, for example, can reduce combustion efficiency, while the presence of hazardous materials in the waste can pose environmental and operational challenges.
- Public Perception and Opposition: Waste-to-energy plants sometimes face public opposition due to concerns over air quality, environmental health, and the potential for increased traffic associated with waste collection. Community engagement and transparency are essential to address these concerns.
- Ash Disposal and Recycling: Although WTE plants reduce waste volume, the ash produced still needs to be managed. Fly ash, in particular, can contain hazardous substances and requires careful disposal. Efforts to recycle ash or use it in construction are ongoing, but challenges remain.
- Waste Availability: The availability and composition of waste can vary by region and may affect the economic viability of a WTE plant. In some areas, waste management practices such as recycling may reduce the amount of available waste for incineration, impacting the efficiency of the plant.
Applications of Waste-to-Energy Plants
- Municipal Solid Waste Management: WTE plants are particularly effective in urban areas where large amounts of waste are generated. They help municipalities manage waste more efficiently by diverting it from landfills and converting it into useful energy.
- District Heating: In cities with district heating systems, WTE plants can provide a significant portion of the heat required for residential and commercial buildings. The steam or hot water produced from the waste incineration process can be distributed through a network of pipes to provide heat for entire districts.
- Industrial Applications: WTE plants can supply energy to industrial processes that require heat, such as in cement production, paper manufacturing, or chemical industries. These applications benefit from both the electricity and heat generated by WTE plants.
- Electricity Generation: Waste-to-energy plants produce electricity that can be fed into the grid, helping to meet the energy demands of the surrounding area. In some cases, the plant may operate in a combined heat and power (CHP) mode, producing both electricity and useful thermal energy.
- Waste Diversion and Recycling: WTE plants contribute to the circular economy by diverting waste from landfills and reducing the environmental impacts associated with traditional waste disposal methods. In some cases, the ash produced from incineration can be recycled into construction materials.
Conclusion
Waste-to-energy plants provide a sustainable solution for waste disposal and energy generation. They offer significant benefits, including waste reduction, renewable energy production, and a reduction in greenhouse gas emissions. Despite challenges such as high capital costs and emissions control, WTE plants play an essential role in waste management and energy recovery systems. By converting waste into valuable resources, WTE plants contribute to a more sustainable and circular economy, helping cities and industries meet energy needs while addressing environmental concerns.
Introduction to Industrial Applications of Waste-to-Energy Plants
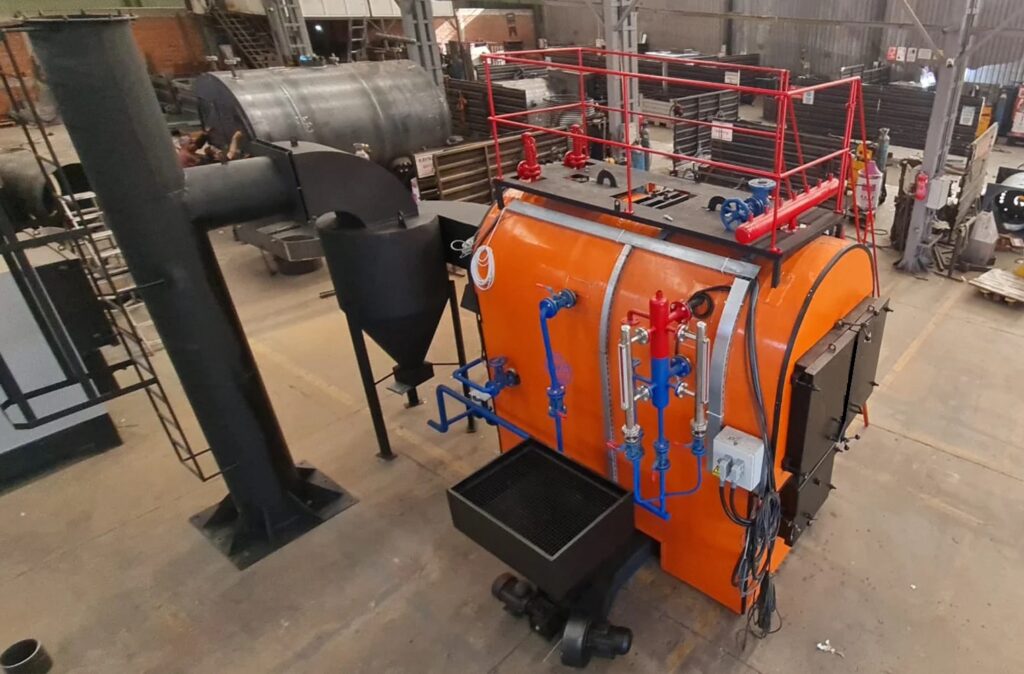
Industrial applications of Waste-to-Energy (WTE) plants involve the use of waste materials to generate both energy and heat for industrial processes. These plants serve as a sustainable solution for managing waste while providing valuable energy for manufacturing, production, and other industrial activities. The energy produced from waste incineration can be used to power machinery, provide heat for industrial processes, and generate electricity, making WTE plants an integral part of industrial energy systems. This approach helps reduce reliance on fossil fuels, lowers operating costs, and contributes to environmental sustainability by diverting waste from landfills.
Waste-to-energy technology is particularly advantageous in industries that require significant amounts of heat or electricity, such as cement production, paper manufacturing, and chemical processing. In these industries, WTE plants not only address waste disposal challenges but also improve energy efficiency, reduce carbon footprints, and enhance overall productivity by providing a reliable source of energy.
Components of Waste-to-Energy Plants for Industrial Applications
- Waste Collection and Preparation: In industrial settings, waste may come from various sources, such as manufacturing by-products, packaging materials, or organic waste. Before being incinerated, waste is typically sorted and processed to remove non-combustible items, hazardous materials, and recyclables. Pre-treatment such as shredding or drying can also be employed to optimize combustion efficiency.
- Incineration or Combustion System: The incineration system burns the waste at high temperatures to release thermal energy. For industrial applications, this combustion system is often designed to handle large volumes of waste while achieving high combustion efficiency. The system typically includes a furnace or combustion chamber where the waste undergoes controlled burning, producing heat in the form of hot gases or steam.
- Heat Recovery Steam Generator (HRSG): The heat produced during incineration is captured by a heat recovery steam generator (HRSG), which converts water into steam. In industrial applications, this steam can be used directly for process heating, to drive turbines, or to generate electricity. The HRSG maximizes the efficiency of the WTE plant by converting as much of the waste energy into useful thermal or electrical energy.
- Electricity Generation: The steam produced by the HRSG is used to drive a turbine connected to a generator. In many industrial WTE plants, this electricity is used on-site to power equipment, machinery, and other electrical systems. Some plants may also sell excess electricity back to the grid, providing an additional revenue stream.
- Flue Gas Treatment System: Industrial WTE plants are equipped with advanced flue gas treatment systems to reduce emissions of pollutants such as particulate matter, sulfur dioxide (SO2), nitrogen oxides (NOx), and heavy metals. These systems include technologies like electrostatic precipitators, scrubbers, and selective catalytic reduction to ensure that emissions comply with environmental standards.
- Ash Disposal and Recycling: After combustion, the remaining ash is collected and disposed of or recycled. Bottom ash can be repurposed for use in construction materials, while fly ash requires more careful handling due to its potential for containing hazardous substances.
- Cooling System: Cooling systems, typically through cooling towers or air-cooled condensers, are used to condense the steam back into water after it has passed through the turbine. This allows the plant to continue the cycle of energy production.
Operational Process of Waste-to-Energy Plants in Industrial Settings
- Waste Collection and Sorting: Industrial waste materials such as manufacturing scraps, packaging, or other waste are delivered to the WTE plant. The waste is sorted to eliminate materials that cannot be safely burned or those that are better suited for recycling.
- Incineration and Heat Recovery: The waste is fed into the combustion chamber, where it is incinerated at high temperatures, releasing energy in the form of heat. This heat is captured by the heat recovery steam generator, where water is heated to produce steam.
- Electricity Generation and Heat Utilization: The steam produced in the HRSG is used to drive a turbine connected to a generator, producing electricity that powers the plant and its industrial processes. The steam can also be used for direct industrial applications, such as in heating, drying, or chemical processes.
- Emissions Control: The flue gases from the incineration process are treated by pollution control equipment to minimize harmful emissions. This ensures that the WTE plant complies with local environmental regulations and contributes to reducing air pollution.
- Ash Handling and Disposal: After combustion, the remaining ash is collected and processed. Bottom ash is generally disposed of in landfills or used in construction, while fly ash, which may contain hazardous materials, requires special disposal or recycling procedures.
- Energy Distribution: The energy produced from the WTE plant is used to power industrial machinery and processes. The waste-to-energy system enhances operational efficiency by providing a reliable, local source of energy. In some cases, the plant may also contribute to district heating networks or sell excess electricity to the grid.
Advantages of Waste-to-Energy Plants in Industrial Applications
- Waste Reduction: WTE plants significantly reduce the amount of waste that would otherwise be sent to landfills. This waste reduction is particularly beneficial in industries that produce large volumes of by-products or packaging waste, such as food processing, manufacturing, and construction.
- Cost Savings: By generating electricity and heat from waste, industrial facilities can reduce their dependence on external energy sources, lowering operational costs. Waste-to-energy systems can also help businesses avoid the costs associated with waste disposal, such as landfill fees or recycling costs.
- Sustainable Energy Production: WTE plants convert waste materials into renewable energy, reducing the reliance on fossil fuels. This makes them an attractive option for industries looking to meet sustainability goals or reduce their carbon footprint.
- Energy Efficiency: Modern WTE plants are designed to be highly efficient, recovering a significant portion of the energy from waste. The integration of heat recovery systems and combined heat and power (CHP) technologies ensures that the maximum amount of energy is extracted from the waste stream.
- Environmental Benefits: By diverting waste from landfills and utilizing it as a fuel source, WTE plants contribute to reducing methane emissions, which are generated when waste decomposes in landfills. The efficient incineration process, when combined with advanced emissions control systems, ensures that the environmental impact of waste disposal is minimized.
- Reliability and Stability: Waste-to-energy systems provide a reliable and stable source of energy, which is crucial for industries that operate continuously or require a consistent supply of electricity and heat. This is particularly important in energy-intensive sectors such as cement manufacturing, chemicals, and paper mills.
Challenges of Waste-to-Energy Plants in Industrial Applications
- High Initial Capital Investment: The construction and installation of a WTE plant require a significant upfront investment, including the cost of equipment, infrastructure, and compliance with environmental regulations. While these systems can provide long-term cost savings, the initial capital costs can be a barrier for some industries.
- Waste Composition Variability: The quality and composition of waste can vary significantly, which can affect the efficiency of the incineration process. Waste with high moisture content or low calorific value may reduce combustion efficiency, making it harder to recover energy. In some cases, industries may need to pre-treat the waste to improve its combustion characteristics.
- Emissions and Pollution Control: Although WTE plants can reduce the environmental impact of waste disposal, they still produce emissions that need to be carefully managed. Flue gas treatment systems can be expensive to maintain, and the incineration process may generate pollutants such as nitrogen oxides, sulfur dioxide, and particulate matter, which require careful treatment to meet environmental standards.
- Ash Management: The disposal and recycling of ash produced during the incineration process can pose environmental challenges, particularly for fly ash, which may contain hazardous substances. Proper handling, storage, and disposal of ash are essential to prevent contamination of soil or water sources.
- Regulatory Compliance: WTE plants are subject to strict environmental regulations, which can vary by region. Compliance with emissions standards, waste handling protocols, and safety requirements can add complexity to the operation of WTE systems in industrial settings.
- Public Perception: Industrial WTE plants may face public opposition due to concerns about air quality, noise, and the potential for increased truck traffic associated with waste transportation. Engaging with local communities and ensuring transparency in operations can help mitigate these concerns.
Applications of Waste-to-Energy Plants in Industry
- Cement Production: Cement manufacturing is an energy-intensive process that requires a substantial amount of heat. WTE plants can provide a reliable source of energy, reducing the reliance on fossil fuels like coal and helping cement plants meet sustainability goals. The heat generated from waste can be used to fuel rotary kilns, which are essential in cement production.
- Paper and Pulp Industry: The paper industry generates significant amounts of organic waste, such as wood chips, pulping residues, and paper scraps. WTE plants can convert this waste into energy, providing heat and electricity for the manufacturing process. The heat can be used for drying paper or for other thermal processes in the paper mill.
- Food and Beverage Manufacturing: Food processing generates large amounts of organic waste, including food scraps, packaging materials, and by-products. WTE systems can help food manufacturers manage waste while producing energy for production processes. The steam generated from waste incineration can be used for heating or sterilization in food processing.
- Chemical Manufacturing: Chemical plants often require large quantities of heat and electricity to run various processes, including distillation, refining, and chemical reactions. Waste-to-energy systems can provide the necessary heat and electricity, improving energy efficiency and reducing operating costs. Waste streams from the manufacturing process can also be used as a fuel source for incineration.
- Textile Industry: The textile industry generates waste in the form of fabric scraps, dye residues, and chemical by-products. These materials can be processed in WTE plants to produce energy that powers textile production. The energy can be used for heating, dyeing, and other textile manufacturing processes.
Conclusion
Waste-to-energy plants offer significant benefits for industrial applications, providing a sustainable solution for waste disposal while generating valuable energy. These systems contribute to waste reduction, cost savings, energy efficiency, and environmental sustainability. By converting industrial waste into energy, WTE plants help industries reduce their carbon footprint, lower energy costs, and minimize their impact on landfills. However, challenges such as high initial capital investment, emissions control, and waste composition variability must be carefully managed. Despite these challenges, waste-to-energy technology is increasingly being adopted in industries seeking to enhance energy security, improve waste management, and meet sustainability objectives.
Introduction to Geothermal and Biomass Energy Systems
Geothermal and biomass energy systems are renewable energy solutions that harness natural resources to produce electricity and heat. Both systems play a crucial role in reducing dependence on fossil fuels, mitigating environmental impacts, and promoting sustainability. Geothermal energy taps into the Earth’s internal heat, while biomass energy uses organic materials as fuel. Together, they represent clean, reliable, and renewable sources of energy that are suitable for various applications, ranging from residential and industrial heating to large-scale power generation. These systems provide a sustainable and environmentally friendly alternative to conventional energy sources.
Geothermal Energy Systems
Geothermal energy involves harnessing the heat stored beneath the Earth’s surface to generate electricity or provide heating. This energy is primarily found in the form of hot water or steam reservoirs located deep within the Earth. Geothermal energy is a highly reliable source of energy because it is available 24/7, unlike other renewable sources such as wind and solar, which are intermittent.
Geothermal energy systems can be categorized into three main types: dry steam, flash steam, and binary cycle plants.
- Dry Steam Plants: These plants directly use steam from geothermal reservoirs to drive turbines connected to electricity generators. This type of plant is most commonly used in areas with high-temperature geothermal resources.
- Flash Steam Plants: Flash steam plants use geothermal water from deep underground, which is under high pressure. When the water reaches the surface, the pressure is reduced (or “flashed”), causing the water to turn into steam. The steam is then used to drive turbines for electricity generation.
- Binary Cycle Plants: Binary cycle plants transfer heat from geothermal hot water to a secondary fluid with a lower boiling point, which then vaporizes and drives a turbine. These systems are typically used in areas with lower temperature geothermal resources.
Key Components of Geothermal Energy Systems
- Geothermal Wells: These wells are drilled into geothermal reservoirs to extract hot water or steam. The wells are often deep, sometimes reaching several kilometers underground, where the temperature and pressure are sufficient to produce geothermal energy.
- Heat Exchanger: In binary cycle plants, heat exchangers are used to transfer heat from geothermal fluids to a secondary working fluid, which is vaporized and used to drive turbines. This allows the system to operate efficiently, even in areas with lower geothermal temperatures.
- Turbine and Generator: The steam or vaporized secondary fluid from the geothermal resource drives turbines connected to generators, producing electricity. In some systems, steam is directly used to power turbines, while in others, a secondary fluid is involved in the process.
- Cooling System: Geothermal plants require cooling systems to condense steam after it has passed through the turbines. This process is essential for maintaining the efficiency of the system and reintroducing the water back into the reservoir.
- Reinjection Wells: After the geothermal fluid has been used to generate power, it is often reinjected back into the geothermal reservoir to maintain the sustainability of the resource. This helps to prevent depletion of the geothermal reservoir and ensures the continued availability of energy.
Advantages of Geothermal Energy Systems
- Sustainability: Geothermal energy is highly sustainable because the Earth’s internal heat is vast and virtually inexhaustible over human timescales. By reinjecting used fluids back into the reservoirs, geothermal power plants can operate indefinitely with minimal environmental impact.
- Reliability: Unlike solar and wind energy, geothermal energy is not dependent on weather conditions. It provides a stable, continuous supply of energy, making it a reliable source for base-load power generation.
- Low Carbon Footprint: Geothermal energy systems have a low environmental impact compared to fossil fuel-based power generation. They produce little to no greenhouse gas emissions, making them an excellent choice for reducing carbon footprints.
- Efficiency: Geothermal plants have high capacity factors and are highly efficient in generating electricity or providing heating. They can operate continuously, producing energy at a consistent level throughout the year.
- Small Land Footprint: Geothermal power plants typically have a smaller land footprint compared to other renewable energy technologies, such as solar farms or wind turbines, making them a suitable option for areas with limited space.
Challenges of Geothermal Energy Systems
- Geographical Limitations: Geothermal energy is location-specific, with viable resources only available in certain regions, typically near tectonic plate boundaries or volcanic activity zones. This limits its widespread application.
- High Initial Costs: The upfront costs of drilling geothermal wells and establishing infrastructure can be high. While the long-term operational costs are low, the initial investment required can be a barrier for some developers.
- Resource Depletion: In some cases, geothermal reservoirs may become depleted if not managed properly, which is why reinjection and careful management of geothermal resources are critical to ensuring sustainability.
- Environmental Impact: While geothermal energy has a low environmental footprint, drilling and reservoir management can still produce localized environmental impacts, including land use changes, noise, and potential for minor seismic activity.
Biomass Energy Systems
Biomass energy systems convert organic materials such as plant and animal waste into heat, electricity, or biofuels. Biomass can include wood, agricultural residues, waste from forestry, food processing, and even municipal solid waste. Biomass energy is considered carbon-neutral because the carbon dioxide released during combustion is roughly equivalent to the amount absorbed by plants during their growth, offsetting the emissions produced.
Biomass energy can be used for various applications, including heating, electricity generation, and transportation fuels. The conversion of biomass into energy can take several forms, including direct combustion, gasification, and anaerobic digestion.
- Direct Combustion: This is the most common method of converting biomass into heat and power. Biomass is burned in a furnace or boiler to generate heat, which is used to produce steam that drives a turbine connected to a generator for electricity production.
- Gasification: In gasification, biomass is heated in a low-oxygen environment to produce a gas known as syngas, which can be used to generate electricity or converted into biofuels.
- Anaerobic Digestion: Organic waste is broken down by bacteria in the absence of oxygen to produce biogas, primarily composed of methane. This biogas can be used to generate electricity or heat.
Key Components of Biomass Energy Systems
- Biomass Feedstock: The primary input for biomass energy systems is the organic material or feedstock, which can include wood chips, agricultural residues, food waste, or algae. The quality and type of biomass feedstock can impact the efficiency of the system.
- Boilers and Furnaces: Biomass is typically burned in specialized boilers or furnaces to generate heat. The heat is used to produce steam, which drives turbines for electricity generation or is used for industrial heating applications.
- Gasifiers: In gasification systems, biomass is heated in a controlled environment to produce syngas. The syngas can then be used to power gas turbines or internal combustion engines, providing electricity.
- Biogas Digesters: Anaerobic digesters break down organic materials to produce biogas. The digesters may be used to treat agricultural waste, sewage sludge, or food waste, turning it into valuable energy.
- Combined Heat and Power (CHP) Systems: Biomass energy systems are often integrated with CHP technology, which allows for both electricity generation and the use of waste heat for industrial processes or district heating.
Advantages of Biomass Energy Systems
- Renewable Resource: Biomass is considered a renewable energy source because it can be replenished through the growth of new plants. This makes it a sustainable alternative to fossil fuels, especially in regions with abundant agricultural or forestry waste.
- Waste Reduction: Biomass energy systems help reduce waste by converting organic materials into useful energy. This helps divert waste from landfills and minimizes environmental pollution.
- Carbon Neutrality: Biomass energy is considered carbon-neutral because the carbon dioxide released during combustion is offset by the carbon absorbed by plants during their growth, contributing to a net-zero carbon footprint.
- Energy Security: Biomass energy systems contribute to energy security by utilizing locally available resources. This reduces dependence on imported fuels and enhances the resilience of the energy system.
- Job Creation: Biomass energy production can create jobs in the agricultural, forestry, and waste management sectors, promoting local economic development.
Challenges of Biomass Energy Systems
- Resource Availability: The availability of biomass feedstock can be inconsistent, depending on seasonal and regional factors. This can lead to supply chain challenges and fluctuations in energy production.
- Land Use and Environmental Concerns: Large-scale biomass production may require significant land use for growing crops or harvesting timber. This can lead to competition with food production or biodiversity concerns, especially if not managed sustainably.
- High Operational Costs: While biomass systems can be cost-effective in the long term, the initial investment and ongoing operational costs, such as feedstock collection and transportation, can be high.
- Air Pollution: Biomass combustion can produce emissions, including particulate matter and volatile organic compounds, which require careful management to meet environmental standards.
Conclusion
Geothermal and biomass energy systems are two powerful renewable energy sources that help reduce dependence on fossil fuels, promote sustainability, and mitigate environmental impacts. Geothermal energy offers a reliable, continuous energy source, while biomass provides flexibility in feedstock and a way to utilize waste products. Both systems have distinct advantages and challenges but contribute significantly to the global transition to clean and renewable energy. By leveraging these technologies, industries and communities can reduce carbon emissions, enhance energy security, and support sustainable economic growth.
Introduction to HVAC Systems
HVating, Ventilation, and Air Conditioning (HVAC) systems are essential for maintaining a comfortable indoor environment in residential, commercial, and industrial buildings. These systems are designed to regulate temperature, humidity, air quality, and air circulation, ensuring optimal conditions for both occupants and equipment. HVAC systems can be found in homes, office buildings, shopping malls, factories, hospitals, and many other settings where indoor climate control is needed. The primary goal of an HVAC system is to create a comfortable, healthy, and energy-efficient environment, regardless of external weather conditions.
HVAC systems play a crucial role in improving indoor air quality (IAQ) and controlling the climate within buildings. By regulating temperature, airflow, and humidity levels, they help prevent discomfort, health issues, and the deterioration of materials and equipment. With advances in energy efficiency and automation, modern HVAC systems are increasingly sophisticated, offering a wide range of features for improved performance, energy savings, and user control.
Components of HVAC Systems
- Heating Equipment: The heating component of an HVAC system is responsible for maintaining indoor temperatures during colder weather. It can include furnaces, boilers, heat pumps, and electric heaters. The most common form of heating in residential systems is through forced air using a furnace, which heats air and distributes it through ducts. In larger commercial settings, boilers or heat exchangers might be used to provide heat, circulating hot water or steam to maintain a comfortable environment.
- Ventilation Equipment: Ventilation refers to the process of exchanging indoor air with fresh outdoor air and ensuring proper circulation of air inside a building. This component is critical for maintaining healthy indoor air quality (IAQ) by removing pollutants, moisture, and odors. Ventilation systems can include mechanical fans, air ducts, air exchangers, and exhaust systems. Proper ventilation helps to prevent the buildup of harmful gases and pollutants, such as carbon dioxide and volatile organic compounds (VOCs), which can negatively impact health and comfort.
- Air Conditioning Equipment: Air conditioning is designed to cool the indoor environment and control humidity levels, providing comfort in hot weather. The most common form of air conditioning in residential and commercial applications is through refrigerant-based cooling systems. These systems use a compressor, condenser, evaporator, and expansion valve to absorb and remove heat from the indoor air. In industrial or large commercial settings, chillers or cooling towers may be used to provide large-scale cooling for building spaces or processes.
- Control Systems: Modern HVAC systems often incorporate advanced control systems to optimize performance, comfort, and energy efficiency. These systems include thermostats, sensors, programmable controllers, and building automation systems (BAS). Thermostats regulate temperature by controlling heating and cooling equipment, while sensors monitor conditions like humidity, air quality, and occupancy levels to adjust the system’s operations accordingly. Building automation systems integrate all HVAC components to ensure optimal functioning, energy use, and comfort.
- Ductwork and Piping: Ducts are used to distribute conditioned air (heated or cooled) throughout the building. These air ducts are often made of metal or flexible materials and are connected to registers or vents in various rooms. In addition to ducts, HVAC systems may also use pipes to distribute hot water or steam for heating, as well as refrigerant pipes for cooling.
- Air Filters: Filters are critical components in HVAC systems, used to trap dust, allergens, and other particles from the air before it is circulated through the system. Clean air filters help to maintain air quality, reduce the accumulation of dust in the system, and improve the overall efficiency of the HVAC system. Regular filter replacement is essential to prevent system overloading and ensure good air quality.
Types of HVAC Systems
- Central HVAC Systems: Centralized HVAC systems are commonly used in larger buildings, such as commercial buildings, hospitals, and industrial plants. These systems typically include a central furnace or air handler that heats or cools air before it is distributed throughout the building via ducts or pipes. Central systems can be either heating-only, cooling-only, or combined heating and cooling units. These systems often have greater capacity and can handle larger areas more efficiently.
- Ductless HVAC Systems: Ductless systems, also known as mini-split systems, are designed for use in smaller buildings or rooms that do not have the space for extensive ductwork. These systems consist of an outdoor unit and one or more indoor air-handling units connected by refrigerant lines. Ductless HVAC systems provide individualized control of temperature for different areas of the building and are often used in retrofits or in buildings with limited space.
- Hybrid HVAC Systems: Hybrid systems combine both electric and gas heating technologies, allowing the system to switch between energy sources based on efficiency and demand. This can be especially useful in areas with fluctuating energy prices or extreme weather conditions. Hybrid systems are designed to optimize energy use by automatically choosing the most efficient mode of operation.
- Geothermal HVAC Systems: Geothermal heating and cooling systems use the stable temperature of the earth to heat and cool a building. These systems typically include a heat pump connected to a series of underground loops filled with water or refrigerant. Geothermal systems are highly energy-efficient because they rely on the earth’s natural heat rather than on combustion-based or electrical heating.
- Variable Refrigerant Flow (VRF) Systems: VRF systems are a type of HVAC technology that allows for multiple indoor units to be connected to a single outdoor unit, providing flexibility and energy efficiency. These systems can provide both heating and cooling simultaneously to different parts of a building, making them ideal for large commercial spaces. The key feature of VRF systems is their ability to vary the flow of refrigerant to each indoor unit, optimizing energy use and comfort.
HVAC System Design Considerations
Designing an effective HVAC system involves several key considerations that ensure the system is both efficient and appropriate for the building’s needs:
- Building Size and Layout: The size, layout, and purpose of the building play a significant role in determining the type and capacity of the HVAC system. For example, a multi-story office building requires a different system compared to a small single-story residential home.
- Energy Efficiency: Energy efficiency is a critical aspect of HVAC design. Modern HVAC systems are designed to meet energy standards and minimize energy use while maintaining comfort. Proper insulation, high-efficiency components, and smart control systems help to reduce energy consumption and costs.
- Indoor Air Quality: Ensuring high indoor air quality is a priority for HVAC systems. The design should include appropriate filtration and ventilation systems to remove pollutants and provide a constant supply of fresh air. Controlling humidity and temperature also helps prevent mold growth and ensure a comfortable living or working environment.
- Climate and Environment: The geographic location and climate conditions of a building’s location determine the specific needs for heating, cooling, and ventilation. HVAC systems in areas with extreme temperatures must be capable of handling higher heating or cooling loads, whereas systems in temperate regions may have more moderate requirements.
- System Zoning: Zoning involves dividing the building into different sections that can be controlled independently. This allows for more precise control of temperature and airflow, improving comfort and energy efficiency. Zoning systems are often used in larger homes or commercial buildings to provide customized heating and cooling for different areas.
Maintenance of HVAC Systems
Proper maintenance is essential for ensuring the long-term performance, efficiency, and reliability of HVAC systems. Regular servicing can help prevent breakdowns, improve energy efficiency, and extend the lifespan of the equipment. Key maintenance tasks include:
- Filter Replacement: Replacing air filters regularly is crucial for maintaining air quality and preventing system clogging. Dirty filters can reduce airflow and increase energy consumption, leading to inefficient operation.
- Cleaning Coils and Ducts: Over time, dust and debris can accumulate on the coils and inside ducts, reducing the system’s ability to operate efficiently. Cleaning these components ensures optimal performance and reduces the risk of system failure.
- Inspecting Refrigerant Levels: Low refrigerant levels can lead to inefficient cooling and potential damage to the system. Regular checks and recharges of refrigerant help maintain the cooling capacity of the air conditioning components.
- Checking Insulation: Insulation in the ducts, piping, and components of the HVAC system helps maintain temperature control and prevent energy loss. Regular inspection ensures that the insulation is intact and functioning properly.
- Lubricating Moving Parts: Parts like motors, fans, and bearings require lubrication to reduce wear and tear. Regular lubrication helps prevent mechanical failures and extends the life of these components.
Advantages of HVAC Systems
- Comfort: HVAC systems ensure a consistent and comfortable indoor environment by regulating temperature, humidity, and air quality. They provide relief from extreme weather conditions, whether it’s heating during the winter or cooling during the summer.
- Improved Indoor Air Quality: Ventilation and filtration components of HVAC systems improve indoor air quality by removing pollutants, allergens, and moisture. This contributes to healthier indoor environments, which is especially important in commercial buildings, healthcare facilities, and schools.
- Energy Efficiency: Modern HVAC systems are designed to optimize energy use, reducing operational costs. Advanced control systems, variable-speed fans, and energy-efficient compressors all contribute to minimizing energy consumption.
- Control and Automation: Smart thermostats and building automation systems allow users to control and monitor HVAC systems remotely, providing convenience and further energy savings. Automated controls adjust temperature settings based on occupancy or time of day, improving overall efficiency.
- Long-Term Savings: Although the initial cost of installing an HVAC system can be high, proper installation and maintenance lead to long-term energy savings. Energy-efficient systems reduce utility bills, and well-maintained equipment lasts longer, reducing the need for costly repairs or replacements.
Conclusion
HVAC systems are vital for maintaining comfortable and healthy indoor environments across various settings. They regulate temperature, humidity, and air quality, ensuring that buildings are comfortable and efficient. With advances in energy-efficient technologies, automation, and control systems, modern HVAC solutions are designed to meet the evolving needs of residential, commercial, and industrial applications. Proper installation, design, and maintenance of HVAC systems are essential for optimizing their performance, extending their lifespan, and minimizing energy consumption, making them a key component of sustainable building practices.
Introduction to Exhaust Gas Heat Exchangers
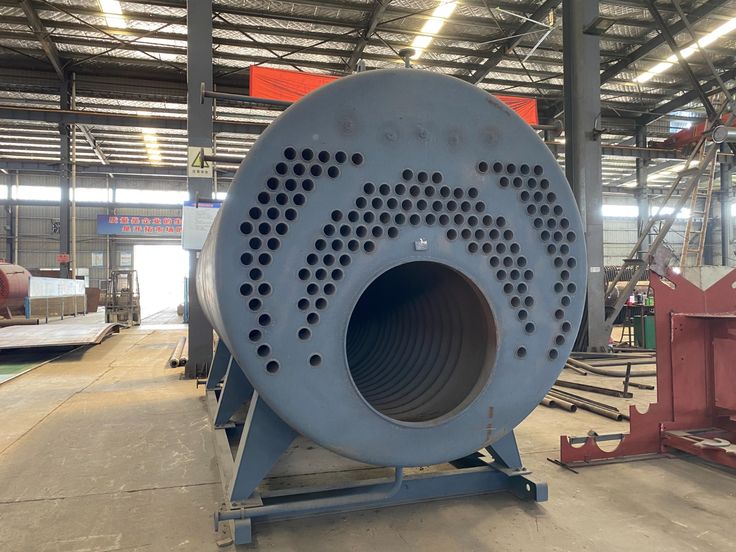
Exhaust gas heat exchangers (EGHEs) are critical components in various industrial and energy systems, designed to capture and recover heat from exhaust gases that would otherwise be wasted. These systems are primarily used in power plants, refineries, petrochemical facilities, and other energy-intensive industries where large amounts of hot gases are produced as byproducts of combustion or chemical processes. By recovering heat from these exhaust gases, EGHEs improve energy efficiency, reduce fuel consumption, and lower emissions, contributing to cost savings and environmental sustainability.
Exhaust gas heat exchangers are part of a broader strategy known as heat recovery, which is focused on reclaiming wasted thermal energy for reuse. The recovered heat can be used to preheat incoming air, water, or other fluids, lowering the demand for primary heating systems and improving overall system efficiency. This principle of energy recovery is fundamental to enhancing the sustainability and operational performance of various industrial processes, especially in energy generation.
Working Principle of Exhaust Gas Heat Exchangers
Exhaust gas heat exchangers operate on the principle of thermal transfer, where heat is transferred from the hot exhaust gases to a secondary fluid, such as water, air, or oil. The heat exchanger is designed to maximize the contact surface area between the exhaust gas and the working fluid, promoting efficient heat transfer. Typically, the exhaust gases flow through one set of channels, while the secondary fluid flows through separate channels, often in a counter-flow arrangement to maximize the temperature difference and improve heat transfer efficiency.
The exhaust gases typically pass through tubes or ducts, while the heat recovery medium (water, air, or another fluid) flows through surrounding passages or the opposite set of tubes. The heat exchange process occurs via conduction, convection, or both, depending on the design and materials used in the heat exchanger. The secondary fluid absorbs the heat from the exhaust gases, which lowers the temperature of the gases before they are released into the atmosphere.
Types of Exhaust Gas Heat Exchangers
Exhaust gas heat exchangers come in several types, depending on the design, application, and specific needs of the system. The most common types include:
- Shell and Tube Heat Exchangers: Shell and tube heat exchangers are commonly used in exhaust gas heat recovery applications due to their robust construction and high heat transfer capacity. In this design, hot exhaust gases flow through a set of tubes, while the secondary fluid circulates around the outside of the tubes in a shell. This configuration provides a large surface area for heat exchange and is highly effective in high-temperature environments.
- Plate Heat Exchangers: Plate heat exchangers are made up of multiple thin plates that create channels for the exhaust gases and the heat recovery fluid to flow through. The plates are stacked together, allowing for efficient heat transfer between the fluids. Plate heat exchangers are compact, lightweight, and can offer high thermal performance in smaller spaces compared to shell and tube heat exchangers.
- Air-to-Air Heat Exchangers: In applications where the exhaust gases are used to preheat incoming air (for example, in air handling systems), air-to-air heat exchangers are commonly employed. These heat exchangers utilize two separate air streams—one for the exhaust gases and the other for the incoming air—to transfer heat. They are particularly useful in applications like industrial ventilation systems, where heat recovery can help reduce heating costs.
- Regenerative Heat Exchangers: Regenerative heat exchangers are designed to temporarily store heat from the exhaust gases before transferring it to the incoming fluid. These systems use a heat-absorbing medium, such as ceramic or metal, to capture the heat from the exhaust gases. The heat is then transferred to the incoming air or water during the next cycle. This type of heat exchanger is typically used in systems with intermittent exhaust gas flow, such as those found in large engines or turbines.
- Plate Fin Heat Exchangers: In some applications, exhaust gas heat exchangers use plate fin designs, which consist of alternating plates and fins to increase the surface area for heat exchange. These designs are typically used in systems where compactness and high heat transfer efficiency are important, such as in automotive or aerospace applications.
Applications of Exhaust Gas Heat Exchangers
Exhaust gas heat exchangers are employed in a wide range of industries and applications, including:
- Power Generation: In power plants, EGHEs recover waste heat from the exhaust gases of turbines, boilers, or engines. This recovered heat can be used to preheat air, water, or steam for the power generation process, improving the overall efficiency of the plant. Combined-cycle power plants, which use both gas and steam turbines, benefit greatly from exhaust gas heat recovery.
- Industrial Manufacturing: Many industrial manufacturing processes generate significant amounts of exhaust gases, including cement production, metal processing, and chemical manufacturing. Exhaust gas heat exchangers help recover heat from these exhaust gases, reducing the need for additional energy inputs and improving process efficiency.
- Refining and Petrochemical Plants: In refineries and petrochemical facilities, where large amounts of heat are produced from the combustion of hydrocarbons, exhaust gas heat exchangers play a crucial role in reducing energy costs. By recovering heat from furnace or stack gases, these facilities can reuse the thermal energy for preheating feedstock or other processes.
- Marine Industry: In marine applications, such as in ships or offshore platforms, exhaust gas heat exchangers are used to recover heat from engine exhaust gases to support auxiliary systems, like fresh water production (via desalination) or heating systems. This reduces fuel consumption and increases the overall energy efficiency of the vessel.
- Automotive and Aerospace: In automotive and aerospace applications, exhaust gas heat exchangers are used to enhance the efficiency of engines and turbines by recovering waste heat. These systems can help reduce fuel consumption and improve the performance of propulsion systems.
- HVAC Systems: In large commercial and industrial HVAC systems, exhaust gas heat exchangers are used to recover waste heat from the air and utilize it to preheat incoming air, reducing the load on heating systems and saving energy. These systems can improve overall building energy efficiency and reduce heating costs.
- Waste-to-Energy Plants: In waste-to-energy facilities, where municipal solid waste is burned to generate electricity or heat, EGHEs are used to recover heat from the flue gases. This heat can be used to produce steam for power generation or district heating systems, making the overall process more energy-efficient.
Advantages of Exhaust Gas Heat Exchangers
- Energy Efficiency: One of the primary benefits of exhaust gas heat exchangers is their ability to recover waste heat and improve energy efficiency. By capturing and reusing heat that would otherwise be lost, these systems reduce the need for additional fuel and energy consumption, leading to cost savings and improved operational efficiency.
- Reduced Environmental Impact: By recovering and reusing exhaust heat, EGHEs help reduce the carbon footprint of industrial and power generation systems. Lower fuel consumption and emissions result in less environmental pollution and contribute to sustainability goals.
- Cost Savings: Heat recovery through exhaust gas heat exchangers can lead to significant cost savings over time. The recovery of waste heat reduces the need for external fuel sources and lowers operational costs associated with heating and energy consumption.
- Improved System Performance: EGHEs help optimize the performance of industrial processes and power generation systems. By preheating air, water, or other fluids, they ensure that systems operate at optimal temperatures, improving efficiency and reducing wear and tear on equipment.
- Flexibility: Exhaust gas heat exchangers are versatile and can be applied in a wide range of industries and applications. They can be tailored to meet specific system requirements, such as temperature ranges, flow rates, and heat recovery needs, making them adaptable to various setups.
Challenges and Considerations
- Corrosion and Fouling: Exhaust gases often contain corrosive substances, such as sulfur compounds or particulate matter, which can damage the heat exchanger over time. Regular maintenance and cleaning are necessary to prevent fouling and corrosion, which can reduce the heat exchanger’s efficiency and lifespan.
- High Initial Costs: The installation of exhaust gas heat exchangers can involve high initial costs, particularly for large-scale or custom-built systems. However, the long-term energy savings and operational benefits often outweigh these initial investments.
- Space Requirements: Depending on the type and size of the system, exhaust gas heat exchangers may require significant space for installation. This can be a challenge in environments with limited space or complex layouts, such as in ships or small industrial facilities.
- Temperature Limitations: Exhaust gas heat exchangers are designed to operate within certain temperature ranges. If the exhaust gases are too hot, the heat exchanger may need to be specially designed to handle the extreme temperatures, which can increase costs and complicate installation.
Conclusion
Exhaust gas heat exchangers play a vital role in improving energy efficiency, reducing fuel consumption, and minimizing environmental impact in various industrial and power generation applications. By capturing waste heat from exhaust gases and transferring it to a secondary fluid, these systems contribute to cost savings, enhanced performance, and sustainability. Despite some challenges related to maintenance, initial costs, and space requirements, exhaust gas heat exchangers are an essential technology for industries seeking to optimize energy use and reduce their carbon footprint. As energy efficiency becomes increasingly important in industrial operations, the role of EGHEs in heat recovery and waste reduction will continue to grow.
Working Principle of Exhaust Gas Heat Exchangers
The working principle of exhaust gas heat exchangers is based on the efficient transfer of thermal energy from hot exhaust gases to a secondary fluid, such as air, water, or oil, which can then be reused in the system for various purposes. Exhaust gases, typically produced by combustion processes in industries, power plants, engines, or turbines, contain significant amounts of thermal energy. Without recovery, this heat is often wasted as the exhaust is vented into the atmosphere at high temperatures. Exhaust gas heat exchangers are designed to capture and transfer this waste heat, allowing for its reuse in preheating incoming fluids, improving the overall energy efficiency of the system, and reducing fuel consumption.
The process begins with the flow of exhaust gases through one side of the heat exchanger. These gases, often at high temperatures, pass through a set of channels, tubes, or passages within the heat exchanger. On the other side, a secondary fluid, which is typically cooler than the exhaust gases, flows through a separate set of channels. The key feature of this design is the physical separation between the exhaust gases and the secondary fluid, preventing direct mixing while allowing heat to transfer between the two streams.
Heat is transferred primarily through conduction, where the thermal energy from the hot exhaust gases flows through the walls of the tubes or the material of the heat exchanger, to the cooler secondary fluid. In some designs, heat transfer can also occur via convection, where the movement of the fluids enhances the heat transfer process. The heat exchange process is most efficient when the temperature difference between the exhaust gases and the secondary fluid is maximized, which is why many heat exchangers are designed with counterflow or crossflow configurations. In a counterflow setup, the exhaust gases and the secondary fluid flow in opposite directions, which creates a higher temperature gradient and thus improves heat transfer efficiency.
The recovered heat can then be used for various purposes, such as preheating air, water, or combustion air, depending on the needs of the system. For example, in power plants or industrial settings, the heat from exhaust gases can be used to preheat the air entering a furnace, reducing the amount of energy needed for the combustion process. This recovery of waste heat not only enhances the overall efficiency of the system but also reduces the environmental impact by lowering the demand for additional fuel and minimizing harmful emissions.
The design of the exhaust gas heat exchanger varies depending on factors such as the type of exhaust gas, the required heat recovery, the temperature range, and the specific application. The exchanger material is chosen to withstand the high temperatures of the exhaust gases and to resist corrosion, as exhaust gases often contain harmful components like sulfur, nitrogen oxides, and particulates that can degrade the system over time. Regular maintenance is crucial for ensuring optimal performance, as buildup of particulate matter or corrosive substances can impair heat transfer efficiency.
Overall, exhaust gas heat exchangers operate by capturing and transferring the thermal energy from hot exhaust gases to a secondary fluid, thereby recovering waste heat, improving energy efficiency, and reducing the need for additional fuel consumption. This principle of heat recovery is an essential technology in various industries, including power generation, manufacturing, and marine operations, contributing to both cost savings and environmental sustainability.
Shell and Tube Heat Exchangers
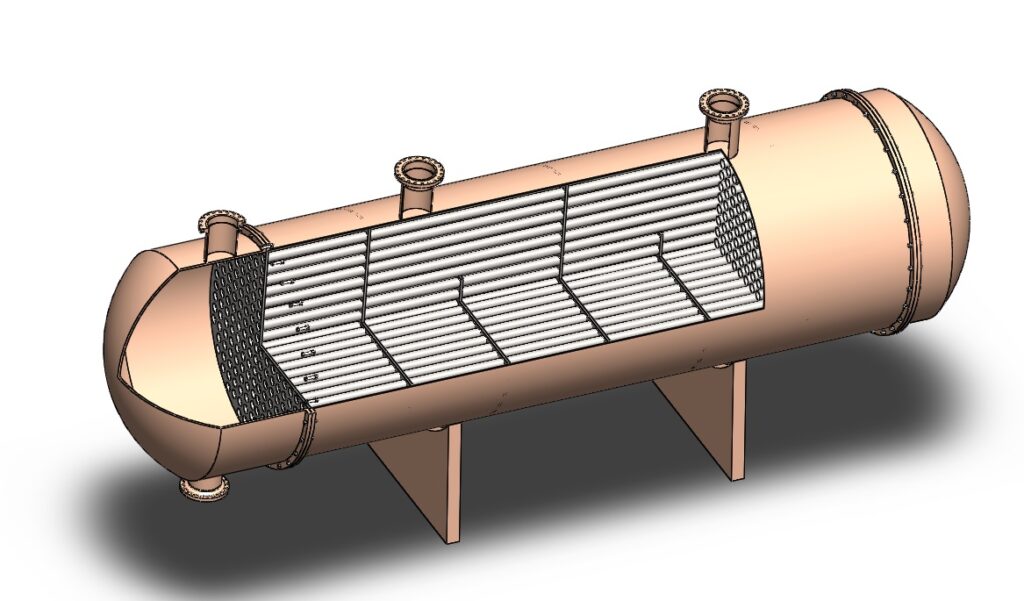
Shell and tube heat exchangers are one of the most widely used types of heat exchangers in industrial applications due to their robust design and efficient heat transfer capabilities. The basic design consists of a series of tubes housed within a cylindrical shell, with one fluid flowing inside the tubes and the other fluid circulating around the tubes within the shell. This arrangement allows for effective heat exchange between the two fluids as heat is transferred from the hotter fluid inside the tubes to the cooler fluid outside the tubes. Shell and tube heat exchangers are particularly suitable for high-pressure and high-temperature applications, and they can handle large flow rates of fluids, making them ideal for industries like power generation, petrochemical processing, HVAC, and many others.
The working principle of shell and tube heat exchangers revolves around the thermal conduction and convection processes. As the hot fluid flows through the tubes, it releases heat to the surrounding cooler fluid, which flows in the shell space around the tubes. The heat transfer occurs through the tube walls, with the temperature of the hot fluid decreasing as it moves along the length of the tubes, while the temperature of the cooler fluid increases as it absorbs the heat. To enhance heat transfer efficiency, the design of the heat exchanger may incorporate features such as baffles, which help to direct the fluid flow and improve mixing, as well as increase the turbulence around the tubes, further promoting heat transfer.
One of the key advantages of shell and tube heat exchangers is their versatility. They can be designed to handle a wide range of temperature and pressure conditions and can be configured for both liquid-to-liquid and gas-to-liquid heat exchange. This versatility makes them suitable for a variety of applications, from cooling systems in power plants to heating and cooling processes in the chemical industry. The design can be customized to meet specific requirements, such as the number of tube passes, the materials used for construction, the arrangement of the baffles, and the type of fluids involved. This flexibility allows for optimal performance in different operating conditions and with different fluids.
Shell and tube heat exchangers also offer significant durability and reliability. The tube side of the heat exchanger is typically designed to withstand high-pressure fluids, while the shell side can be used to handle larger flow rates. The materials used for the construction of the tubes and the shell are chosen to ensure resistance to corrosion, erosion, and other environmental factors that may degrade the system over time. Common materials include stainless steel, carbon steel, and titanium, depending on the specific needs of the application. These materials are selected to ensure long service life and minimal maintenance requirements.
However, shell and tube heat exchangers do have some limitations. One of the primary concerns is fouling, which occurs when deposits such as dirt, scale, or biological growth build up on the surfaces of the tubes or inside the shell. This reduces heat transfer efficiency and can lead to operational issues such as pressure drops and flow restrictions. Regular cleaning and maintenance are necessary to prevent fouling and to maintain the performance of the heat exchanger. Additionally, shell and tube heat exchangers can be bulky and require significant space for installation, which may not be ideal in applications with limited available space.
In terms of heat transfer efficiency, shell and tube heat exchangers are generally effective but may not be as efficient as other types of heat exchangers, such as plate heat exchangers, for certain applications. The efficiency of heat transfer depends on several factors, including the flow arrangement, the fluid properties, and the temperature difference between the two fluids. In some cases, the addition of heat transfer enhancement features, such as helically wound tubes or the use of extended surfaces, may be required to achieve the desired performance levels.
Despite these limitations, shell and tube heat exchangers remain a popular choice in industries where durability, flexibility, and high heat transfer rates are essential. Their ability to handle a wide range of fluids, pressures, and temperatures, coupled with their robust construction, make them indispensable in many industrial systems. Regular maintenance and proper design considerations can ensure their longevity and efficiency, making them a critical component in energy recovery, heating and cooling processes, and numerous other industrial operations.
Plate Heat Exchangers
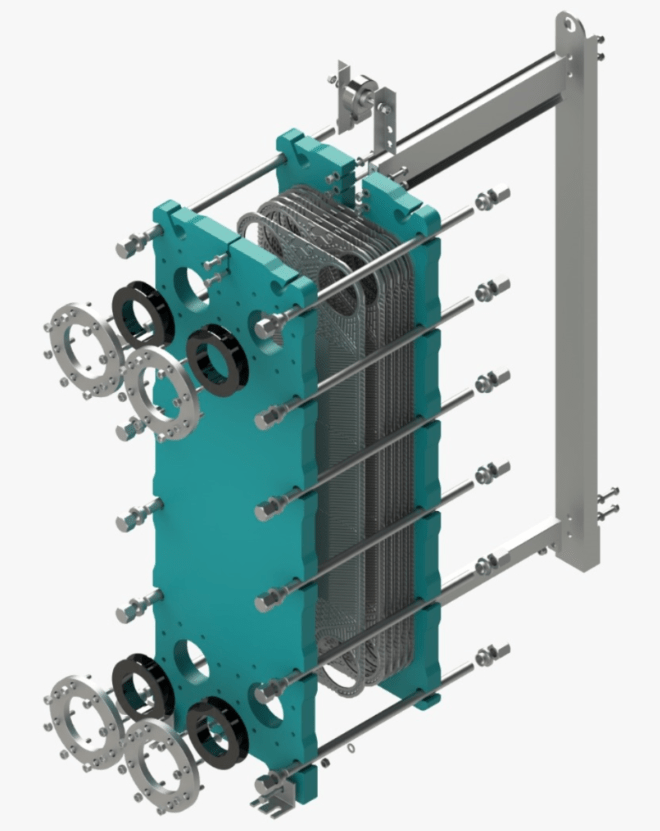
Plate heat exchangers are a type of heat exchanger that consists of multiple thin plates arranged in a stack, creating channels through which two fluids can flow, allowing heat to be transferred between them. The fluid flows through these channels, and heat is transferred from the hot fluid to the cooler fluid through the plates. The design of plate heat exchangers offers high heat transfer efficiency due to the large surface area provided by the plates and the turbulent flow generated as the fluids pass through narrow channels. This design also facilitates better thermal exchange between the fluids compared to other types of heat exchangers, such as shell and tube exchangers. Plate heat exchangers are commonly used in a wide range of applications, including heating, cooling, and heat recovery processes in industries like food and beverage, chemical processing, HVAC, and power generation.
One of the main advantages of plate heat exchangers is their compact and space-efficient design. The plates are thin, which allows for a large surface area in a relatively small space. This makes them particularly useful in applications where space is limited or where a high heat transfer rate is required within a smaller footprint. The plates are typically made from materials such as stainless steel, titanium, or other corrosion-resistant metals, ensuring durability and resistance to various fluids, including those with corrosive properties. Additionally, the modular design of plate heat exchangers allows for easy scalability, meaning that more plates can be added or removed from the unit to adjust the heat transfer capacity based on the specific needs of the system.
The working principle of plate heat exchangers relies on the flow arrangement of the fluids through the channels formed by the plates. The plates are arranged in a pattern that creates alternating hot and cold fluid channels. The fluids flow in opposite directions, which helps to maximize the temperature difference between them and enhances the efficiency of heat transfer. In many plate heat exchangers, the fluids flow in a counter-current direction, meaning that the hot fluid flows in one direction while the cold fluid flows in the opposite direction. This counterflow configuration increases the heat exchange rate and helps maintain a consistent temperature gradient, improving the overall heat transfer efficiency.
Plate heat exchangers also feature gaskets or welded seals to prevent leakage between the fluid channels, ensuring that the fluids do not mix. The gaskets are typically made from elastomers or other sealing materials that can withstand the temperatures and chemical properties of the fluids being processed. The ability to remove or replace individual plates in the unit makes plate heat exchangers easy to maintain and clean. Fouling, which is the accumulation of deposits like scale or biological growth, is a common issue in heat exchangers, but plate heat exchangers can be disassembled for cleaning, which helps restore their efficiency.
One of the significant benefits of plate heat exchangers is their high thermal efficiency, which is largely attributed to the turbulent flow conditions created by the narrow channels between the plates. This turbulence increases the rate of heat transfer by disrupting the boundary layer of the fluid, making the heat transfer more effective. Additionally, the high surface area provided by the plates enhances the overall heat exchange process, leading to better thermal performance compared to other heat exchanger designs with similar sizes.
In terms of versatility, plate heat exchangers are suitable for a wide range of fluids, including liquids, gases, and even slurries. The design can be tailored to meet the specific needs of different applications, with variations in the number of plates, the size of the channels, and the materials used. This flexibility makes plate heat exchangers ideal for use in industries such as food processing, pharmaceuticals, chemicals, and HVAC, where precise temperature control and efficient heat recovery are critical.
However, plate heat exchangers also have some limitations. While they are highly efficient, they may not be suitable for all types of applications, particularly those involving very high pressures or temperatures. In such cases, shell and tube heat exchangers may be more appropriate due to their ability to handle higher pressures and larger flow rates. Plate heat exchangers are also more prone to clogging in systems where the fluids contain particulate matter, requiring more frequent maintenance and cleaning. Additionally, the gaskets used in plate heat exchangers can degrade over time, especially in aggressive fluid environments, requiring periodic replacement.
Despite these limitations, plate heat exchangers offer numerous advantages, including high thermal efficiency, compact design, ease of maintenance, and adaptability to different fluids and applications. Their ability to provide effective heat transfer in a small footprint makes them an essential component in a variety of industries, contributing to energy savings, improved system performance, and reduced environmental impact. With ongoing advancements in materials and design, plate heat exchangers continue to evolve and remain a popular choice for industrial and commercial heat exchange applications.
Air-to-Air Heat Exchangers
Air-to-air heat exchangers are devices designed to transfer heat between two air streams without mixing them. These systems are commonly used in various applications where one air stream needs to be cooled or heated, while the other air stream serves as the source or sink of heat. They are widely used in ventilation systems, HVAC (Heating, Ventilation, and Air Conditioning) applications, industrial processes, and energy recovery systems to improve energy efficiency, reduce energy consumption, and enhance overall system performance. By recovering heat from one air stream and transferring it to another, air-to-air heat exchangers help to minimize the need for external heating or cooling, leading to significant energy savings and environmental benefits.
The working principle of air-to-air heat exchangers is based on the concept of heat transfer through conduction and convection. The two air streams pass through separate channels or passages within the heat exchanger, ensuring that they do not mix. Heat is transferred from the warmer air stream to the cooler air stream via the walls of the heat exchanger, typically made of materials with good thermal conductivity, such as aluminum or stainless steel. The design of the heat exchanger maximizes the surface area for heat transfer, increasing the efficiency of the system. In some cases, the air streams flow in a counterflow or crossflow arrangement, where the warm air flows in one direction while the cold air flows in the opposite direction or across the warm air stream, enhancing the temperature gradient and improving the heat transfer process.
Air-to-air heat exchangers come in various configurations, including plate heat exchangers, rotary heat exchangers, and tube-and-fin designs. Plate heat exchangers consist of multiple plates stacked together, creating channels through which the air streams flow. The heat transfer surfaces are typically thin and have a large surface area to facilitate efficient thermal exchange. Rotary heat exchangers use a rotating wheel made of a material with high thermal conductivity to transfer heat from one air stream to the other. As the wheel rotates, it absorbs heat from the hot air stream and releases it to the cooler air stream, continuously transferring energy between the two air flows. Tube-and-fin designs feature a series of tubes with fins attached, allowing air to flow through the tubes while the fins increase the surface area for heat transfer. The specific design of an air-to-air heat exchanger depends on the requirements of the application, such as the desired heat recovery rate, space constraints, and air flow rates.
One of the primary advantages of air-to-air heat exchangers is their ability to recover and reuse energy without mixing the two air streams, making them ideal for applications where contamination between the air streams is a concern, such as in clean rooms, laboratories, or industrial processes involving hazardous materials. Additionally, these heat exchangers can significantly reduce the need for external heating or cooling, as they allow for the transfer of thermal energy between incoming and outgoing air, resulting in lower energy consumption. For example, in a building ventilation system, an air-to-air heat exchanger can preheat or precool the incoming fresh air by using the exhaust air’s thermal energy, reducing the load on the building’s heating or cooling systems and improving the overall efficiency of the HVAC system.
Air-to-air heat exchangers are also beneficial for improving indoor air quality. In systems where outdoor air needs to be introduced into a building or facility, the heat exchanger allows for the exchange of thermal energy without introducing pollutants or contaminants from the outdoor air into the indoor environment. This is particularly important in buildings that require a high level of indoor air quality, such as hospitals, schools, and commercial buildings. By maintaining a balanced air exchange while recovering heat, air-to-air heat exchangers help to ensure that indoor environments remain comfortable and healthy.
Despite their many advantages, air-to-air heat exchangers also have some limitations. One of the main challenges is the potential for fouling or clogging of the heat exchanger surfaces, particularly if the air contains dust, moisture, or other particulate matter. Over time, this can reduce the efficiency of heat transfer and may require regular cleaning or maintenance to ensure optimal performance. Additionally, air-to-air heat exchangers are typically more effective in applications with moderate temperature differences between the air streams. In extreme temperature conditions, their performance may be less efficient compared to other types of heat exchangers, such as air-to-water heat exchangers or evaporative cooling systems.
Another limitation is the space requirement for installation. Depending on the design and size of the system, air-to-air heat exchangers may require a significant amount of space, which could be a concern in applications with limited available space, such as in residential buildings or compact industrial environments. The size of the heat exchanger may also be influenced by the air flow rates and the temperature recovery requirements of the system, further impacting the space needed for installation.
In addition, while air-to-air heat exchangers are effective at transferring heat between air streams, they cannot achieve the same level of heat transfer as liquid-based heat exchangers in some high-demand applications. For instance, in systems that require rapid or large-scale heat exchange, such as in heavy industrial processes or large-scale power plants, liquid-to-liquid heat exchangers may be preferred due to their higher heat transfer capacity and versatility.
Despite these challenges, air-to-air heat exchangers are an important technology in improving energy efficiency and reducing environmental impact in a variety of settings. They offer a simple, reliable, and cost-effective solution for recovering waste heat and optimizing thermal energy use. Whether in residential, commercial, or industrial applications, air-to-air heat exchangers provide significant benefits by reducing energy consumption, improving indoor comfort, and contributing to more sustainable building operations. As energy efficiency and environmental sustainability become increasingly important, the role of air-to-air heat exchangers in achieving these goals is expected to continue growing.
Regenerative Heat Exchangers
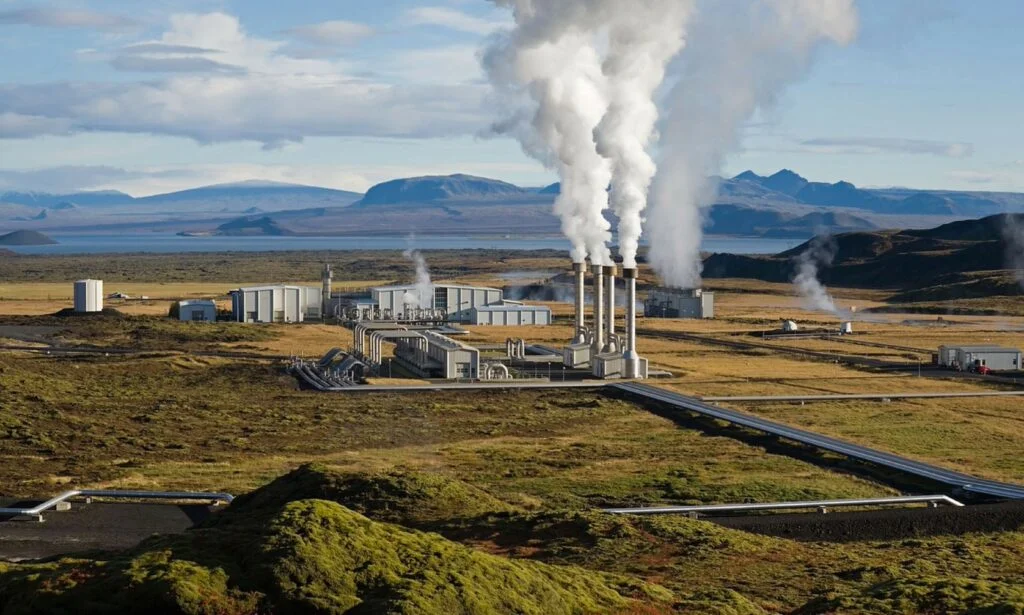
Regenerative heat exchangers are a type of heat recovery system designed to transfer thermal energy between two fluid streams in a way that maximizes efficiency by temporarily storing heat in a solid material, which is then transferred to another fluid. These heat exchangers are often used in situations where there are significant temperature differences between the two fluids, or where the heat source and heat sink are in alternating cycles. Unlike conventional heat exchangers that directly transfer heat from one fluid to another, regenerative heat exchangers temporarily store heat in the solid medium during one phase of the process and release it to the other fluid during the subsequent phase. This cyclical process allows for highly efficient heat transfer and energy recovery.
The working principle behind regenerative heat exchangers is based on thermal storage. Typically, these heat exchangers consist of a solid matrix, often made from materials with high heat capacity and thermal conductivity, such as metal or ceramic. The fluid carrying heat flows through the matrix and heats it up, and then, during the next phase of the cycle, a cooler fluid passes through the matrix, transferring the heat that was stored in the material. This process can be continuous, with the heat exchanger alternating between heating and cooling phases, depending on the design of the system.
Regenerative heat exchangers are most commonly found in applications where the temperature of the fluid changes cyclically or intermittently, such as in furnace systems, air preheating in combustion processes, and thermal energy recovery systems. A well-known example is the regenerative air preheater used in power plants, where exhaust gases are used to preheat the incoming combustion air. In these applications, the regenerative heat exchanger helps to reduce the need for additional fuel by using waste heat to raise the temperature of the air entering the combustion process. This leads to improved efficiency and reduced operating costs.
One of the key advantages of regenerative heat exchangers is their ability to provide high thermal efficiency. Because the heat is stored in a solid matrix and then transferred to another fluid, the temperature gradients between the fluids are maximized, which improves the overall heat transfer. The cyclic nature of the heat exchange process allows for continuous operation with minimal energy loss, making regenerative heat exchangers particularly suitable for systems where constant temperature control is required.
Another benefit is that regenerative heat exchangers can be designed to operate in extreme conditions. The solid heat storage materials can be chosen to withstand very high temperatures and harsh operating environments, making them ideal for use in industries like steel manufacturing, glass production, and other high-temperature processes. The ability to operate at high temperatures without compromising the material properties of the heat exchanger makes regenerative heat exchangers a reliable choice for industries with demanding thermal management requirements.
In addition, regenerative heat exchangers can contribute significantly to energy savings and environmental sustainability. By recovering waste heat and reusing it within the system, regenerative heat exchangers reduce the need for external energy input, thus lowering fuel consumption and greenhouse gas emissions. This not only makes the process more cost-effective but also helps to reduce the overall carbon footprint of industrial operations. In sectors where energy consumption is a major cost factor, the integration of regenerative heat exchangers can have a substantial economic impact, providing a clear incentive for their use.
Despite their many advantages, regenerative heat exchangers also have certain limitations. One of the primary challenges is the complexity of their design and operation. The need for a solid matrix to store heat, along with the alternating flow of hot and cold fluids, requires careful engineering to ensure that the system operates efficiently. The materials used for the matrix must be chosen for their ability to withstand thermal cycling without degradation. Furthermore, because of the cyclical nature of the heat exchange process, regenerative heat exchangers are typically less suitable for applications where continuous heat exchange is needed without interruption. They are ideal for processes with periodic or cyclic thermal demands but may not be as effective in systems requiring steady-state heat transfer.
Maintenance can also be a concern with regenerative heat exchangers. Over time, the solid matrix can accumulate contaminants or undergo thermal stress, leading to a decrease in its heat storage capacity or even damage to the material. Regular inspection, cleaning, and maintenance are essential to ensure the longevity and optimal performance of the system. Additionally, the moving parts associated with the cycling process may experience wear and tear, which could require more frequent maintenance compared to traditional heat exchangers.
In summary, regenerative heat exchangers are a highly efficient and effective technology for recovering and reusing thermal energy in applications where there are periodic or alternating temperature changes. Their ability to store and transfer heat between fluid streams enhances the overall thermal efficiency of the system, leading to significant energy savings and reduced operational costs. While they may not be suitable for all types of applications, regenerative heat exchangers offer a valuable solution in industries with high-temperature processes and cyclical heating and cooling needs. Their ability to reduce energy consumption and minimize environmental impact makes them an important technology in the pursuit of more sustainable industrial operations.
Plate Fin Heat Exchangers
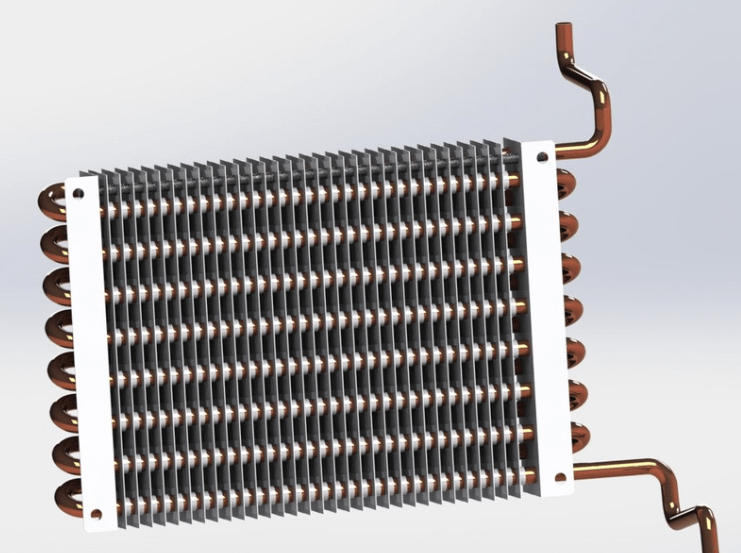
Plate fin heat exchangers are compact and efficient thermal devices used to transfer heat between two fluid streams. They are designed with a series of flat plates and fins arranged in a stack, which creates multiple parallel flow paths for the fluids. The plates typically serve as the primary heat transfer surface, while the fins enhance the surface area and improve heat transfer efficiency by promoting turbulence in the fluid flow. Plate fin heat exchangers are widely used in applications that require high thermal efficiency and a compact design, such as in aerospace, refrigeration, chemical processing, and HVAC systems.
The working principle of a plate fin heat exchanger is based on the transfer of thermal energy from one fluid to another through the plate surfaces. One fluid flows through the channels formed by the plates, while the other fluid flows through the spaces between the fins. As the fluids pass through the system, heat is transferred from the hotter fluid to the cooler fluid by conduction through the plates and fins. The fins increase the surface area for heat transfer, which results in a higher heat exchange rate in a smaller space. The arrangement of the plates and fins allows for efficient heat transfer even with relatively low fluid velocities, making plate fin heat exchangers ideal for applications where space is limited and high performance is needed.
One of the key advantages of plate fin heat exchangers is their compact and lightweight design. The use of thin plates and fins allows for a large heat transfer area within a small footprint, which is especially beneficial in applications such as aerospace and automotive industries, where space and weight constraints are critical. In addition to their compactness, the design can be customized to suit specific heat transfer requirements by adjusting the number of plates, the size of the fins, and the flow arrangement. This flexibility allows for optimization of the heat exchanger’s performance in various applications, from high-efficiency cooling systems to energy recovery processes.
Plate fin heat exchangers are also known for their high thermal performance. The combination of plates and fins promotes turbulence in the fluid flow, which increases the heat transfer coefficient and enhances the efficiency of thermal energy exchange. The use of multiple channels for the fluids allows for more effective contact between the fluids and the heat transfer surfaces, improving heat exchange even when the temperature differences between the fluids are small. This makes plate fin heat exchangers particularly effective in applications where high thermal efficiency is required, such as in refrigeration systems, air conditioning units, and power generation plants.
Another advantage of plate fin heat exchangers is their versatility. They can handle a wide range of fluids, including gases, liquids, and mixtures, which makes them suitable for a variety of industrial applications. For example, in the oil and gas industry, plate fin heat exchangers are used for cooling or heating fluids in refinery processes, while in the chemical industry, they are employed in processes that involve corrosive or volatile substances. Their ability to accommodate different fluids and operating conditions makes them adaptable to various sectors, from industrial manufacturing to energy generation.
However, despite their many advantages, plate fin heat exchangers also have some limitations. One of the main challenges is their susceptibility to fouling, which occurs when particles, scale, or other contaminants accumulate on the heat transfer surfaces, reducing the efficiency of heat transfer. Since the channels in plate fin heat exchangers are often small and narrow, they are more prone to clogging than other types of heat exchangers. Regular cleaning and maintenance are essential to ensure optimal performance, particularly in applications where the fluids may contain particulate matter or other contaminants.
Another limitation is that plate fin heat exchangers are typically designed for specific fluid flow arrangements, such as parallel flow, counterflow, or crossflow. While these configurations provide excellent heat transfer in many situations, they may not be as effective in applications where the fluid flow needs to be more flexible or dynamic. Additionally, the cost of manufacturing plate fin heat exchangers can be higher compared to other types of heat exchangers, due to the precision required in manufacturing the plates and fins, and the materials used in their construction.
Despite these challenges, plate fin heat exchangers are widely used in applications that demand compactness, high thermal performance, and versatility. Their ability to provide efficient heat transfer in a small and lightweight design makes them invaluable in industries such as aerospace, automotive, HVAC, and chemical processing. With ongoing advancements in materials and design techniques, plate fin heat exchangers continue to evolve, offering improved performance and durability in a wide range of demanding applications. Their role in energy recovery and heat management remains a key factor in optimizing system efficiency and reducing environmental impact in many industrial sectors.
Applications of Exhaust Gas Heat Exchangers
Exhaust gas heat exchangers play a vital role in various industries by recovering thermal energy from exhaust gases that would otherwise be wasted. These heat exchangers are designed to capture and transfer heat from hot exhaust gases to other fluid streams, which can then be used for heating, power generation, or preheating purposes. This process not only enhances the energy efficiency of industrial systems but also contributes to reducing overall fuel consumption, lowering operating costs, and decreasing environmental impact by reducing greenhouse gas emissions. Exhaust gas heat exchangers find applications in a wide range of sectors, including power generation, automotive, manufacturing, HVAC systems, and marine industries.
In power generation, exhaust gas heat exchangers are used to recover heat from the exhaust gases of gas turbines, boilers, or internal combustion engines. The heat recovered from the exhaust gases can be used to preheat incoming combustion air or feedwater, which improves the efficiency of the power generation process. By reducing the need for additional fuel to heat these fluids, the overall fuel consumption of the power plant is reduced, leading to lower operational costs and improved sustainability. Exhaust gas heat exchangers are also commonly used in combined-cycle power plants, where waste heat from gas turbines is used to generate steam, which drives a steam turbine to produce additional power. This system significantly boosts the efficiency of power generation by utilizing the waste heat that would otherwise be lost to the environment.
In the automotive industry, exhaust gas heat exchangers are employed in various applications to enhance the performance and efficiency of vehicles. They are often used in exhaust gas recirculation (EGR) systems to recover heat from the engine’s exhaust gases and use it to heat the incoming air, improving engine efficiency and reducing emissions. Exhaust gas heat exchangers are also used in the cooling systems of diesel and gasoline engines, where the heat from the exhaust gases is transferred to coolant or air, helping to regulate the engine temperature and prevent overheating. By optimizing engine temperature and reducing the need for additional cooling systems, exhaust gas heat exchangers contribute to fuel efficiency and performance.
In industrial manufacturing processes, exhaust gas heat exchangers are widely used to recover heat from exhaust gases produced during combustion or other high-temperature processes. For example, in cement plants, steel mills, and glass production facilities, large volumes of hot exhaust gases are generated, and using exhaust gas heat exchangers allows these industries to recover thermal energy and use it for various purposes, such as drying, preheating, or heating incoming process materials. This not only reduces the need for additional heating systems but also improves the overall energy efficiency of the facility, lowering fuel consumption and associated costs. Additionally, it helps industries meet regulatory requirements related to energy efficiency and emissions.
In HVAC systems, exhaust gas heat exchangers are used for heat recovery ventilation (HRV) and energy recovery ventilation (ERV) systems. These systems are designed to improve the energy efficiency of buildings by recovering heat from exhaust air and transferring it to incoming fresh air, which helps to maintain indoor comfort while reducing the load on heating or cooling systems. By preheating or precooling the incoming air, these heat exchangers help to minimize the energy required to maintain a comfortable indoor temperature, leading to significant savings on heating and cooling costs. This is especially beneficial in commercial buildings, residential homes, and industrial facilities where efficient energy use is a priority.
In the marine industry, exhaust gas heat exchangers are used on ships and offshore platforms to recover heat from the exhaust gases of marine engines. The heat recovered can be used for various purposes, such as heating fuel, providing hot water, or preheating the air in the engine room. In addition to improving energy efficiency, these heat exchangers help reduce the environmental impact of maritime operations by reducing the fuel consumption of onboard systems. Exhaust gas heat exchangers are also critical in maintaining engine performance and preventing overheating, which can lead to costly repairs and downtime.
Exhaust gas heat exchangers are also used in the chemical, petrochemical, and food processing industries, where they help recover heat from exhaust gases produced during various manufacturing processes. In the chemical industry, for example, exhaust gas heat exchangers are used to preheat raw materials, heat process fluids, or generate steam for use in other parts of the plant. In food processing, these heat exchangers can recover heat from exhaust gases produced during cooking, drying, or sterilization processes, improving energy efficiency and reducing the need for additional heating or cooling systems. By recovering and reusing waste heat, these industries can reduce their overall energy consumption and minimize environmental impacts.
The application of exhaust gas heat exchangers is also expanding in renewable energy systems, such as biomass and waste-to-energy plants. In these systems, exhaust gases produced during the combustion of organic materials or waste are recovered using heat exchangers to generate steam or heat water for various uses, including district heating or electricity generation. This process helps improve the efficiency of renewable energy systems by reducing the need for external fuel inputs and making use of the thermal energy contained in the exhaust gases.
In addition to the direct energy recovery benefits, the use of exhaust gas heat exchangers also contributes to improving environmental sustainability. By recovering and reusing waste heat, these systems help reduce the overall carbon footprint of industrial operations. This is particularly important as industries strive to meet increasingly stringent environmental regulations and reduce their greenhouse gas emissions. Moreover, exhaust gas heat exchangers help minimize the need for additional fuel to generate heat, which contributes to reducing the overall consumption of fossil fuels and mitigating the environmental impact associated with their use.
In conclusion, exhaust gas heat exchangers are versatile and efficient systems that play a crucial role in improving energy efficiency across a wide range of industries. By recovering heat from exhaust gases and transferring it to other fluids or processes, these heat exchangers help to reduce fuel consumption, lower operational costs, and reduce environmental impact. Their applications span across power generation, automotive, industrial manufacturing, HVAC systems, marine, and renewable energy sectors, providing significant benefits in terms of energy savings and sustainability. As industries continue to focus on improving energy efficiency and reducing their carbon footprints, the use of exhaust gas heat exchangers is expected to grow, contributing to a more sustainable and cost-effective future.
Power Generation
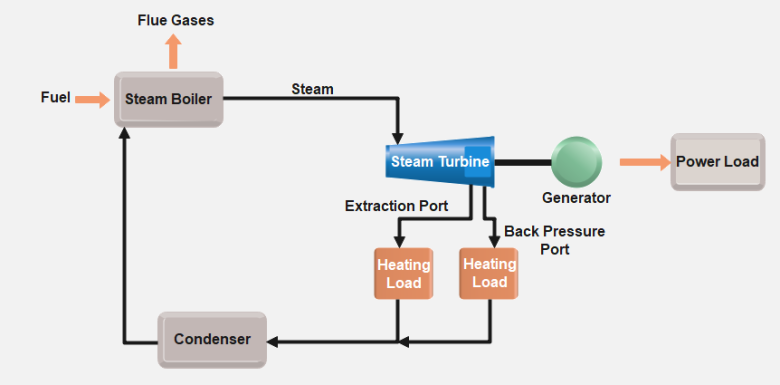
Power generation is the process of producing electricity from various energy sources to meet the needs of residential, commercial, industrial, and infrastructure systems. This process involves converting primary energy sources, such as fossil fuels, nuclear energy, renewable resources, or even waste, into electrical power that can be distributed through grids and consumed by end-users. The method of power generation used in a particular region or facility depends on several factors, including resource availability, economic considerations, environmental impact, and technological advancements. Power generation is a critical component of modern society, powering homes, industries, transportation, and enabling the functioning of economies worldwide.
The most traditional form of power generation is through the combustion of fossil fuels, such as coal, natural gas, and oil. In thermal power plants, these fuels are burned to produce heat, which is used to generate steam. The steam drives a turbine connected to a generator, which produces electricity. Fossil fuel-based power plants are widely used because they are relatively simple and established technologies. However, they also have significant environmental impacts, primarily due to the emissions of carbon dioxide (CO2), sulfur dioxide (SO2), and nitrogen oxides (NOx), which contribute to air pollution, climate change, and acid rain. Efforts to mitigate these environmental impacts include the development of carbon capture and storage (CCS) technologies, which aim to capture CO2 emissions before they enter the atmosphere.
Another common method of power generation is nuclear power, where nuclear fission reactions are used to produce heat, which is then used to generate steam and drive a turbine. Nuclear power plants have the advantage of being highly efficient and producing large amounts of electricity with relatively low emissions of CO2. However, nuclear power comes with concerns about radioactive waste, the risk of nuclear accidents, and the high initial cost of building and maintaining nuclear power plants. Despite these challenges, nuclear energy remains an important part of the energy mix in many countries, particularly in those that seek to reduce their reliance on fossil fuels and greenhouse gas emissions.
Renewable energy sources, including solar, wind, hydro, geothermal, and biomass, are gaining increasing importance in power generation due to their lower environmental impact and sustainability. Solar power, for example, harnesses energy from the sun using photovoltaic (PV) panels or concentrated solar power (CSP) systems. These systems convert sunlight directly into electricity, and as solar technology continues to improve, it has become one of the fastest-growing sources of electricity generation worldwide. Similarly, wind power uses turbines to convert the kinetic energy of wind into electrical power. Wind farms, both onshore and offshore, are now a significant source of renewable energy in many countries, and their capacity is increasing rapidly.
Hydropower, or hydroelectric power, has been used for centuries to generate electricity. In a hydroelectric power plant, water stored in reservoirs is released to flow through turbines, which generate electricity as the water moves. Hydropower is one of the oldest and most reliable sources of renewable energy, providing a substantial percentage of the world’s electricity. However, large-scale hydropower projects can have environmental and social impacts, including ecosystem disruption and displacement of communities, which has led to a growing interest in smaller, more sustainable forms of hydroelectric generation.
Geothermal power generation utilizes the heat from beneath the Earth’s surface to produce steam, which drives turbines connected to generators. This form of energy is particularly useful in regions with significant geothermal activity, such as Iceland, parts of the United States, and the Philippines. Geothermal energy is reliable, renewable, and has a low environmental impact, making it an attractive alternative to fossil fuels in suitable locations. Similarly, biomass power generation involves burning organic materials, such as wood, agricultural waste, or dedicated energy crops, to produce heat and electricity. Biomass can be a carbon-neutral energy source, as the CO2 released during combustion is offset by the CO2 absorbed by plants during growth. However, there are concerns about the sustainability of large-scale biomass production, particularly in terms of land use and deforestation.
While renewable energy sources have gained momentum, the transition away from fossil fuels and nuclear power has presented challenges related to energy storage, grid integration, and the variability of renewable sources like wind and solar. Energy storage systems, such as batteries, pumped hydro storage, and compressed air energy storage, are being developed to store excess energy generated during times of high production and release it during periods of low generation. These storage solutions help smooth out the intermittency of renewable energy sources and ensure a stable and reliable supply of electricity.
Combined-cycle power generation, which combines gas turbines and steam turbines, is an example of a more efficient system that maximizes energy output by using both gas and steam turbines in a single process. In a combined-cycle power plant, natural gas is burned to drive a gas turbine, and the waste heat from the gas turbine is used to generate steam that drives a steam turbine. This system increases the overall efficiency of power generation, as it captures and utilizes waste heat that would otherwise be lost in a traditional single-cycle power plant.
Cogeneration and combined heat and power (CHP) systems are also growing in importance as energy efficiency technologies. In these systems, both electricity and useful heat are produced from a single fuel source, such as natural gas, biomass, or waste heat. The heat generated in the process is typically used for heating buildings or industrial processes, making cogeneration systems highly efficient in terms of energy use. Cogeneration is commonly used in industrial plants, district heating systems, and commercial buildings, where the demand for both electricity and heat can be met simultaneously.
The global shift toward more sustainable energy systems has spurred the development of new technologies in power generation. Advancements in energy efficiency, grid modernization, and smart grid technologies are allowing for better integration of renewable energy into existing power grids, optimizing the distribution and consumption of electricity. Additionally, innovations in power generation technologies, such as advanced nuclear reactors, hydrogen power, and wave energy, hold promise for further reducing the environmental impact of power generation while ensuring reliable and secure electricity supplies for the future.
Power generation is at the heart of the energy system, and its evolution is driven by the need to balance environmental, economic, and social considerations. The growing demand for clean and sustainable energy sources is reshaping the global energy landscape, pushing for advancements in renewable energy technologies and the development of systems that maximize energy efficiency and minimize emissions. As the world continues to transition toward a more sustainable energy future, power generation technologies will play a critical role in meeting the challenges of climate change, energy security, and economic development.
Industrial manufacturing is the backbone of modern economies, providing the goods and products that meet the needs of society, from raw materials to finished consumer products. It encompasses a wide range of processes and industries that involve the transformation of raw materials into finished goods through various physical, chemical, and mechanical methods. These processes are carried out in factories, plants, and industrial facilities, where the integration of machinery, labor, and technology allows for large-scale production. Industrial manufacturing spans a broad spectrum, including sectors such as automotive, aerospace, electronics, textiles, food processing, pharmaceuticals, chemicals, and metals, among others.
One of the key drivers of industrial manufacturing is the need for efficiency. As industries seek to maximize productivity while minimizing costs, they rely heavily on advanced technologies, automation, and optimization techniques. Automation has become a critical component in industrial manufacturing, where robots and automated machinery perform repetitive tasks, leading to increased precision, reduced human error, and faster production times. In many industries, the integration of industrial robots has revolutionized manufacturing by streamlining assembly lines, performing welding, painting, packaging, and material handling with great speed and accuracy. This allows for the production of higher quantities of goods with consistent quality.
Additionally, the use of advanced manufacturing techniques such as 3D printing (additive manufacturing), computer numerical control (CNC) machining, and advanced casting and molding methods has further improved manufacturing capabilities. 3D printing, for example, allows for the creation of complex parts and prototypes with minimal waste, which is particularly valuable in industries like aerospace, automotive, and healthcare. CNC machining, on the other hand, enables the production of high-precision parts through automated control of machine tools, allowing for the efficient creation of complex metal, plastic, and composite components.
Energy efficiency is another critical aspect of industrial manufacturing. Factories and production plants are large consumers of energy, particularly in energy-intensive industries like steelmaking, cement production, and chemical manufacturing. As concerns over climate change and energy costs grow, manufacturers are increasingly adopting energy-efficient technologies and processes. These include the implementation of heat recovery systems, the optimization of electrical systems, and the integration of renewable energy sources into manufacturing operations. The goal is to reduce energy consumption, lower operational costs, and minimize the environmental impact of production activities.
Industrial manufacturing is also significantly impacted by the need for sustainable practices. With growing awareness of environmental issues, many industries are shifting towards greener production methods. This includes the use of sustainable materials, such as biodegradable plastics and recycled metals, as well as the reduction of waste through lean manufacturing principles. Lean manufacturing focuses on minimizing waste in all forms, including time, materials, and labor, to optimize production efficiency. This approach helps companies cut costs, improve quality, and meet regulatory requirements while also supporting sustainability goals.
The role of supply chains in industrial manufacturing is crucial, as it enables the efficient movement of raw materials, components, and finished products between suppliers, manufacturers, and customers. Globalization has expanded supply chains, allowing manufacturers to source materials and components from all over the world, which has led to the optimization of production and reduced costs. However, managing complex supply chains requires advanced logistical systems and real-time data analytics to ensure smooth operations and reduce delays. Manufacturers often rely on just-in-time (JIT) inventory systems, which allow them to minimize stock levels and receive materials only when needed for production, helping to reduce storage costs and waste.
Technology is also playing an increasingly important role in the monitoring and management of industrial manufacturing processes. The rise of Industry 4.0, often referred to as the Fourth Industrial Revolution, is characterized by the integration of digital technologies such as the Internet of Things (IoT), artificial intelligence (AI), machine learning, big data analytics, and cloud computing into manufacturing operations. These technologies enable real-time monitoring of equipment performance, predictive maintenance, and enhanced decision-making, which collectively improve operational efficiency, reduce downtime, and extend the lifespan of machinery. IoT sensors, for instance, can detect when a machine is operating inefficiently or when it requires maintenance, allowing for corrective action to be taken before a failure occurs, preventing costly repairs and unplanned downtime.
As manufacturing processes become more advanced, the workforce is also evolving. The demand for skilled workers, particularly those with expertise in advanced manufacturing technologies, data analysis, and robotics, is on the rise. This shift is prompting many industries to invest in workforce development, training programs, and partnerships with educational institutions to ensure that workers have the necessary skills to thrive in a technology-driven manufacturing environment. Automation, while improving efficiency, has also led to concerns about job displacement, but it has also created new roles and opportunities in industries that require higher levels of technical expertise.
The global nature of industrial manufacturing has led to challenges related to quality control, standardization, and regulatory compliance. Manufacturers often have to navigate complex international regulations and standards, particularly when producing goods for export. This requires a thorough understanding of different market requirements and an ability to meet the specifications set by various regulatory bodies. In highly regulated industries such as pharmaceuticals, automotive, and aerospace, quality control is paramount, and manufacturers must adhere to strict standards to ensure the safety and reliability of their products. Advanced quality assurance methods, such as statistical process control (SPC), automated inspection systems, and Six Sigma, are commonly employed to maintain high levels of quality and reduce defects in production.
The importance of innovation in industrial manufacturing cannot be overstated. As markets become more competitive, manufacturers must continuously innovate to meet consumer demand for higher-quality, more affordable, and faster products. Research and development (R&D) are essential in driving innovation, as manufacturers explore new materials, production techniques, and technologies that can improve product performance, reduce costs, and open up new market opportunities. In some industries, such as automotive and electronics, R&D is crucial for developing new product lines and maintaining a competitive edge. As the manufacturing landscape evolves, companies that embrace innovation and adaptability are better positioned to succeed in the face of changing market conditions and consumer preferences.
Industrial manufacturing is also deeply tied to the concept of economic development. Manufacturing industries provide employment opportunities, contribute to GDP, and play a significant role in a country’s industrial base. For many nations, a strong manufacturing sector is vital for economic growth, technological advancement, and competitiveness in the global marketplace. As a result, governments often implement policies and incentives to support manufacturing, such as research grants, tax incentives, infrastructure development, and trade agreements.
In conclusion, industrial manufacturing is a dynamic and critical sector that encompasses a wide range of processes, industries, and technologies. From traditional production methods to advanced automation, energy efficiency, and sustainability practices, manufacturing continues to evolve to meet the demands of a rapidly changing world. The integration of new technologies, the optimization of production systems, and the drive for innovation will continue to shape the future of industrial manufacturing, ensuring that it remains an essential part of the global economy. As industries strive for greater efficiency, sustainability, and technological advancement, industrial manufacturing will remain a cornerstone of modern society and economic development.
Refining and Petrochemical Plants
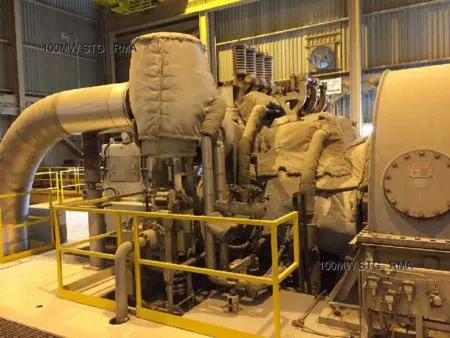
Refining and petrochemical plants are essential components of the global energy and chemical industries. These plants are responsible for processing raw materials, primarily crude oil and natural gas, into a wide range of valuable products that are used across various sectors, including energy, manufacturing, transportation, agriculture, and consumer goods. The refining process primarily focuses on transforming crude oil into fuels, such as gasoline, diesel, kerosene, and jet fuel, while petrochemical plants produce chemicals that serve as raw materials for plastics, fertilizers, synthetic fibers, and other industrial products. Both refining and petrochemical processes are highly complex and rely on advanced technologies, large-scale infrastructure, and strict regulatory compliance to ensure safety, efficiency, and environmental sustainability.
In refining operations, crude oil is first subjected to distillation, where it is heated and separated into various fractions based on their boiling points. The lighter fractions, such as gasoline and kerosene, are distilled at lower temperatures, while heavier fractions, such as diesel, fuel oil, and bitumen, are separated at higher temperatures. This process is the foundation of the refining industry and produces the base components that are further processed and refined to meet the specific needs of various markets. Once distillation is complete, other refining processes such as cracking, reforming, and treating are employed to break down heavier fractions into lighter, more useful products, improve the quality of fuels, and remove impurities.
Cracking is one of the most critical processes in modern refineries. In this process, large hydrocarbon molecules are broken down into smaller ones to produce high-demand products like gasoline, diesel, and other lighter fuels. There are several types of cracking processes, including thermal cracking, catalytic cracking, and hydrocracking. Catalytic cracking uses a catalyst to speed up the reaction, producing higher yields of gasoline and diesel, while hydrocracking uses hydrogen to break down heavy oils into lighter, cleaner fuels. These methods are essential for refining crude oil into products that meet the specifications for modern engines and environmental standards.
Reforming is another key process in refining, which alters the chemical structure of hydrocarbons to improve the quality of gasoline and other products. Catalytic reforming uses a catalyst to increase the octane rating of gasoline, making it more suitable for high-performance engines. In addition to producing high-quality fuels, reforming also produces hydrogen, which is used in other refinery processes such as hydrocracking and desulfurization.
Desulfurization is a crucial process in refining to remove sulfur compounds from crude oil and its refined products. Sulfur in fuels leads to the production of harmful sulfur dioxide emissions when burned, which contributes to air pollution and acid rain. The process involves the use of hydrogen in a hydrodesulfurization unit, where sulfur is chemically removed from the hydrocarbons. This process ensures that refined products comply with environmental regulations aimed at reducing harmful emissions and improving air quality.
Petrochemical plants, on the other hand, focus on converting natural gas liquids (NGLs) and refinery byproducts into a variety of chemical products. These plants use a range of processes such as cracking, polymerization, alkylation, and aromatization to produce petrochemicals. Petrochemicals are essential building blocks for the production of plastics, synthetic fibers, paints, adhesives, detergents, fertilizers, and many other industrial and consumer products. The most common petrochemicals produced are ethylene, propylene, benzene, toluene, and xylene, which serve as the foundation for countless applications.
Ethylene is one of the most widely used petrochemicals, produced by cracking hydrocarbons like ethane, propane, or naphtha. It is primarily used to make polyethylene, the most common plastic in the world, as well as other products such as ethylene glycol (used in antifreeze and polyester production), and styrene (used in the production of polystyrene and synthetic rubber). Propylene, another key petrochemical, is used to make polypropylene, which is used in packaging, textiles, automotive components, and various consumer goods.
Petrochemical plants also play a significant role in the production of fertilizers, which are essential for global agriculture. Nitrogen-based fertilizers, such as ammonia, are produced through the Haber-Bosch process, which involves the synthesis of ammonia from nitrogen and hydrogen. Ammonia is then used to produce urea, ammonium nitrate, and other nitrogen fertilizers that support crop growth worldwide.
The petrochemical industry is deeply integrated with the refining process, as both rely on the same feedstocks, such as crude oil, natural gas, and their byproducts. Refineries and petrochemical plants are often co-located, allowing for the efficient transfer of raw materials and intermediate products between the two operations. This integration helps to optimize the use of resources and reduce operational costs, as both refining and petrochemical operations benefit from shared infrastructure, such as pipelines, storage facilities, and utilities.
Safety is a major concern in both refining and petrochemical operations due to the highly volatile and flammable nature of the materials being processed. To mitigate risks, refineries and petrochemical plants adhere to stringent safety standards and regulations that require regular inspections, maintenance, and upgrades to equipment. Advanced process control systems are used to monitor and control operations, ensuring that equipment operates within safe parameters and minimizing the risk of accidents. Additionally, emergency response plans and safety training programs are critical components of plant operations, helping to protect workers, the environment, and surrounding communities.
Environmental concerns are a growing challenge for both refining and petrochemical industries. Both sectors are energy-intensive and produce significant emissions, including greenhouse gases, volatile organic compounds (VOCs), and particulate matter, all of which can contribute to air pollution and climate change. As a result, refineries and petrochemical plants are under increasing pressure to reduce their environmental impact. Many plants have adopted advanced technologies, such as carbon capture and storage (CCS), to reduce CO2 emissions, and have implemented energy recovery systems to improve overall energy efficiency. Additionally, regulations governing the discharge of wastewater, air emissions, and solid waste require plants to take steps to minimize environmental pollution and ensure compliance with local and international standards.
The global refining and petrochemical industries are also facing increasing pressure to meet the growing demand for sustainable and cleaner products. Bio-based and renewable feedstocks are gaining attention as alternatives to traditional petroleum-based raw materials. For example, bioplastics, produced from renewable sources such as corn or sugarcane, are becoming more common as industries seek to reduce their dependence on fossil fuels and minimize the environmental impact of plastic waste. Additionally, there is a push toward the development of advanced biofuels, such as algae-based biofuels, which can be used as alternatives to gasoline and diesel in transportation.
In conclusion, refining and petrochemical plants are integral to the production of essential energy and chemical products that power industries and economies around the world. These plants use a variety of advanced processes to convert raw materials into fuels, chemicals, and other products, which are critical to modern life. As the world continues to focus on sustainability, the refining and petrochemical industries are evolving to meet the demand for cleaner, more energy-efficient, and environmentally responsible solutions. By adopting new technologies, improving efficiency, and integrating renewable resources, these industries can continue to play a central role in supporting global economic growth while addressing the challenges posed by climate change and resource scarcity.
The marine industry is a broad and diverse sector that encompasses a range of activities and services related to the design, construction, operation, and maintenance of ships, boats, and other vessels used for transportation, trade, recreation, and defense. It plays a vital role in the global economy, facilitating international trade, supporting coastal and offshore industries, and providing transportation and logistics services across vast distances. The marine industry is also a key player in the development of renewable energy technologies, such as offshore wind farms, and plays an important role in tourism and leisure activities. The sector is heavily influenced by global economic trends, technological advancements, environmental regulations, and geopolitical factors.
At the core of the marine industry is shipping, which is responsible for the transportation of goods across the world’s oceans. Shipping is by far the most cost-effective means of transporting large volumes of goods over long distances, with more than 80% of global trade being carried by sea. The ships that make up the global fleet vary widely in terms of size, design, and purpose. These include container ships, bulk carriers, tankers, passenger vessels, ferries, and specialized ships used in industries such as oil and gas exploration, fishing, and research. The marine industry also includes the ports and terminals where ships are loaded and unloaded, as well as the logistics networks that manage the movement of goods to and from these locations.
Shipping companies rely on various types of vessels to meet the diverse needs of global trade. Container ships, which transport standardized cargo in large containers, are the backbone of global trade, enabling the efficient movement of consumer goods, electronics, machinery, and raw materials. Bulk carriers are used to transport commodities such as coal, grain, and iron ore, while tankers are designed to carry liquid cargo, including crude oil, chemicals, and liquefied natural gas (LNG). Passenger vessels, such as cruise ships and ferries, are part of the leisure and tourism sector, offering transportation and entertainment to millions of people around the world each year. The oil and gas industry also relies on specialized vessels, such as offshore drilling rigs, supply ships, and seismic survey vessels, to explore and extract resources from the sea.
The design, construction, and maintenance of ships and vessels is a complex and highly specialized area of the marine industry. Shipbuilding is an ancient industry that has evolved over centuries, with modern shipyards using advanced technologies and materials to build ships that are faster, more efficient, and environmentally friendly. The process of designing a ship involves a detailed analysis of its intended use, the types of cargo or passengers it will carry, and the environmental conditions it will operate in. This is followed by the construction phase, which involves the assembly of the hull, propulsion system, and other essential components. Modern shipyards employ cutting-edge techniques, such as computer-aided design (CAD), 3D modeling, and simulation, to optimize the design and construction process. Shipbuilders also use advanced materials, such as high-strength steel, aluminum, and composite materials, to create vessels that are lightweight, durable, and fuel-efficient.
Maintenance and repair are critical aspects of the marine industry, ensuring that vessels remain safe, functional, and compliant with international regulations. Regular inspections, repairs, and upgrades are essential to maintain the integrity of a ship’s hull, engine, and other key systems. The marine industry is governed by a wide range of international regulations and standards, such as those set by the International Maritime Organization (IMO), which address safety, environmental protection, and the prevention of marine pollution. These regulations govern various aspects of maritime operations, including ship design, crew training, waste management, and emissions control, and compliance with these standards is essential for the safe and sustainable operation of vessels.
In addition to shipping and shipbuilding, the marine industry also includes a wide range of other activities, such as marine engineering, offshore exploration and production, and recreational boating. Marine engineering involves the design, construction, and maintenance of systems that support the operation of ships, including propulsion systems, navigation equipment, electrical systems, and cargo handling systems. Offshore exploration and production, particularly in the oil and gas sector, involves the extraction of resources from beneath the sea, often in deepwater environments. Offshore drilling rigs, production platforms, and subsea pipelines are used to extract and transport oil and gas from offshore fields to onshore facilities. The marine industry also plays a significant role in renewable energy development, particularly offshore wind energy. Offshore wind farms, which consist of large turbines installed in the ocean, are becoming an increasingly important source of clean energy, with several countries investing in the development of offshore wind projects.
The marine industry is also closely linked to the tourism and leisure sector. Cruise ships, luxury yachts, and recreational boats provide transportation and entertainment to millions of people each year. Cruise tourism has grown significantly in recent decades, with major cruise lines offering trips to destinations around the world, from the Caribbean and Mediterranean to the Arctic and Antarctic. The demand for recreational boating is also on the rise, with millions of people participating in boating and water sports as part of their leisure activities. Yacht building and ownership have become symbols of wealth and luxury, with high-end yachts featuring advanced technology, luxurious amenities, and custom designs.
Sustainability is an increasingly important issue for the marine industry. As global concerns over climate change and environmental degradation grow, the industry is under increasing pressure to reduce its environmental impact. Shipping is a major source of greenhouse gas emissions, air pollution, and marine pollution, and efforts are underway to develop cleaner, more energy-efficient technologies. The introduction of stricter emissions standards by international bodies such as the IMO has led to the development of new technologies, such as LNG-powered vessels, hybrid propulsion systems, and exhaust gas cleaning systems (scrubbers), which help reduce sulfur emissions and other pollutants. The industry is also exploring the use of alternative fuels, such as hydrogen and biofuels, to reduce its reliance on traditional fossil fuels and cut emissions. Furthermore, the issue of marine pollution, including plastic waste and oil spills, has led to efforts to improve waste management practices, enhance spill response capabilities, and develop technologies to reduce the environmental impact of shipping.
In addition to sustainability concerns, the marine industry also faces challenges related to geopolitical tensions, security threats, and the rise of digital technologies. Piracy and theft remain significant concerns in some regions, particularly in areas such as the Gulf of Aden, the Strait of Malacca, and the West African coast. As a result, shipping companies and governments have implemented measures to protect vessels and crews, including the use of armed security personnel, surveillance systems, and international cooperation. The increasing use of digital technologies, such as artificial intelligence, big data analytics, and blockchain, is also transforming the marine industry by improving efficiency, reducing costs, and enhancing safety. For example, digital twin technology allows operators to monitor and optimize the performance of vessels in real time, while blockchain technology can be used to streamline shipping logistics and improve supply chain transparency.
In conclusion, the marine industry is a critical and dynamic sector that encompasses a wide range of activities, from shipping and shipbuilding to offshore exploration, recreational boating, and renewable energy development. It plays a central role in global trade, tourism, and resource extraction, while also facing challenges related to sustainability, environmental impact, security, and technological advancements. As the industry continues to evolve, it will increasingly focus on the development of cleaner, more efficient technologies, digital transformation, and sustainable practices to meet the demands of a rapidly changing world.
Waste-to-Energy Plants
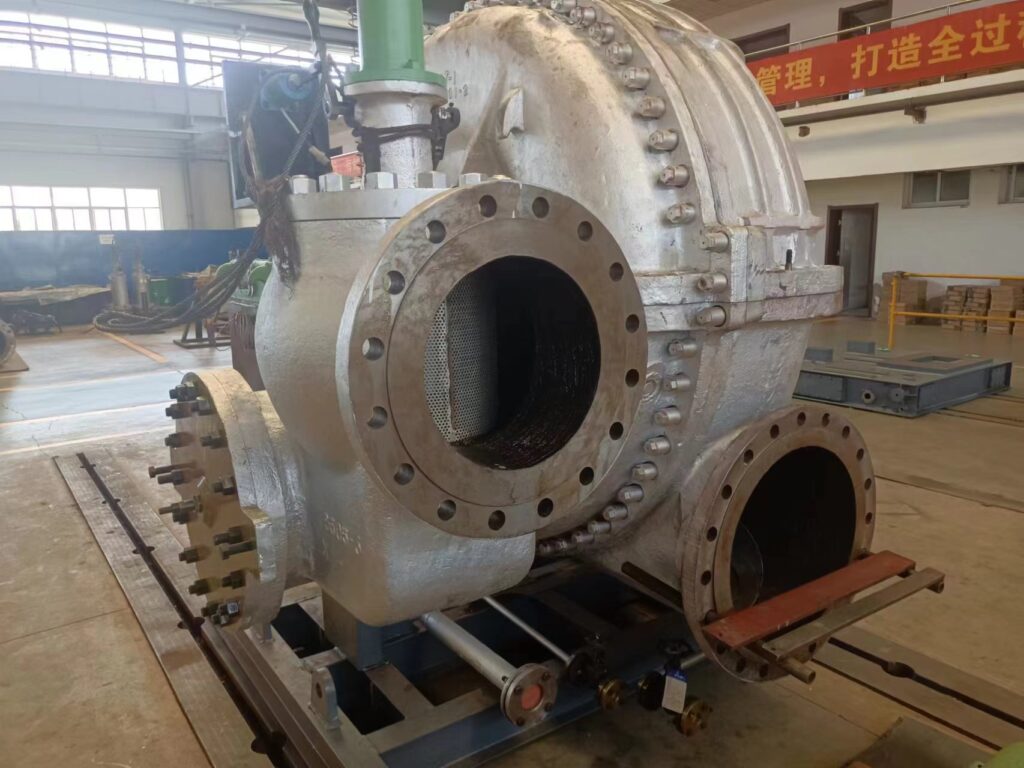
Waste-to-energy (WTE) plants are facilities that convert non-recyclable waste materials into usable forms of energy, typically electricity and heat. The technology behind these plants plays an important role in waste management and the generation of renewable energy, especially in urban areas where waste production is high. Waste-to-energy has gained traction as a sustainable solution to two critical global challenges: the growing waste management problem and the demand for clean, renewable energy. These plants help reduce the volume of waste that would otherwise end up in landfills, minimizing the environmental impact of waste disposal. In addition, they harness the energy embedded in waste materials, reducing the need for fossil fuels and lowering greenhouse gas emissions.
The process of converting waste into energy typically involves thermal treatment methods, although other technologies like biological and mechanical processes are also used. The most common method used in waste-to-energy plants is incineration, where waste is burned at high temperatures to produce heat. This heat is used to generate steam, which drives turbines connected to electricity generators. The process produces electricity that can be fed into the grid, providing power to homes and businesses. In addition to electricity generation, the heat from incineration can be used for district heating, where it is distributed to nearby buildings for space heating and hot water, offering an additional energy benefit.
WTE plants vary in their design and operational processes depending on the type of waste they handle and the technology employed. The waste that is processed in these plants includes household trash, industrial waste, and sometimes even agricultural and medical waste. However, it is essential that only non-recyclable, non-toxic waste is burned, as some materials can release harmful pollutants when combusted. To prevent this, waste-to-energy plants often include advanced filtration and scrubbing systems to capture harmful gases and particulates released during combustion. These systems ensure that emissions comply with strict environmental regulations, and their effectiveness is a crucial part of the plant’s operation.
One of the advantages of waste-to-energy technology is its ability to reduce the volume of waste significantly. When waste is burned in a WTE plant, it can be reduced by up to 90% in volume and 75% in weight. This reduction in waste helps ease the pressure on landfills, which are becoming increasingly full in many parts of the world. Landfills are associated with several environmental issues, including land and water pollution, methane emissions (a potent greenhouse gas), and the long-term occupation of land that could be used for other purposes. By diverting waste from landfills, waste-to-energy plants help mitigate these environmental concerns.
Another benefit of waste-to-energy plants is that they provide a reliable and consistent source of energy. Unlike some renewable energy sources such as wind and solar, which can be intermittent depending on weather conditions, waste-to-energy plants can operate continuously, as long as there is a supply of waste to process. This makes them a stable addition to the energy grid, providing baseload power that can complement other renewable energy sources and help maintain a balanced energy supply. Moreover, waste-to-energy is considered carbon-neutral in many cases because the carbon dioxide released during the combustion process is roughly equivalent to the amount of carbon absorbed by the waste during its natural decomposition. As a result, it is viewed as a more sustainable option compared to burning fossil fuels.
In addition to reducing landfill waste and producing renewable energy, waste-to-energy plants can also recover valuable materials from the waste stream. Modern WTE plants often incorporate advanced sorting and recycling processes that extract metals, plastics, and other recyclable materials from the waste before it is incinerated. This helps further reduce the environmental footprint of waste disposal by recycling materials that can be reused in manufacturing. The ash produced by the incineration process can also be processed to remove heavy metals and other contaminants, with some of it being repurposed for use in construction materials or as fill for land reclamation projects.
Despite the many advantages, waste-to-energy plants face certain challenges. One of the primary concerns is the cost of building and operating these plants. Waste-to-energy facilities require significant investment in infrastructure, including incinerators, boilers, turbines, and emissions control systems. Operating and maintaining these plants also requires skilled labor and continuous monitoring to ensure compliance with environmental regulations. In some regions, the cost of electricity generated by waste-to-energy plants can be higher than that of electricity generated from conventional sources, making it less competitive economically. However, advances in technology and increasing demand for clean energy are gradually lowering these costs, making waste-to-energy more economically viable.
Environmental concerns related to the emissions from waste-to-energy plants are also an important issue. While modern facilities are equipped with technologies to reduce pollutants, the combustion of waste still releases pollutants such as nitrogen oxides, sulfur dioxide, and particulate matter. Moreover, certain waste materials can release toxic compounds, such as dioxins and furans, when burned. These compounds are highly harmful to human health and the environment. As a result, stringent regulations and monitoring systems are in place to ensure that emissions are kept within safe limits. Many countries also require waste-to-energy plants to implement best practices for waste sorting, reducing the amount of hazardous material that is burned.
Another challenge is the public perception of waste-to-energy technology. Some communities oppose the construction of waste-to-energy plants due to concerns over air pollution, health risks, and the potential for unpleasant odors. Public opposition can make it difficult to site new facilities, despite the environmental and energy benefits they offer. To address these concerns, proponents of waste-to-energy must demonstrate the safety and sustainability of these plants through transparent communication, environmental monitoring, and the use of advanced technologies that minimize emissions and odor.
Waste-to-energy technology is also linked to the broader issue of waste reduction and recycling. Critics of waste-to-energy argue that it could discourage efforts to reduce waste generation and increase recycling rates. If waste is viewed as a valuable resource for energy production, there may be less incentive for businesses and individuals to reduce their consumption, reuse materials, or recycle. However, many waste-to-energy plants emphasize the importance of waste reduction and recycling programs and focus on processing only non-recyclable waste. In some regions, waste-to-energy is seen as a complementary solution to recycling, not a replacement for it. The ideal waste management strategy involves reducing the amount of waste generated, recycling as much as possible, and using waste-to-energy for materials that cannot be recycled or reused.
Overall, waste-to-energy plants offer a promising solution to some of the pressing environmental challenges faced by modern society. They help address the growing volume of waste generated by urban populations, reduce the environmental impact of landfills, and provide a renewable source of energy. As technology continues to improve and environmental regulations become more stringent, waste-to-energy plants are likely to play an increasingly important role in sustainable waste management and renewable energy production. However, the successful implementation of waste-to-energy technology requires careful consideration of environmental impacts, community concerns, and economic viability to ensure that it remains a sustainable and effective solution in the long term.
Energy Recovery Systems with Steam Turbine
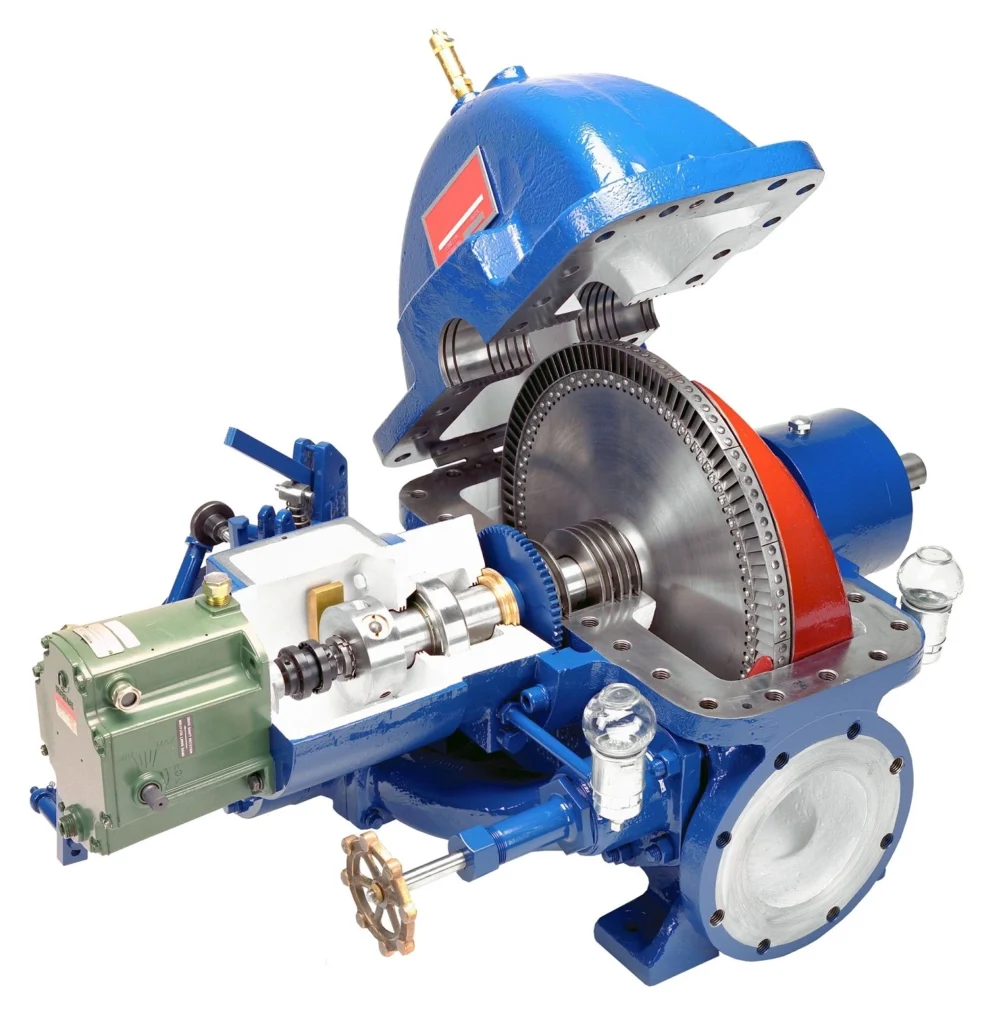
Energy recovery systems utilizing steam turbines represent a highly efficient method of capturing and converting waste heat from industrial processes, power plants, and other energy-intensive activities into usable power. These systems are an essential component of sustainable energy strategies, offering significant economic and environmental benefits. By leveraging the principles of thermodynamics, energy recovery systems with steam turbines transform heat energy—often lost in conventional systems—into electricity or mechanical work, reducing fuel consumption and greenhouse gas emissions. Their integration into various industrial settings has proven to be a game-changer, maximizing energy efficiency and contributing to a circular energy economy.
The core of such systems lies in the steam turbine, a device that converts the thermal energy of steam into mechanical energy, which is subsequently used to generate electricity. The process begins with the collection of waste heat, often produced as a byproduct in industrial applications such as chemical manufacturing, oil refining, and metallurgical processes. This waste heat is captured through heat exchangers, boilers, or other thermal devices, and used to produce high-pressure steam. The generated steam is directed into a steam turbine, where it expands, causing the turbine blades to rotate. This rotational motion drives a generator, producing electricity that can be used onsite or fed into the grid.
One of the primary advantages of energy recovery systems with steam turbines is their ability to utilize otherwise wasted energy. Industrial processes often operate at high temperatures, resulting in the release of significant amounts of heat into the environment. This waste heat, if not captured, represents a lost opportunity for energy production. By implementing a steam turbine system, industries can recover and repurpose this heat, effectively increasing the overall efficiency of their operations. This not only reduces the need for additional energy inputs but also lowers operational costs, making it a financially attractive option for energy-intensive sectors.
Steam turbines are highly versatile and can be designed to accommodate a wide range of operational conditions. Depending on the specific requirements of a given application, turbines can operate with varying steam pressures, temperatures, and flow rates. For instance, back-pressure steam turbines are commonly used in cogeneration systems, where the exhaust steam is utilized for heating or other industrial processes. Condensing steam turbines, on the other hand, are often employed in applications where maximizing electricity generation is the primary objective. This flexibility allows steam turbines to be tailored to the unique needs of different industries, ensuring optimal performance and energy recovery.
In addition to their operational adaptability, energy recovery systems with steam turbines are known for their reliability and long lifespan. Steam turbines are robust machines that can operate continuously under demanding conditions with minimal maintenance requirements. When properly designed and maintained, these systems can provide decades of efficient service, making them a durable and dependable investment. Their proven track record in industries such as power generation, pulp and paper, and petrochemical production underscores their reliability and effectiveness in real-world applications.
The environmental benefits of steam turbine-based energy recovery systems are equally compelling. By capturing and utilizing waste heat, these systems reduce the reliance on fossil fuels, which are typically burned to generate additional energy. This leads to a significant reduction in carbon dioxide and other greenhouse gas emissions, helping industries meet stringent environmental regulations and sustainability goals. Moreover, the reduction in fuel consumption translates into lower air pollutants, such as nitrogen oxides and sulfur dioxide, further minimizing the environmental impact of industrial operations. In the context of global efforts to combat climate change, energy recovery systems with steam turbines represent a practical and impactful solution.
Energy recovery systems with steam turbines are also highly scalable, making them suitable for a wide range of applications, from small-scale industrial plants to large power generation facilities. In smaller installations, these systems can provide on-site power generation, reducing dependence on external energy sources and enhancing energy security. In larger setups, they can be integrated into combined-cycle power plants, where they work in conjunction with gas turbines to maximize energy output. In these configurations, the waste heat from the gas turbine is used to produce steam, which then powers a steam turbine, resulting in exceptionally high overall efficiency.
The integration of steam turbines into energy recovery systems often involves significant upfront investment, as these systems require advanced engineering and specialized equipment. However, the long-term financial benefits, including reduced energy costs and lower environmental compliance expenses, typically outweigh the initial costs. Many governments and organizations also offer incentives, grants, or tax benefits for adopting energy recovery technologies, further enhancing their economic viability. These financial incentives, coupled with rising energy prices and stricter environmental regulations, are driving the adoption of steam turbine-based energy recovery systems across various sectors.
Technological advancements continue to enhance the efficiency and performance of steam turbines in energy recovery systems. Innovations such as advanced blade designs, improved materials, and more efficient heat exchange technologies have led to higher energy conversion rates and lower operational losses. Digital monitoring and control systems are also being integrated into steam turbine setups, allowing operators to optimize performance in real time. These advancements not only improve the overall efficiency of energy recovery systems but also extend their operational life and reduce maintenance costs.
In industrial applications, the versatility of steam turbines enables them to play a critical role in processes requiring both power and heat. For example, in the chemical and petrochemical industries, energy recovery systems can be integrated into processes like steam cracking and catalytic reforming, where large amounts of waste heat are generated. Similarly, in the steel and aluminum industries, where furnaces operate at extremely high temperatures, waste heat recovery through steam turbines can significantly enhance energy efficiency. These systems are also increasingly being employed in food processing, textiles, and other manufacturing sectors, where they contribute to both energy savings and improved environmental performance.
In conclusion, energy recovery systems with steam turbines represent a highly effective solution for addressing the dual challenges of energy efficiency and environmental sustainability. By harnessing waste heat from industrial processes and other sources, these systems reduce energy waste, lower greenhouse gas emissions, and provide a reliable source of renewable energy. Their adaptability, scalability, and proven reliability make them a valuable asset in a wide range of applications, from small industrial plants to large-scale power generation facilities. As technological advancements continue to improve their performance and reduce costs, steam turbine-based energy recovery systems are poised to play an increasingly vital role in the global transition to a more sustainable energy future.
The adoption of energy recovery systems with steam turbines is not just limited to traditional industrial settings or power plants; their potential extends into emerging sectors and innovative applications. For instance, these systems are gaining traction in renewable energy projects, where they can complement solar thermal and geothermal power generation. In solar thermal plants, where sunlight is concentrated to produce heat, steam turbines can efficiently convert the generated thermal energy into electricity. Similarly, in geothermal systems, the heat from underground reservoirs is used to produce steam, which powers turbines to generate electricity. These applications highlight the versatility of steam turbine technology in harnessing a variety of heat sources for energy production.
Another promising area for steam turbine-based energy recovery systems is the integration with district heating networks. In urban environments, waste heat from industrial processes or power plants can be recovered and distributed through a network of insulated pipes to provide heating and hot water for residential and commercial buildings. By incorporating steam turbines into these systems, it is possible to produce electricity alongside heat, further enhancing the energy efficiency and economic value of the setup. This approach, known as cogeneration or combined heat and power (CHP), is becoming increasingly popular in regions with high energy demand and a focus on sustainable urban development.
The potential of steam turbines in energy recovery also extends to transportation and maritime industries. In marine applications, for example, steam turbines are used to recover waste heat from ship engines, converting it into additional propulsion power or electricity for onboard systems. This not only improves the fuel efficiency of vessels but also reduces their environmental footprint, which is particularly important in light of stringent international regulations on maritime emissions. In land-based transportation, similar systems can be implemented in trains and large freight vehicles, where waste heat from engines can be harnessed for auxiliary power needs.
Moreover, the incorporation of energy recovery systems with steam turbines into waste-to-energy plants has further demonstrated their ability to address environmental challenges while generating energy. In these facilities, the heat produced from burning municipal solid waste is captured and used to produce steam, which powers turbines to generate electricity. This process not only reduces the volume of waste sent to landfills but also provides a renewable energy source, contributing to a circular economy. The adaptability of steam turbines to work with varying heat inputs makes them an ideal choice for waste-to-energy operations, where the quality and quantity of heat can fluctuate.
Industries are also exploring advanced cycles, such as the Organic Rankine Cycle (ORC), which allows energy recovery from lower-temperature waste heat sources that traditional steam turbines cannot efficiently utilize. In ORC systems, a working fluid with a lower boiling point than water is vaporized by the waste heat and drives a turbine, generating electricity. While these systems are distinct from conventional steam turbines, they are often integrated into broader energy recovery strategies, further expanding the range of recoverable heat sources. This integration demonstrates the ongoing innovation in utilizing steam turbines and related technologies to maximize energy recovery potential.
In the context of global energy transitions, energy recovery systems with steam turbines align with international goals to reduce carbon footprints and improve energy security. By making better use of existing energy resources, these systems help decrease reliance on non-renewable energy sources, mitigating the impacts of volatile fuel prices and geopolitical risks associated with fossil fuel supply chains. Furthermore, their contribution to reducing greenhouse gas emissions supports national commitments to international agreements such as the Paris Accord, where countries strive to limit global temperature increases through sustainable energy practices.
To ensure the widespread adoption of steam turbine-based energy recovery systems, ongoing efforts are needed to address key barriers such as initial capital costs and technological complexity. Educational initiatives, training programs, and the development of standard guidelines can help industries and stakeholders better understand the potential of these systems and how to implement them effectively. Financial incentives, such as tax credits, subsidies, or grants, can also play a pivotal role in encouraging investment and accelerating deployment. Collaboration between governments, industries, and research institutions is essential to overcome these challenges and unlock the full potential of steam turbine energy recovery systems.
Looking to the future, the role of digitalization and smart technologies is expected to transform the operation and management of energy recovery systems with steam turbines. Advanced sensors, data analytics, and predictive maintenance tools can optimize system performance, reduce downtime, and improve reliability. Digital twins—virtual replicas of physical systems—can simulate operational scenarios, enabling engineers to design more efficient systems and make informed decisions in real time. Such advancements not only enhance the economic and operational feasibility of steam turbine energy recovery systems but also contribute to the broader goals of Industry 4.0 and sustainable development.
In summary, energy recovery systems with steam turbines represent a cornerstone of sustainable energy strategies. Their ability to convert waste heat into valuable energy, reduce emissions, and enhance industrial efficiency positions them as a critical technology in addressing modern energy challenges. As industries and societies continue to prioritize sustainability, the role of these systems will only grow, driving innovation and paving the way for a cleaner, more energy-efficient future. Through continued technological advancements, supportive policies, and increased awareness, energy recovery systems with steam turbines are set to play a transformative role in shaping the global energy landscape.
Heat Recovery Steam Generator Design
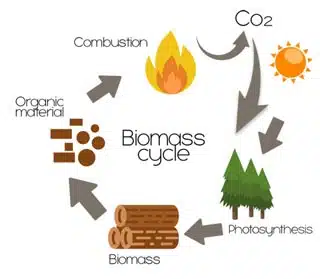
Heat Recovery Steam Generator (HRSG) design is a crucial aspect of energy systems that seek to maximize efficiency by recovering waste heat from high-temperature exhaust gases, typically from gas turbines or industrial processes, and using it to generate steam. This steam can then be utilized for power generation, heating, or industrial processes, depending on the specific application. The design of an HRSG is both a science and an art, requiring careful consideration of thermodynamic principles, material science, engineering mechanics, and operational requirements to create a system that optimally balances performance, cost, and durability.
At its core, HRSG design revolves around the transfer of heat from exhaust gases to water and steam in an efficient and controlled manner. The process begins with the exhaust gases, which enter the HRSG at high temperatures, often exceeding 500°C (932°F). These gases flow through a series of heat exchangers, including economizers, evaporators, and superheaters. Each component serves a specific function: the economizer preheats the water, the evaporator converts water to steam, and the superheater raises the steam temperature to desired levels. The design and arrangement of these components must be tailored to the specific characteristics of the exhaust gases and the operational requirements of the steam system.
One of the most critical aspects of HRSG design is the selection and configuration of heat transfer surfaces. Heat transfer efficiency is paramount, as it directly influences the overall performance of the HRSG. Finned tubes are often used to enhance the heat exchange process by increasing the surface area exposed to the exhaust gases. The arrangement of these tubes—whether in horizontal, vertical, or hybrid layouts—depends on factors such as space constraints, gas flow characteristics, and maintenance accessibility. Computational fluid dynamics (CFD) simulations are frequently employed during the design phase to optimize gas flow distribution and minimize pressure drop across the system.
Modularity is another key consideration in HRSG design. Modern HRSGs are often constructed in modular sections, allowing for greater flexibility and scalability. This modularity enables designers to customize the HRSG to meet the specific requirements of different gas turbines or process setups while maintaining a standardized approach to manufacturing and assembly. Additionally, modular designs facilitate easier transportation and installation, reducing the overall project timeline and costs.
The type of HRSG—whether single-pressure, dual-pressure, or triple-pressure—depends on the energy recovery goals and the steam demands of the application. Single-pressure HRSGs are simpler and more cost-effective, typically used in smaller installations or where the steam demand is limited. Dual- and triple-pressure designs, on the other hand, include multiple steam drums and pressure levels, allowing for the recovery of heat at varying temperature gradients. These multi-pressure systems are commonly used in combined-cycle power plants, where maximizing efficiency is a primary objective. In such setups, high-pressure steam is often sent to a steam turbine for electricity generation, while lower-pressure steam may be used for heating or industrial processes.
Another important aspect of HRSG design is the integration of supplementary firing systems. These systems allow for the injection of additional fuel into the exhaust gas stream, increasing the temperature and enhancing steam production. Supplementary firing is particularly beneficial in applications where fluctuating steam demands must be met or where the exhaust gases alone cannot provide sufficient thermal energy. The design of these systems must account for factors such as fuel type, combustion efficiency, and emissions control to ensure optimal performance and compliance with environmental regulations.
The materials used in HRSG construction play a critical role in ensuring reliability and longevity. High-temperature alloys and corrosion-resistant materials are often required for components exposed to the hottest sections of the exhaust gas stream, such as the superheaters and reheaters. In addition to withstanding high temperatures, these materials must resist thermal fatigue, oxidation, and other forms of degradation that can occur during prolonged operation. Proper selection and treatment of materials help prevent failures and reduce maintenance requirements, ensuring the HRSG operates efficiently over its intended lifespan.
Emissions control is another crucial element of HRSG design, especially in modern installations where strict environmental regulations are in place. HRSGs often incorporate systems such as selective catalytic reduction (SCR) units and oxidation catalysts to reduce nitrogen oxides (NOx) and carbon monoxide (CO) emissions, respectively. The placement and integration of these systems within the HRSG must be carefully designed to maintain efficiency while meeting regulatory standards. The positioning of catalysts and ammonia injection systems, for instance, requires precise engineering to achieve optimal chemical reactions without negatively impacting gas flow or heat transfer.
Operational flexibility is a growing focus in HRSG design, driven by the increasing integration of renewable energy sources into the power grid. As renewables like wind and solar introduce variability in power generation, HRSGs in combined-cycle plants must be able to adapt to fluctuating loads and frequent startups and shutdowns. This requires designs that minimize thermal stress during transient operations and enable rapid response times. Advanced control systems, robust materials, and innovative engineering solutions are being developed to address these challenges and ensure reliable performance under dynamic operating conditions.
Safety is a fundamental consideration in HRSG design. The high pressures and temperatures involved in steam generation pose inherent risks, making robust safety features a non-negotiable requirement. Components such as pressure relief valves, drum level controls, and temperature monitoring systems are integrated into the design to prevent overpressure, overheating, and other hazardous conditions. Regular inspections, rigorous testing, and adherence to industry standards are essential to maintaining a safe operating environment.
Lastly, economic factors play a significant role in HRSG design. While maximizing efficiency and performance is critical, the system must also be cost-effective to build, operate, and maintain. This requires a careful balance of upfront investment, operational costs, and expected returns. Advances in manufacturing techniques, material science, and digital technologies are helping to reduce costs and improve the overall value proposition of HRSG systems.
In summary, the design of a Heat Recovery Steam Generator is a complex and multifaceted process that integrates thermodynamics, material science, engineering, and economics. By recovering waste heat and converting it into valuable steam, HRSGs enhance energy efficiency, reduce emissions, and provide significant economic benefits across a wide range of applications. As energy systems continue to evolve, driven by technological innovation and the global push for sustainability, HRSG design will remain a critical area of development, enabling industries to meet the challenges of a rapidly changing energy landscape.
Advancements in technology are continually shaping the design and functionality of Heat Recovery Steam Generators (HRSGs), making them more efficient, versatile, and reliable. One of the most transformative trends is the integration of digital technologies and smart systems into HRSG operations. Advanced monitoring and control systems now enable real-time tracking of key parameters such as temperature, pressure, and gas flow, allowing operators to optimize performance and detect potential issues before they escalate. Predictive maintenance tools, powered by artificial intelligence and machine learning, can analyze historical data to predict failures, schedule maintenance proactively, and minimize downtime. These digital solutions are enhancing the reliability and lifespan of HRSGs, reducing operating costs, and maximizing energy recovery potential.
In addition to digitalization, innovations in materials science are driving significant improvements in HRSG design. New alloys and coatings are being developed to withstand higher temperatures and resist corrosion, allowing HRSGs to operate under more demanding conditions. These materials enable designers to push the boundaries of thermal efficiency, particularly in systems exposed to extremely high exhaust gas temperatures or aggressive chemical environments. Enhanced materials also contribute to longer operational lifespans, reducing the frequency and cost of component replacement.
Another area of progress in HRSG design is the development of compact and lightweight systems. Space constraints are often a major consideration in industrial facilities and power plants, particularly in retrofitting projects where existing infrastructure must be accommodated. Compact HRSG designs, featuring innovative tube arrangements and modular construction, address these challenges by minimizing the footprint and simplifying installation. Lightweight materials and efficient layouts further reduce structural support requirements, making it easier to integrate HRSGs into a variety of environments.
Energy storage and hybridization are emerging trends that are influencing HRSG design. In combined-cycle power plants, the incorporation of thermal energy storage systems can enhance the flexibility and efficiency of operations. By storing excess heat during periods of low demand and releasing it during peak times, these systems help balance energy supply and demand. Hybrid configurations, which combine HRSGs with other technologies such as battery storage or renewable energy sources, are also gaining traction. These setups enable more dynamic energy management, allowing facilities to adapt to changing grid requirements and renewable energy variability.
Environmental considerations continue to play a pivotal role in shaping HRSG design. As global efforts to reduce greenhouse gas emissions intensify, the focus on improving the environmental performance of HRSGs has increased. In addition to emissions control systems like selective catalytic reduction (SCR), innovative approaches such as carbon capture and utilization (CCU) are being explored. HRSGs integrated with carbon capture technologies can help facilities reduce their carbon footprint while generating valuable byproducts for industrial use. The design of these systems must carefully balance efficiency, cost, and environmental impact, highlighting the complexity of modern HRSG engineering.
The global shift towards renewable energy and decarbonization is also driving the adaptation of HRSGs for low-carbon fuels. In many regions, natural gas—a relatively clean-burning fossil fuel—remains the dominant source of energy for gas turbines feeding HRSGs. However, efforts are underway to transition to hydrogen and other alternative fuels. HRSGs designed to handle the unique combustion properties and exhaust characteristics of these fuels are being developed, ensuring that energy recovery systems remain relevant in a changing energy landscape. This requires adjustments in heat exchanger design, materials, and emissions control technologies to accommodate the new operational demands.
Flexibility is becoming increasingly critical in HRSG design as the energy industry moves toward more dynamic and decentralized systems. With the growth of distributed generation and renewable energy sources, HRSGs must be able to handle variable loads, frequent startups and shutdowns, and fluctuating exhaust gas temperatures. Advanced designs that minimize thermal stresses and improve ramp-up times are addressing these challenges, ensuring that HRSGs can operate effectively in modern energy systems. This adaptability makes HRSGs a key component in enabling the transition to a more flexible and resilient energy grid.
The role of HRSGs in industrial applications is also expanding. Beyond traditional power generation, HRSGs are being tailored for use in processes such as desalination, chemical production, and district heating. For example, in desalination plants, HRSGs can provide the steam needed for thermal desalination processes, reducing the reliance on standalone boilers and improving overall efficiency. Similarly, in district heating systems, HRSGs can supply both electricity and hot water, optimizing energy use and reducing emissions in urban environments. These diverse applications demonstrate the versatility of HRSGs and their ability to meet a wide range of energy needs.
Economic considerations remain central to HRSG design and deployment. While the initial capital costs of HRSG systems can be significant, the long-term savings in energy costs and emissions penalties often justify the investment. Developers and operators must carefully evaluate factors such as fuel savings, maintenance expenses, and regulatory incentives to determine the economic feasibility of HRSG projects. The growing availability of financing options, government subsidies, and tax incentives for energy efficiency projects is helping to lower the financial barriers to HRSG adoption.
Research and development efforts are continuously pushing the boundaries of what HRSGs can achieve. Collaboration between academia, industry, and government agencies is driving innovation in areas such as high-efficiency heat exchangers, advanced thermodynamic cycles, and novel energy recovery techniques. These efforts are not only improving the performance of HRSGs but also opening up new possibilities for their application in unconventional settings, such as offshore platforms, remote industrial sites, and even spacecraft thermal management systems.
In conclusion, the design of Heat Recovery Steam Generators is a dynamic and evolving field that combines cutting-edge technology, engineering expertise, and a deep understanding of thermodynamics. As energy systems transition towards greater efficiency, sustainability, and flexibility, HRSGs will continue to play a vital role in capturing waste heat and converting it into valuable energy. With advancements in digitalization, materials science, and hybrid energy systems, HRSG design is poised to address the challenges of the future, enabling industries and power plants to achieve their energy efficiency and environmental goals. The ongoing development and refinement of HRSG technology ensure that these systems will remain a cornerstone of modern energy infrastructure for decades to come.
Waste Heat Recovery Technologies and Applications
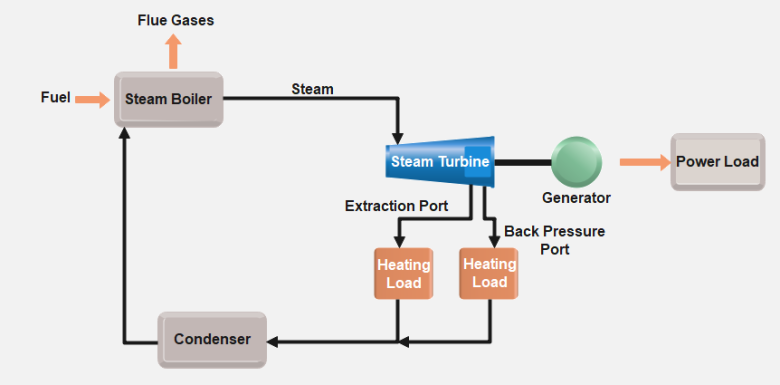
Waste heat recovery technologies and applications are at the forefront of global efforts to improve energy efficiency and sustainability in industrial and commercial settings. These technologies aim to capture and utilize the heat that is typically lost in industrial processes, power generation, and transportation systems, transforming it into a valuable energy source. By doing so, waste heat recovery not only reduces energy consumption and greenhouse gas emissions but also lowers operational costs, making it a critical component of modern energy systems.
At the heart of waste heat recovery lies the principle of utilizing thermal energy that would otherwise be discarded into the environment. Industrial processes, such as metal smelting, glass manufacturing, cement production, and refining, generate vast amounts of heat that often escape through exhaust gases, cooling water, or other waste streams. Similarly, power plants and engines, whether in vehicles or stationary applications, release significant quantities of heat during operation. Capturing and repurposing this heat can significantly improve the overall energy efficiency of these systems, offering both economic and environmental benefits.
A wide range of technologies is available for waste heat recovery, each tailored to specific temperature ranges, heat sources, and applications. Heat exchangers, for instance, are among the most commonly used technologies, transferring heat from a hot medium, such as exhaust gases, to a cooler medium, such as water or air. These devices come in various designs, including shell-and-tube, plate, and air-to-air configurations, allowing for flexibility in adapting to different industrial processes and spatial constraints.
Thermal storage systems are another critical component of waste heat recovery strategies. These systems capture excess heat during periods of high activity and store it for later use, smoothing out fluctuations in energy supply and demand. Phase-change materials, molten salts, and other advanced storage media are increasingly being employed in these systems to enhance their efficiency and storage capacity. This capability is particularly valuable in facilities where energy demand varies throughout the day, as it allows for greater operational flexibility and cost savings.
Organic Rankine Cycle (ORC) systems represent a cutting-edge approach to waste heat recovery, particularly for low- and medium-temperature heat sources. In ORC systems, an organic working fluid with a low boiling point is used instead of water, enabling the recovery of heat from sources that traditional steam-based systems cannot efficiently utilize. These systems are increasingly being adopted in industries such as geothermal energy, biomass power generation, and small-scale industrial plants, where waste heat temperatures are typically below 300°C (572°F).
Another innovative waste heat recovery technology is thermoelectric generation, which leverages the Seebeck effect to directly convert heat into electricity. Thermoelectric modules are solid-state devices with no moving parts, making them highly reliable and maintenance-free. While their efficiency is currently lower than that of other technologies, ongoing research and development are improving their performance and making them suitable for niche applications, such as in automotive exhaust systems and remote power generation.
Applications of waste heat recovery technologies span a broad spectrum of industries and sectors. In industrial manufacturing, waste heat recovery is used to preheat combustion air, process feedstocks, or water, reducing the energy required for these operations. For example, in steel and aluminum production, waste heat from furnaces can be captured and used to generate steam, which in turn powers turbines to produce electricity or provides process heat for downstream operations. In cement plants, waste heat recovery systems are integrated into the kiln exhaust and clinker cooling processes to generate electricity, offsetting the plant’s overall energy consumption.
In power generation, combined-cycle plants are a prime example of waste heat recovery in action. These plants combine gas turbines with heat recovery steam generators (HRSGs) to capture and convert the exhaust heat from gas turbines into steam, which drives additional turbines to generate more electricity. This process significantly boosts the efficiency of power generation, often achieving thermal efficiencies of 50% to 60% or higher compared to the 30% to 40% typical of single-cycle plants.
The transportation sector also benefits from waste heat recovery technologies. In automotive applications, for example, systems such as turbochargers and thermoelectric generators capture heat from engine exhaust to improve fuel efficiency or generate electricity for onboard systems. Similarly, in marine and aviation applications, waste heat recovery systems can be used to supplement propulsion power or provide electricity for auxiliary systems, reducing fuel consumption and emissions.
Waste-to-energy (WTE) plants represent another critical application of waste heat recovery technologies. In these facilities, municipal solid waste is incinerated to generate heat, which is then captured to produce steam for electricity generation or district heating. By recovering heat from waste combustion, WTE plants not only reduce the volume of waste sent to landfills but also provide a renewable energy source, contributing to a circular economy.
In commercial and residential buildings, waste heat recovery systems are employed in heating, ventilation, and air conditioning (HVAC) systems to improve energy efficiency. For example, heat recovery ventilators (HRVs) and energy recovery ventilators (ERVs) capture heat from outgoing exhaust air and transfer it to incoming fresh air, reducing the energy required for heating or cooling. Similarly, heat pump systems can recover waste heat from refrigeration or air conditioning units and use it for space or water heating.
District energy systems are another area where waste heat recovery technologies play a vital role. These systems distribute recovered heat through a network of insulated pipes to provide heating and cooling to multiple buildings in urban or industrial areas. By centralizing energy production and utilizing waste heat from industrial facilities, power plants, or even data centers, district energy systems can achieve higher overall efficiency and lower emissions than individual heating and cooling systems.
Despite their numerous benefits, the widespread adoption of waste heat recovery technologies faces several challenges. The initial capital cost of implementing these systems can be high, particularly for retrofitting existing facilities. Technical challenges, such as integrating waste heat recovery systems with existing processes or managing variable heat sources, also need to be addressed. Additionally, the economic feasibility of waste heat recovery depends on factors such as the availability and quality of the waste heat source, the cost of energy, and the presence of regulatory incentives or carbon pricing mechanisms.
To overcome these challenges, ongoing research and development are focused on improving the performance, cost-effectiveness, and versatility of waste heat recovery technologies. Advances in materials science, thermodynamics, and system design are enabling more efficient and compact solutions, while digital tools such as simulation software and data analytics are helping optimize system integration and operation. Policy support, including subsidies, tax incentives, and regulations promoting energy efficiency, is also crucial in driving the adoption of waste heat recovery technologies.
In conclusion, waste heat recovery technologies and applications are essential for improving energy efficiency, reducing emissions, and lowering costs across a wide range of industries and sectors. By capturing and repurposing heat that would otherwise be wasted, these technologies provide a sustainable and economically viable solution to modern energy challenges. As innovation continues and awareness of the benefits of waste heat recovery grows, these systems are set to play an increasingly important role in global energy strategies, contributing to a cleaner, more sustainable future.
The role of waste heat recovery technologies is becoming even more critical as global energy demands increase and efforts to mitigate climate change intensify. Governments, industries, and research institutions are placing greater emphasis on optimizing energy use to reduce dependency on fossil fuels and curb greenhouse gas emissions. Waste heat recovery offers a practical and immediate pathway to achieving these goals by maximizing the efficiency of existing systems without requiring significant new energy inputs.
One of the key areas of focus in advancing waste heat recovery technologies is the development of systems capable of utilizing low-grade heat sources. Traditionally, waste heat recovery has been most effective for high-temperature heat streams, such as those found in furnaces, kilns, and power plants. However, a significant portion of waste heat is released at lower temperatures, often below 150°C (302°F), making it difficult to capture and use efficiently. Innovations in materials and systems design are overcoming these barriers. For instance, advanced thermoelectric materials, absorption chillers, and low-temperature ORC systems are being developed to harness low-grade heat for electricity generation, cooling, or process heat.
Micro- and small-scale waste heat recovery systems are also gaining attention, particularly in distributed energy applications. Small-scale solutions enable individual facilities or even residential buildings to recover waste heat, providing localized energy efficiency benefits. Technologies such as compact heat exchangers, micro-ORC systems, and modular heat recovery units are designed to be easily integrated into smaller-scale systems, making them accessible for a broader range of applications. This decentralization of waste heat recovery can complement larger centralized systems, creating a more robust and distributed energy network.
Another area of exploration is the use of waste heat in synergistic applications that combine multiple energy functions. For example, in cogeneration or combined heat and power (CHP) systems, waste heat is simultaneously used for electricity generation and heating. In more advanced configurations, these systems can be extended to include cooling through technologies like absorption chillers, creating a combined cooling, heating, and power (CCHP) system. Such integrated solutions maximize the utilization of waste heat, achieving efficiencies that are difficult to match with standalone systems.
The integration of waste heat recovery technologies with renewable energy systems presents another promising frontier. Renewable energy sources like solar, wind, and geothermal often face variability and intermittency challenges, which can be partially mitigated through waste heat recovery. For instance, hybrid systems that combine solar thermal energy with waste heat recovery can enhance overall energy output and reliability. Similarly, waste heat recovery can be used to support the operation of biomass and biogas power plants, improving their economic and environmental performance.
Emerging industrial processes and technologies are also creating new opportunities for waste heat recovery. For example, the rise of electric arc furnaces (EAFs) in steel production, which operate at high temperatures, presents a significant source of recoverable heat. Similarly, advanced manufacturing techniques like additive manufacturing (3D printing) and chemical recycling processes generate localized heat that can be captured and repurposed. Adapting waste heat recovery systems to these new processes ensures that energy efficiency keeps pace with technological innovation.
The environmental benefits of waste heat recovery extend beyond energy efficiency and emissions reductions. By lowering energy consumption, waste heat recovery reduces the demand for fuel extraction, transportation, and processing, mitigating the environmental impact of these activities. Furthermore, in industries like waste-to-energy, integrating waste heat recovery helps reduce the release of pollutants by ensuring more complete combustion and energy extraction. This aligns with broader sustainability goals and supports compliance with increasingly stringent environmental regulations.
Urban environments are emerging as a unique setting for waste heat recovery applications. Urban waste heat sources, such as subway systems, data centers, and commercial buildings, represent an untapped resource for district heating and cooling networks. For example, waste heat from server farms and IT infrastructure, which operate continuously and generate significant heat loads, can be captured and used to provide heating or hot water for nearby residential or commercial buildings. This not only reduces energy consumption but also addresses the growing demand for sustainable energy solutions in densely populated areas.
The role of digital technologies in optimizing waste heat recovery systems cannot be overstated. Advanced sensors, data analytics, and control systems enable precise monitoring and management of heat recovery processes, ensuring maximum efficiency and reliability. Digital twins, which create virtual replicas of physical systems, allow operators to simulate and optimize waste heat recovery performance under various scenarios, reducing operational risks and improving decision-making. The integration of these digital tools with waste heat recovery systems represents a significant step forward in harnessing the full potential of this technology.
Economic drivers for waste heat recovery continue to strengthen as energy prices rise and carbon pricing mechanisms become more widespread. The financial savings from reduced energy consumption, combined with potential revenue from generating additional electricity or heat, make waste heat recovery an attractive investment for industries and facilities of all sizes. In many cases, government incentives, subsidies, and grants further improve the economic case for adopting these technologies. For instance, tax credits for energy efficiency projects or funding for renewable energy initiatives often include provisions for waste heat recovery systems, lowering the barrier to entry for businesses.
Research and development efforts are crucial to advancing waste heat recovery technologies and expanding their applications. Collaborative projects involving academia, industry, and government agencies are driving innovation in areas such as high-efficiency heat exchangers, advanced thermodynamic cycles, and novel materials for heat capture and transfer. These efforts are not only improving the technical performance of waste heat recovery systems but also reducing their costs, making them accessible to a broader range of users.
In conclusion, waste heat recovery technologies and applications are indispensable in the quest for energy efficiency, sustainability, and economic viability. By capturing and repurposing thermal energy that would otherwise be lost, these technologies provide a practical and scalable solution to modern energy challenges. As innovations continue and new opportunities emerge, waste heat recovery is poised to play an increasingly central role in global energy systems, contributing to a cleaner, more efficient, and sustainable future.
Waste Heat Recovery System in Ships
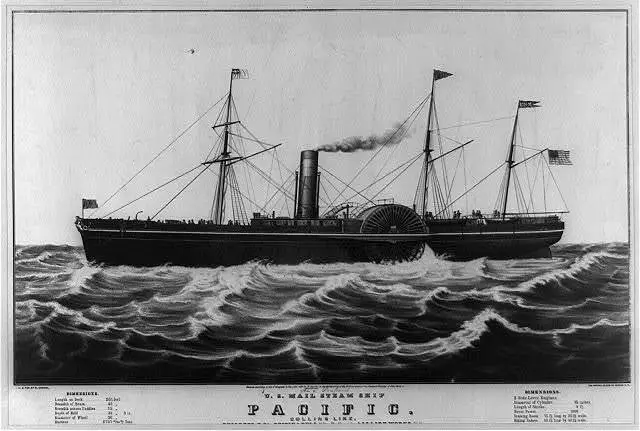
Waste heat recovery systems in ships play a critical role in enhancing energy efficiency, reducing fuel consumption, and minimizing environmental impact in maritime operations. The fundamental principle of these systems is to capture the thermal energy that would otherwise be wasted in the form of exhaust gases, cooling water, or other heat streams generated by marine engines and auxiliary machinery. This recovered heat is then repurposed for various on-board applications, such as generating additional power, heating, or even supporting propulsion systems.
Marine vessels, particularly those powered by large internal combustion engines like two-stroke and four-stroke diesel engines, are significant producers of waste heat. These engines operate at high temperatures and release substantial amounts of energy through exhaust gases, which can constitute up to 50% of the total energy produced during combustion. Instead of allowing this energy to dissipate into the atmosphere, waste heat recovery systems capture it to improve the overall efficiency of the ship’s energy systems.
A typical waste heat recovery system in a ship integrates various components, including exhaust gas boilers or economizers, steam turbines, and heat exchangers. The exhaust gas boiler is a critical component that harnesses heat from the engine’s exhaust gases to produce steam. This steam can then be used to drive a steam turbine generator for additional electricity generation or provide heat for other purposes, such as desalination, heating fuel oil, or maintaining living spaces on board. This multi-functional use of recovered heat significantly reduces the dependency on fuel oil for auxiliary boilers, leading to substantial cost savings and lower emissions.
One of the primary advantages of waste heat recovery systems in ships is their ability to enhance fuel efficiency. By utilizing waste heat to generate auxiliary power, ships can reduce their reliance on conventional fuel-burning generators, thereby cutting fuel consumption. This reduction is particularly important for large vessels such as tankers, container ships, and cruise liners, where operational costs and environmental compliance are critical considerations. Improved fuel efficiency also translates to extended voyage ranges and reduced operational costs, which are vital in competitive shipping markets.
Another significant benefit of waste heat recovery in ships is the reduction of greenhouse gas emissions, particularly carbon dioxide (CO2), sulfur oxides (SOx), and nitrogen oxides (NOx). By capturing and reusing heat that would otherwise contribute to thermal pollution, ships can operate more sustainably and meet stringent international regulations such as those imposed by the International Maritime Organization (IMO). For instance, IMO’s Energy Efficiency Design Index (EEDI) and Energy Efficiency Existing Ship Index (EEXI) encourage the adoption of technologies like waste heat recovery to meet efficiency and emissions targets.
In addition to exhaust gases, waste heat recovery systems in ships can utilize other heat sources, such as cooling water from engine jackets or lubricating oil systems. Engine cooling water absorbs heat during operation and can be directed through heat exchangers to preheat fuel oil, power absorption chillers, or support other thermal processes on board. Similarly, heat from lubricating oil systems can be recovered to improve the efficiency of auxiliary systems, further enhancing energy utilization.
The integration of waste heat recovery systems with other energy-efficient technologies is also gaining traction in the maritime industry. For example, combining waste heat recovery with hybrid propulsion systems or battery storage can create more resilient and flexible energy management solutions. Recovered heat can be used to charge batteries or supplement energy supply during peak demand periods, ensuring smooth and efficient operations. In some cases, waste heat recovery systems are integrated with renewable energy technologies like solar panels or wind-assisted propulsion, creating synergistic benefits and further reducing the environmental footprint of ships.
Despite their numerous advantages, waste heat recovery systems in ships face several challenges. One of the primary challenges is the variability of waste heat availability, which depends on the engine’s operating conditions and load. For instance, during low-load operation, the temperature and flow rate of exhaust gases may not be sufficient to generate significant amounts of steam or energy. To address this, advanced system designs incorporate thermal storage solutions or hybrid configurations that maintain efficiency under varying conditions.
The initial cost of installing waste heat recovery systems on ships can also be a barrier, particularly for older vessels undergoing retrofitting. These systems require careful integration with existing engine and auxiliary systems, which can involve significant engineering and installation costs. However, the long-term fuel savings, regulatory compliance benefits, and reduced emissions often justify the investment. Moreover, many shipping companies are exploring financing options and government incentives to offset the upfront costs of adopting these technologies.
Another challenge is the maintenance and durability of waste heat recovery systems in the harsh marine environment. Components such as heat exchangers, economizers, and steam turbines are exposed to high temperatures, corrosive gases, and mechanical stresses, requiring robust materials and regular maintenance to ensure reliability and longevity. Advances in materials science, such as corrosion-resistant alloys and coatings, are addressing these issues and improving the performance of waste heat recovery systems in marine applications.
Waste heat recovery systems are also playing a vital role in the transition toward greener maritime operations. As the industry moves toward decarbonization, technologies like waste heat recovery are being incorporated into ship designs to complement alternative fuels such as LNG, hydrogen, and ammonia. These systems not only enhance the energy efficiency of ships using conventional fuels but also improve the viability of alternative fuel systems by recovering heat from their specific combustion or reforming processes.
The applications of waste heat recovery in ships extend beyond energy efficiency and emissions reduction. For instance, in cruise ships, recovered heat can be used to power absorption chillers for air conditioning systems, enhancing passenger comfort while reducing energy consumption. In cargo vessels, recovered heat can support refrigeration systems or provide thermal energy for cargo handling processes, such as heating crude oil or maintaining the temperature of liquefied gases.
In conclusion, waste heat recovery systems in ships are a cornerstone of modern energy management in the maritime industry. By capturing and reusing thermal energy from engines and auxiliary systems, these technologies improve fuel efficiency, reduce emissions, and enhance operational sustainability. As the industry faces increasing regulatory pressures and environmental challenges, waste heat recovery systems are set to play an even more prominent role in shaping the future of maritime energy systems. With ongoing advancements in technology, materials, and integration strategies, these systems will continue to drive progress toward cleaner, more efficient, and environmentally responsible shipping practices.
The continued evolution of waste heat recovery systems in ships is driven by both technological advancements and the growing need for compliance with global environmental regulations. Research and development efforts are focused on increasing the efficiency, adaptability, and reliability of these systems to meet the diverse operational requirements of modern ships while reducing their environmental footprint. As shipping companies and vessel designers aim for more sustainable solutions, waste heat recovery systems are becoming integral to next-generation ship designs and retrofitting projects.
One area of significant innovation is the development of advanced heat exchanger designs tailored for maritime applications. Compact and highly efficient plate heat exchangers, for example, are being employed to recover heat from engine jacket water, lubricating oil, and other thermal sources. These designs optimize heat transfer while minimizing space and weight, which are critical considerations on ships where every square meter of space is valuable. Advances in materials, such as corrosion-resistant alloys and nanostructured coatings, further enhance the durability and performance of heat exchangers in the demanding marine environment.
Automation and digitalization are also transforming waste heat recovery systems in ships. Advanced monitoring and control systems equipped with sensors and data analytics enable real-time optimization of waste heat utilization. By continuously adjusting system parameters to match engine operating conditions, these technologies maximize efficiency and ensure stable performance under variable loads. Integration with ship-wide energy management systems allows for coordinated operation of waste heat recovery, propulsion, and auxiliary systems, further improving overall energy efficiency.
Energy storage solutions are another area of exploration in waste heat recovery for ships. Thermal energy storage systems, such as phase change materials (PCMs) or molten salts, can store excess heat recovered during high-load operation and release it during periods of low demand. This capability ensures a consistent supply of thermal energy, even when engine performance fluctuates or when the ship is idling. These systems not only enhance the reliability of waste heat recovery but also enable new applications, such as supporting hybrid propulsion systems or providing energy during port operations.
The integration of waste heat recovery with alternative fuel systems is creating new synergies in maritime energy management. For example, ships powered by liquefied natural gas (LNG) or hydrogen often produce heat during fuel combustion or reforming processes that can be captured and reused. Similarly, vessels equipped with fuel cells, which are gaining popularity for their low emissions, generate waste heat that can be recovered to power auxiliary systems or contribute to propulsion. These integrated systems maximize the energy potential of alternative fuels and accelerate the transition to sustainable shipping.
Innovations in power generation technologies are also enhancing the capabilities of waste heat recovery systems. Organic Rankine Cycle (ORC) systems, which use low-boiling-point working fluids to generate power from low-grade heat, are being increasingly adopted on ships. ORC systems are particularly well-suited for capturing heat from medium-temperature sources, such as engine exhaust gases, and converting it into electricity with high efficiency. Their compact size, low maintenance requirements, and ability to operate in variable conditions make them ideal for maritime applications.
The environmental benefits of waste heat recovery systems extend beyond direct emissions reductions. By improving fuel efficiency and reducing the reliance on auxiliary generators, these systems lower the demand for fuel bunkering and the associated logistical and environmental costs. Additionally, by minimizing heat dissipation into the atmosphere or ocean, waste heat recovery systems help mitigate thermal pollution, which can adversely affect marine ecosystems and local environments near ports.
Policy incentives and regulatory frameworks are playing a crucial role in encouraging the adoption of waste heat recovery systems in the shipping industry. International organizations like the IMO have established ambitious targets for reducing greenhouse gas emissions from ships, including a 50% reduction in total emissions by 2050 compared to 2008 levels. To achieve these goals, waste heat recovery is recognized as a key energy efficiency measure. Many countries and ports are also offering incentives, such as tax breaks or reduced port fees, for ships equipped with energy-efficient technologies, further driving adoption.
As waste heat recovery systems become more advanced and accessible, they are increasingly being implemented across a broader range of vessel types. While large commercial ships like tankers and container vessels have traditionally been the primary adopters, smaller vessels, including ferries, fishing boats, and offshore support ships, are also beginning to incorporate these systems. This trend reflects the growing recognition of the economic and environmental benefits of waste heat recovery, regardless of ship size or operational profile.
Looking ahead, the role of waste heat recovery systems in ships is expected to expand further with the advent of smart and autonomous shipping technologies. Autonomous ships, which rely on sophisticated energy management systems to operate efficiently and safely, will benefit significantly from the integration of waste heat recovery. These systems will not only enhance the energy efficiency of autonomous operations but also provide a sustainable energy source for the advanced electronics and sensors required for navigation and control.
In summary, waste heat recovery systems are a cornerstone of energy efficiency and sustainability in the maritime industry. By capturing and reusing thermal energy from engines and auxiliary systems, these technologies enhance fuel efficiency, reduce emissions, and improve the overall environmental performance of ships. With ongoing advancements in technology, materials, and integration strategies, waste heat recovery systems are poised to play an increasingly central role in the future of shipping, contributing to cleaner, more efficient, and sustainable maritime operations.
Waste Heat Recovery System in Cement Plant
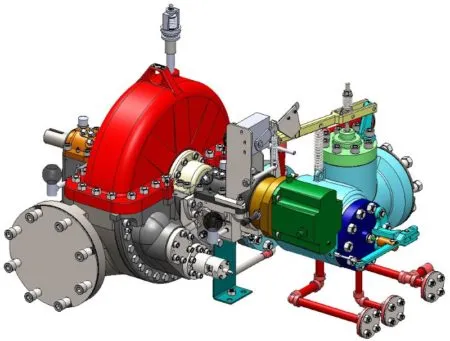
Waste heat recovery systems in cement plants are pivotal in improving energy efficiency, reducing greenhouse gas emissions, and lowering production costs. The cement manufacturing process is highly energy-intensive, with a significant portion of the energy consumed being lost as waste heat through exhaust gases, kiln surfaces, and other sources. Waste heat recovery (WHR) systems capture this otherwise unused thermal energy and convert it into usable power or heat, contributing to a more sustainable and efficient production process.
Cement plants generate waste heat primarily during the clinker production phase, which involves the calcination of raw materials at high temperatures in rotary kilns. The exhaust gases released from the kiln preheater and clinker cooler are the primary sources of waste heat, often accounting for more than 30% of the total energy input. These gases typically leave the system at temperatures ranging from 250°C to 400°C (482°F to 752°F), offering significant potential for energy recovery.
A waste heat recovery system in a cement plant typically includes heat exchangers, waste heat boilers, and power generation equipment, such as steam turbines or organic Rankine cycle (ORC) systems. Heat exchangers capture thermal energy from the high-temperature exhaust gases, transferring it to a working fluid such as water or an organic compound. The heated fluid then drives turbines to generate electricity, which can be used to power plant operations, reducing dependency on external electricity sources. In some cases, the recovered heat is also used directly for other processes within the plant, such as drying raw materials or fuels.
The integration of WHR systems into cement plants brings multiple benefits. One of the most significant advantages is the reduction in energy costs. Cement production requires substantial amounts of electricity and thermal energy, and by utilizing waste heat to generate power, plants can decrease their reliance on purchased electricity. This not only reduces operational expenses but also shields the plant from fluctuations in energy prices, enhancing economic stability and competitiveness.
In addition to cost savings, WHR systems significantly reduce the environmental impact of cement manufacturing. By capturing and reusing waste heat, these systems lower the overall energy consumption of the plant, leading to a corresponding reduction in greenhouse gas emissions. This is particularly important for the cement industry, which is one of the largest industrial sources of CO₂ emissions worldwide. WHR systems also contribute to reducing other pollutants, such as nitrogen oxides (NOx) and sulfur oxides (SOx), by minimizing the need for additional fossil fuel combustion.
Technological advancements have expanded the potential of WHR systems in cement plants, making them more efficient and adaptable to various operating conditions. For instance, ORC systems have gained popularity for their ability to efficiently utilize low-grade heat sources, such as those found in cement plant exhaust gases. Unlike traditional steam Rankine cycle systems, ORC systems use organic working fluids with lower boiling points, enabling them to recover heat from lower-temperature sources. This flexibility makes ORC systems particularly suitable for smaller or older cement plants, where high-temperature waste heat streams may not be readily available.
The implementation of WHR systems in cement plants is also driven by regulatory pressures and sustainability goals. Governments and environmental organizations are increasingly mandating energy efficiency measures and carbon reduction strategies for industrial sectors. WHR systems align with these objectives by enabling plants to achieve substantial energy savings and reduce emissions without compromising production capacity. In many cases, the installation of WHR systems can also qualify for financial incentives, such as tax credits, grants, or subsidies, which further enhance their economic feasibility.
The adoption of WHR systems in cement plants is not without challenges. One of the primary barriers is the high initial investment required for system installation and integration. The capital costs can be significant, especially for retrofitting existing plants with WHR technology. However, the long-term energy savings and emissions reductions often offset these costs, with many systems achieving payback periods of three to five years. Additionally, advancements in technology and economies of scale are gradually reducing the upfront costs of WHR systems, making them more accessible to a wider range of cement manufacturers.
Another challenge is the variability of waste heat availability, which depends on the operational characteristics of the cement plant. For instance, the temperature and flow rate of exhaust gases can fluctuate based on production levels, raw material composition, and kiln operation. To address these issues, modern WHR systems are designed with flexible and adaptive features, such as variable-speed turbines and modular heat exchangers, that optimize performance under varying conditions.
Maintenance and reliability are critical considerations for WHR systems in cement plants. The harsh operating environment, characterized by high temperatures, abrasive particles, and corrosive gases, can lead to wear and tear on system components. Regular maintenance and the use of durable materials, such as corrosion-resistant alloys and advanced coatings, are essential to ensure the longevity and efficiency of the system. Advances in monitoring and diagnostics, including the use of sensors and predictive analytics, are also improving the reliability of WHR systems by enabling early detection and resolution of potential issues.
The role of digitalization in optimizing WHR systems in cement plants is becoming increasingly important. Advanced control systems and data analytics enable real-time monitoring and management of heat recovery processes, ensuring maximum energy efficiency and reliability. Digital twins, which create virtual models of physical systems, allow operators to simulate and optimize WHR system performance under different scenarios, improving decision-making and reducing operational risks.
In conclusion, waste heat recovery systems are a vital component of energy efficiency and sustainability strategies in cement plants. By capturing and repurposing thermal energy from exhaust gases and other sources, these systems reduce energy consumption, lower emissions, and enhance economic competitiveness. As technology continues to evolve, WHR systems are becoming more efficient, adaptable, and cost-effective, making them an increasingly attractive option for cement manufacturers worldwide. With the industry’s growing focus on sustainability and environmental responsibility, waste heat recovery will play a central role in shaping the future of cement production, driving progress toward cleaner, more efficient, and sustainable operations.
The future of waste heat recovery systems in cement plants is strongly tied to global trends in energy efficiency, decarbonization, and circular economy initiatives. As the cement industry faces increasing scrutiny over its environmental impact, waste heat recovery (WHR) technology is evolving to meet more stringent requirements while supporting innovation in energy systems integration and resource optimization. Emerging advancements and strategic approaches are likely to expand the adoption of WHR systems, making them an indispensable part of modern cement manufacturing.
One significant area of focus is the integration of WHR systems with renewable energy sources. Cement plants that harness solar, wind, or biomass energy as part of their operations can use WHR systems to complement and stabilize their energy supply. For instance, when renewable energy output fluctuates due to weather or seasonal conditions, the consistent energy recovered from waste heat can provide a reliable backup, ensuring uninterrupted plant operations. This hybrid approach enhances energy security and aligns with broader sustainability goals, helping plants achieve near-zero carbon emissions.
Additionally, WHR systems are being explored as a means to support carbon capture, utilization, and storage (CCUS) technologies in cement plants. Since cement manufacturing is one of the largest sources of industrial carbon dioxide emissions, CCUS is emerging as a critical pathway to mitigate its environmental footprint. WHR systems can supply the thermal energy required for some carbon capture processes, such as solvent regeneration in post-combustion capture systems. This synergy reduces the energy penalty associated with carbon capture, making it more feasible for large-scale deployment in the cement sector.
On a technological level, the efficiency of WHR systems is being enhanced through advanced thermodynamic cycles and materials science innovations. For example, supercritical carbon dioxide (sCO₂) power cycles are being investigated as an alternative to conventional steam or organic Rankine cycles. sCO₂ cycles operate at higher efficiencies and can extract more energy from the same heat source, particularly in medium- to high-temperature ranges, making them ideal for cement plant applications. Similarly, advanced heat exchanger designs, such as compact heat exchangers with microchannel structures, offer improved heat transfer performance while reducing system size and weight.
Energy storage integration is also emerging as a transformative opportunity for WHR systems in cement plants. By incorporating thermal energy storage solutions, plants can store excess heat generated during peak operation periods and utilize it during times of lower production. This capability not only enhances operational flexibility but also supports load leveling for power grids, particularly in regions with high renewable energy penetration. Innovations such as phase change materials (PCMs) and thermochemical storage systems are making energy storage more efficient and cost-effective, further extending the utility of WHR systems.
Economic incentives and policy frameworks are playing an essential role in driving the adoption of WHR systems in cement plants. Governments and international organizations are increasingly offering financial incentives, such as subsidies, tax credits, and low-interest loans, to encourage the adoption of energy-efficient technologies. Additionally, carbon pricing mechanisms, such as carbon taxes or emissions trading schemes, make it economically advantageous for cement manufacturers to invest in WHR systems to reduce their carbon footprint. These policy measures not only accelerate the implementation of WHR technology but also create a favorable environment for innovation and technological advancement.
Beyond environmental and economic benefits, WHR systems contribute to enhancing the resilience and competitiveness of cement plants. In an era of rising energy costs and supply chain disruptions, the ability to generate on-site power from waste heat provides a strategic advantage. By reducing dependency on external electricity providers, cement plants equipped with WHR systems can mitigate risks associated with energy price volatility and supply interruptions, ensuring stable and cost-effective operations.
Global collaboration and knowledge sharing are critical to overcoming the challenges associated with implementing WHR systems in cement plants, particularly in developing regions where the cement industry is rapidly expanding. Initiatives by international organizations, such as the Global Cement and Concrete Association (GCCA) and the International Energy Agency (IEA), aim to promote best practices, provide technical guidance, and facilitate access to funding for WHR projects. These efforts are vital for scaling up the adoption of WHR systems and achieving global energy efficiency and emissions reduction targets.
As WHR systems become more advanced and widespread, they are increasingly being tailored to the specific needs and characteristics of individual cement plants. Customized solutions that take into account factors such as plant size, production capacity, local climate conditions, and waste heat availability are enabling higher levels of efficiency and performance. For example, modular WHR systems can be designed to accommodate incremental capacity expansions or phased implementation, allowing plants to adopt the technology progressively based on their operational and financial constraints.
The role of digitalization and smart technologies in optimizing WHR systems cannot be overstated. Advanced monitoring and control systems equipped with real-time data analytics, artificial intelligence (AI), and machine learning algorithms are enabling plant operators to maximize the efficiency of heat recovery processes. Predictive maintenance, powered by sensors and IoT devices, ensures system reliability by identifying potential issues before they escalate, reducing downtime and maintenance costs. Digital twins, which provide virtual simulations of physical systems, are also being used to optimize WHR system design and performance, offering insights into energy flows and operational dynamics.
Looking ahead, the development of new business models and financing mechanisms is expected to further drive the adoption of WHR systems in cement plants. Performance-based contracts, where technology providers are compensated based on the energy savings achieved, are gaining traction as a way to lower the upfront investment barriers for plant operators. Similarly, public-private partnerships and collaborative research initiatives are fostering innovation and enabling the deployment of cutting-edge WHR technologies in both developed and emerging markets.
In conclusion, waste heat recovery systems are poised to become a cornerstone of sustainable cement manufacturing. By capturing and reusing thermal energy that would otherwise be wasted, these systems improve energy efficiency, reduce emissions, and enhance the economic viability of cement production. As the industry continues to face increasing pressure to decarbonize and adopt circular economy principles, WHR systems will play an increasingly vital role in driving progress toward cleaner, more efficient, and resilient cement plants. With ongoing advancements in technology, policy support, and global collaboration, waste heat recovery is set to transform the cement industry and contribute significantly to the global transition toward sustainable industrial practices.
Waste Heat Recovery System
A waste heat recovery system (WHRS) is a crucial technology used to capture and repurpose excess heat that would otherwise be lost to the environment in industrial processes. This heat, often generated as a by-product of manufacturing processes, machinery, and power generation, can be harnessed and converted into useful forms of energy, such as electricity or thermal energy. Waste heat recovery is an effective strategy for improving energy efficiency, reducing operating costs, and lowering carbon emissions across various industries. WHRS technologies help optimize the use of energy, contributing to sustainability by lowering the need for additional fuel inputs and minimizing environmental impacts.
In most industrial processes, heat is produced during operations, but a significant portion of it is not directly usable for the ongoing process. Instead of allowing this heat to escape into the atmosphere, waste heat recovery systems capture it and transfer it to another medium, where it can be utilized for different applications. This captured heat is often in the form of high-temperature gases or fluids released from equipment such as furnaces, kilns, turbines, or engines. By recovering and repurposing this heat, industries can significantly reduce their overall energy consumption and dependence on external energy sources.
One of the most common methods of waste heat recovery is through the use of heat exchangers. Heat exchangers transfer the heat from the waste gas or fluid to a working medium, such as water, air, or oil, which can then be used to generate steam, provide space heating, or even drive a turbine to generate electricity. These heat exchangers can be designed in various forms, including plate heat exchangers, shell and tube exchangers, and air-to-air exchangers, depending on the specific temperature and application requirements.
In addition to traditional heat exchangers, more advanced systems, such as the Organic Rankine Cycle (ORC) and the Kalina Cycle, have been developed to improve the efficiency of waste heat recovery. These systems use organic fluids with lower boiling points than water, which allows them to extract heat from lower-temperature sources, such as exhaust gases from industrial machinery or engines. The working fluid in an ORC system is vaporized by the waste heat, and its expansion drives a turbine to generate electricity. The Kalina Cycle operates similarly but uses a mixture of water and ammonia, which increases its efficiency by allowing it to recover heat over a wider range of temperatures.
Another common application of waste heat recovery is in combined heat and power (CHP) or cogeneration systems. In these systems, the recovered heat is used not only to produce electricity but also for thermal energy, providing both power and useful heat for processes or heating systems. The dual-purpose nature of CHP systems makes them highly efficient, as they maximize the use of fuel energy and reduce the need for separate electricity and heat generation systems.
Waste heat recovery systems can be found in a wide variety of industries. In power generation, for example, WHRS can be used to recover heat from exhaust gases in gas turbines, improving the overall efficiency of power plants. In the steel industry, waste heat from the furnaces and other equipment can be recovered to generate steam or electricity. Cement plants are also prime candidates for waste heat recovery, as the high temperatures in rotary kilns and preheaters provide substantial opportunities for heat capture.
In addition to improving energy efficiency, waste heat recovery systems offer substantial environmental benefits. By reducing the need for additional fuel consumption, these systems lower greenhouse gas emissions, contributing to the reduction of the industrial carbon footprint. In power generation, for instance, capturing waste heat and converting it into useful energy reduces the amount of fossil fuel needed to generate electricity, resulting in lower emissions of carbon dioxide and other pollutants. This is particularly important in industries that are heavy consumers of energy and are under increasing pressure to meet stringent environmental regulations and carbon reduction targets.
Implementing a waste heat recovery system requires a thorough assessment of the waste heat sources within a facility. Factors such as temperature, quantity, and availability of waste heat, as well as the potential for integration with existing processes, must all be considered when designing an effective WHRS. For instance, if the waste heat is at a high enough temperature, it can be directly used to generate electricity via steam turbines or ORC systems. In contrast, lower-temperature waste heat may be used for heating purposes or to preheat air or water for other processes, such as drying or heating.
Despite the clear advantages, the initial cost of implementing a waste heat recovery system can be a barrier for some industries. While the long-term energy savings and environmental benefits often outweigh the initial investment, the cost of installing and maintaining the necessary equipment can be significant. However, technological advancements and the growing emphasis on sustainability have led to more affordable and efficient WHRS solutions. Additionally, government incentives and regulations aimed at reducing industrial carbon emissions are providing financial support for companies to invest in these systems, making them more accessible.
In addition to cost considerations, the operational challenges of waste heat recovery systems must also be addressed. For example, the quality and consistency of the recovered heat can fluctuate based on changes in the production process, making it necessary to incorporate robust control systems to optimize performance. Regular maintenance and monitoring of the WHRS are crucial to ensure its reliability and maximize its operational lifespan. Over time, wear and tear, especially in harsh industrial environments, can affect the performance of the heat recovery components, requiring periodic checks and replacements.
The future of waste heat recovery systems looks promising, with ongoing research and development focused on increasing the efficiency, flexibility, and cost-effectiveness of these technologies. The growing demand for clean energy solutions and the adoption of renewable energy sources will further drive the integration of waste heat recovery systems. Moreover, the rapid advancement of smart technologies, such as the Internet of Things (IoT) and data analytics, is enabling better monitoring, optimization, and predictive maintenance of WHRS, improving their overall effectiveness.
As industries continue to focus on sustainability, reducing energy consumption, and minimizing environmental impacts, waste heat recovery systems will play an increasingly important role in achieving these goals. From power generation to manufacturing, the potential for waste heat recovery to contribute to energy savings, cost reductions, and emissions reductions is vast. In combination with other energy efficiency measures, waste heat recovery will be essential in creating a more sustainable and energy-efficient industrial landscape. The growing adoption of these systems across industries will contribute to global efforts to reduce energy consumption and mitigate climate change.
As waste heat recovery systems (WHRS) continue to evolve, innovations in both technology and design are expanding their potential applications and improving their efficiency. One key area of development is in the integration of waste heat recovery with renewable energy systems, forming hybrid energy systems that leverage the strengths of both approaches. For example, pairing waste heat recovery with solar thermal or biomass systems can provide a continuous and stable supply of energy, complementing the variable nature of solar or wind power generation. This integration supports the transition to low-carbon energy systems while enhancing energy security and reliability.
Another promising development is the increasing use of advanced materials in WHRS components, which improves heat transfer, durability, and resistance to high temperatures and corrosive environments. For instance, innovations in heat exchanger materials, such as ceramic coatings and heat-resistant alloys, enable more efficient recovery of heat from harsh environments in industries such as steelmaking, glass manufacturing, and cement production. These materials not only extend the lifespan of WHRS components but also enhance their ability to recover heat from lower-temperature waste streams, making it possible to capture more waste energy.
Further, digital technologies and artificial intelligence (AI) are revolutionizing the management and optimization of waste heat recovery systems. By embedding sensors, IoT devices, and AI-based algorithms into WHRS infrastructure, plants can achieve real-time monitoring, predictive analytics, and automatic adjustments to optimize energy recovery based on changing conditions. AI can predict equipment malfunctions, optimize the flow of heat, and fine-tune energy conversion processes, ensuring that waste heat is utilized as efficiently as possible. This advanced data-driven approach reduces downtime, extends equipment life, and improves the overall performance of WHRS, making them even more cost-effective in the long run.
Energy storage technologies are also increasingly being integrated into WHRS, particularly in applications where waste heat recovery is intermittent or fluctuates due to variations in the production process. Thermal energy storage systems allow plants to store excess recovered heat and use it at times when the demand for energy is high or when production levels are lower. For example, phase-change materials (PCMs) and molten salts can store thermal energy efficiently, making it possible to balance the energy supply and demand within a plant. This integration of energy storage with WHRS can further optimize energy use, reduce waste, and improve the plant’s overall efficiency.
In addition to power generation and process heat recovery, WHRS is also becoming increasingly relevant in district heating systems. These systems supply heating to multiple buildings or industrial facilities from a centralized source, and integrating waste heat recovery into district heating networks allows for more efficient use of energy. Cities and industrial clusters can recover excess heat from various sources—such as industrial plants, data centers, or power stations—and distribute it for residential, commercial, or industrial heating. This type of system reduces reliance on fossil fuels for heating, lowers utility costs, and decreases overall greenhouse gas emissions, contributing to the decarbonization of urban energy systems.
The potential for waste heat recovery in transportation is another area of growing interest. In particular, the marine, automotive, and aerospace industries are exploring the use of waste heat recovery systems to improve fuel efficiency and reduce emissions. For example, ships equipped with WHRS can capture heat from exhaust gases and use it to generate steam or electricity, thereby reducing fuel consumption and lowering emissions. Similarly, in heavy-duty trucks or trains, waste heat recovery technologies can be employed to recover heat from engine exhausts, improving the overall efficiency of the vehicles. As the transportation sector shifts toward cleaner technologies and alternative fuels, integrating waste heat recovery into these systems will play an important role in enhancing efficiency and reducing environmental impacts.
A significant aspect of the future development of WHRS lies in its ability to serve as an integral part of industrial symbiosis, where different industries or facilities share resources like heat, water, and power. In industrial clusters or eco-industrial parks, where various manufacturing plants operate in close proximity, waste heat from one plant can be recovered and used as an energy source for another. This circular approach reduces waste, minimizes resource consumption, and maximizes energy use across multiple facilities. By enabling industries to share energy resources, waste heat recovery can facilitate more sustainable industrial practices and create new opportunities for collaboration between different sectors.
Waste heat recovery is also gaining attention in sectors where it has not been traditionally applied, such as data centers. Data centers, which are a significant consumer of electricity for cooling and IT equipment operation, generate a large amount of waste heat. By implementing waste heat recovery systems, data centers can reuse this heat for space heating or provide it to local district heating networks, offsetting their energy consumption and lowering their environmental footprint. The growing demand for data storage and processing, coupled with increasing energy efficiency regulations, is driving data centers to adopt more sustainable solutions, including waste heat recovery technologies.
As energy markets evolve and the demand for cleaner, more efficient energy solutions grows, waste heat recovery will continue to be a vital part of the global energy transition. The development of policies that encourage energy efficiency, reduce carbon emissions, and provide incentives for adopting WHRS will further accelerate its adoption. Governments and organizations worldwide are increasingly recognizing the value of waste heat as a resource, and they are supporting the widespread implementation of WHRS through incentives, grants, and regulations aimed at reducing industrial energy consumption and promoting sustainable practices.
In conclusion, the future of waste heat recovery systems is bright, with significant technological advancements and growing applications across industries. By capturing and reusing excess heat, these systems offer a powerful tool for improving energy efficiency, reducing environmental impacts, and driving the transition to more sustainable industrial processes. From power generation to transportation and beyond, the integration of waste heat recovery will help industries lower their energy costs, reduce carbon emissions, and contribute to global sustainability goals. With continued innovation, policy support, and industry collaboration, waste heat recovery systems are poised to play an increasingly central role in achieving a cleaner, more energy-efficient future.
Waste Heat Recovery Boiler
A waste heat recovery boiler (WHRB) is an essential piece of equipment used in industrial processes to capture and reuse excess heat that would otherwise be lost. These boilers are specifically designed to recover heat from the flue gases produced by combustion processes or from hot exhaust gases generated during industrial operations. By recovering this otherwise wasted heat, WHRBs improve energy efficiency, reduce fuel consumption, and contribute to sustainability by decreasing greenhouse gas emissions.
The core function of a waste heat recovery boiler is to capture the thermal energy from hot exhaust gases and use it to generate steam or hot water. This steam or hot water can then be used in various applications, such as process heating, power generation, or even driving turbines for electricity production. A WHRB operates by transferring the heat from the exhaust gases to water circulating through the boiler, typically using heat exchangers, such as finned tubes or vertical economizers, to maximize heat transfer. By doing so, WHRBs significantly reduce the need for additional fuel consumption, lowering operational costs and improving the overall energy efficiency of industrial plants.
WHRBs are particularly useful in industries where high-temperature exhaust gases are consistently produced, such as in cement manufacturing, steel production, petrochemical processing, and power plants. For instance, in cement plants, the rotary kiln generates high-temperature flue gases, which can be captured by a waste heat recovery boiler. The recovered heat can be used to produce steam, which in turn can power generators or be used for other processes like preheating air or drying raw materials. In power plants, WHRBs recover heat from the flue gases of gas turbines, which can improve the overall efficiency of the plant and lower its carbon footprint.
There are several key factors to consider when designing and installing a waste heat recovery boiler. One important aspect is the temperature of the exhaust gases, as this will determine the type of boiler and heat recovery system required. Waste heat recovery boilers are typically categorized based on the temperature of the exhaust gases they can handle: low-temperature, medium-temperature, or high-temperature boilers. Low-temperature waste heat recovery boilers are suitable for capturing heat from exhaust gases at temperatures below 300°C, while high-temperature boilers are designed for exhaust gases at temperatures above 600°C. The design and materials of the boiler must also be suited to handle the corrosive nature of the flue gases, which can contain impurities like sulfur, chlorine, or particulates, depending on the fuel used.
In addition to temperature, the composition and volume of the exhaust gases play a significant role in the design of a waste heat recovery boiler. For example, in industries that burn fuels with high sulfur content, such as coal or heavy oils, the waste gases may be highly acidic and corrosive, requiring the use of corrosion-resistant materials like stainless steel or specific coatings. Furthermore, the volume of exhaust gases will determine the size and capacity of the WHRB. Large-scale industrial processes with high exhaust gas volumes will require larger boilers with more heat exchange surfaces to recover sufficient amounts of heat.
The efficiency of a waste heat recovery boiler is largely dependent on its design, including the arrangement of heat transfer surfaces, the effectiveness of the heat exchangers, and the ability to maintain optimal fluid flow rates. Advanced heat exchanger designs, such as finned tubes or compact, multi-pass configurations, can maximize heat transfer and minimize energy losses. Additionally, integrating a waste heat recovery boiler with other systems, such as steam turbines or organic Rankine cycle (ORC) systems, can further enhance efficiency by converting recovered heat into electricity.
One of the key advantages of using a waste heat recovery boiler is its ability to reduce the consumption of fossil fuels. By harnessing waste heat, industries can significantly lower their reliance on external energy sources such as natural gas, coal, or oil, which are often used to generate steam or electricity. This reduction in fuel consumption not only leads to cost savings but also helps lower carbon emissions, making it a crucial component of sustainability initiatives. In industries such as cement or steel production, which are energy-intensive and contribute significantly to greenhouse gas emissions, waste heat recovery can play a vital role in decarbonizing operations.
In addition to fuel savings and emissions reduction, WHRBs provide operational benefits, such as enhanced process stability, improved plant efficiency, and greater control over energy usage. By recovering waste heat, industrial plants can ensure that energy is used more effectively, with excess heat being diverted to areas where it is needed most. For example, the steam generated by a waste heat recovery boiler can be used to preheat combustion air, reduce fuel usage in furnaces or kilns, or be integrated into a district heating system, where it can provide energy to nearby facilities or buildings. This helps balance energy demand across the plant, making operations more flexible and cost-effective.
Despite the clear benefits, there are challenges associated with waste heat recovery boiler systems. One of the main challenges is the initial capital investment required for installation. While waste heat recovery boilers provide significant long-term savings, the upfront cost of purchasing, installing, and commissioning the system can be high. However, the long-term return on investment (ROI) often justifies the initial expenditure, especially in energy-intensive industries where fuel costs are a significant portion of overall operational expenses. Additionally, the maintenance of WHRBs can be more complex than traditional boilers, especially in plants with high concentrations of particulates, acids, or other contaminants in the exhaust gases. Regular maintenance and cleaning of heat exchanger surfaces are essential to ensure maximum efficiency and prevent fouling or scaling.
The integration of waste heat recovery boilers into existing plants also requires careful consideration of the plant’s energy systems. Retrofitting an existing facility with a WHRB may involve modifications to the exhaust gas system, the steam generation system, and potentially even electrical systems. For new plant designs, however, waste heat recovery boilers can be integrated from the outset, ensuring that the entire facility operates as efficiently as possible. In both cases, system integration requires detailed analysis of heat flows, energy demands, and process characteristics to optimize the design and maximize the recovery of waste heat.
Looking to the future, the role of waste heat recovery boilers will continue to grow as industries face increasing pressure to reduce energy consumption and meet stricter environmental regulations. The adoption of advanced technologies, such as digital monitoring systems, predictive maintenance tools, and AI-driven optimization algorithms, will further improve the performance and efficiency of WHRBs. These innovations will allow for better tracking of heat recovery performance, more precise control of energy use, and the ability to identify opportunities for improvement. Furthermore, as industries explore more sustainable fuel sources, such as biogas, hydrogen, or renewable electricity, waste heat recovery systems will continue to adapt, capturing heat from a broader range of energy sources and contributing to the transition toward a more sustainable industrial landscape.
In conclusion, waste heat recovery boilers are an essential technology for improving energy efficiency, reducing operational costs, and decreasing carbon emissions in a wide range of industries. By capturing excess heat and converting it into useful energy, WHRBs play a pivotal role in the global push toward sustainability and cleaner industrial practices. With advancements in design, materials, and integration technologies, waste heat recovery boilers will remain a critical tool for achieving higher levels of energy efficiency and helping industries meet their environmental and economic goals.
As industries continue to evolve toward more sustainable practices, the importance of waste heat recovery boilers (WHRBs) in reducing energy consumption and environmental impact becomes even more significant. With global efforts focused on mitigating climate change and reducing dependence on fossil fuels, WHRBs are poised to be central to the future of industrial energy systems. Their ability to repurpose waste heat for beneficial uses not only reduces operational costs but also plays a crucial role in advancing carbon reduction goals.
The integration of waste heat recovery systems with renewable energy technologies is one of the most promising developments in this field. Combining WHRBs with solar thermal, geothermal, or biomass energy systems can create hybrid energy systems that enhance the sustainability of industrial operations. For example, integrating a WHRB with a biomass boiler can help recover heat from exhaust gases while also generating additional power from renewable biomass sources. This combination enables industries to operate more efficiently and reduce reliance on non-renewable energy sources.
Moreover, the development of advanced heat recovery technologies, such as the Organic Rankine Cycle (ORC) and Kalina Cycle, offers the opportunity to further improve the efficiency of waste heat recovery boilers. These systems use organic fluids or mixtures of water and ammonia that have lower boiling points than water, making them capable of extracting energy from lower-temperature waste streams. This innovation opens up new possibilities for recovering waste heat from a broader range of industrial processes, including those with exhaust gases at relatively low temperatures. Integrating WHRBs with ORC systems can significantly increase overall plant efficiency by converting low-grade waste heat into usable electricity.
A growing trend in the design of waste heat recovery boilers is the use of modular and scalable solutions. Modular designs allow for easier integration of waste heat recovery systems into existing plants, with flexibility for future upgrades and expansion. These modular systems can be customized to match the specific needs of different industries, providing an efficient and cost-effective solution for waste heat recovery. For example, a modular WHRB can be tailored to handle varying exhaust gas volumes or to recover heat from different types of industrial processes, making it adaptable to a wide range of applications.
As industrial plants strive to meet stricter environmental regulations and reduce their carbon footprints, the implementation of waste heat recovery boilers will continue to be a vital part of their strategy. Governments worldwide are setting more ambitious carbon reduction targets and energy efficiency standards, which will further encourage industries to adopt WHRB technology. Financial incentives, such as tax credits or subsidies for energy-efficient equipment, will make waste heat recovery systems more accessible to a broader range of industries, from small manufacturers to large-scale power plants.
Digitalization is another area of innovation that is transforming the operation of waste heat recovery boilers. The integration of sensors, Internet of Things (IoT) devices, and data analytics into WHRB systems enables real-time monitoring and optimization of energy recovery. Through data collection and analysis, plant operators can track the performance of the WHRB, identify potential issues before they cause downtime, and fine-tune the system for maximum efficiency. Predictive maintenance algorithms can help anticipate equipment failures and prevent costly repairs, further improving the reliability and performance of WHRBs.
Artificial intelligence (AI) is also being employed to optimize the operation of waste heat recovery systems. AI algorithms can predict energy demand, adjust system parameters in real-time, and optimize heat exchange processes, ensuring that the maximum amount of waste heat is recovered at all times. This intelligent automation improves the overall energy efficiency of industrial processes, reduces energy waste, and minimizes operational costs.
In industries where fluctuating production levels are common, energy storage systems integrated with waste heat recovery boilers offer a promising solution for balancing energy supply and demand. Thermal energy storage technologies, such as molten salts or phase-change materials (PCMs), allow excess heat recovered by the WHRB to be stored and used when needed. For example, a WHRB might recover heat during off-peak hours and store it in a thermal energy storage unit, which can then release the stored heat to meet peak demand periods. This approach can significantly reduce the need for additional fuel consumption during high-demand times, further improving the overall energy efficiency of the plant.
In the context of industries like cement production, steel manufacturing, and petrochemical processing—where high-temperature exhaust gases are a by-product of essential operations—waste heat recovery boilers can play an especially critical role in improving energy efficiency. For example, in cement plants, WHRBs can recover heat from the exhaust gases produced by the rotary kilns, which operate at temperatures often exceeding 1000°C. The recovered heat can then be used for preheating raw materials, drying, or even for power generation, reducing the need for supplementary heating and improving the overall thermal efficiency of the plant.
Beyond large-scale industrial applications, waste heat recovery boilers can also be beneficial in smaller and more specialized operations. For example, in data centers, which generate significant amounts of heat due to the constant operation of servers and cooling systems, WHRBs can capture this heat and use it for district heating or process heating applications. With the rise of cloud computing and data processing, the demand for data centers is increasing, making it crucial for these facilities to adopt energy-efficient solutions like waste heat recovery boilers.
The ongoing development of alternative fuel sources, such as hydrogen and biogas, presents additional opportunities for waste heat recovery systems. As industries transition to hydrogen or biogas-based processes, WHRBs can capture and utilize the excess heat generated during hydrogen production or biogas combustion. This integration can further reduce the carbon intensity of industrial processes and support the global shift toward cleaner, renewable energy sources.
In conclusion, waste heat recovery boilers represent a vital technology for industries aiming to improve energy efficiency, reduce costs, and meet sustainability targets. By capturing and reusing excess heat, WHRBs provide significant environmental benefits, reduce fuel consumption, and lower carbon emissions. With ongoing advancements in technology, integration with renewable energy systems, and the incorporation of digital tools and AI, the potential applications of waste heat recovery boilers are vast and growing. These systems will continue to play a crucial role in the global effort to create more energy-efficient, sustainable industrial processes while contributing to the reduction of global greenhouse gas emissions.
How to Convert Heat Energy to Electricity
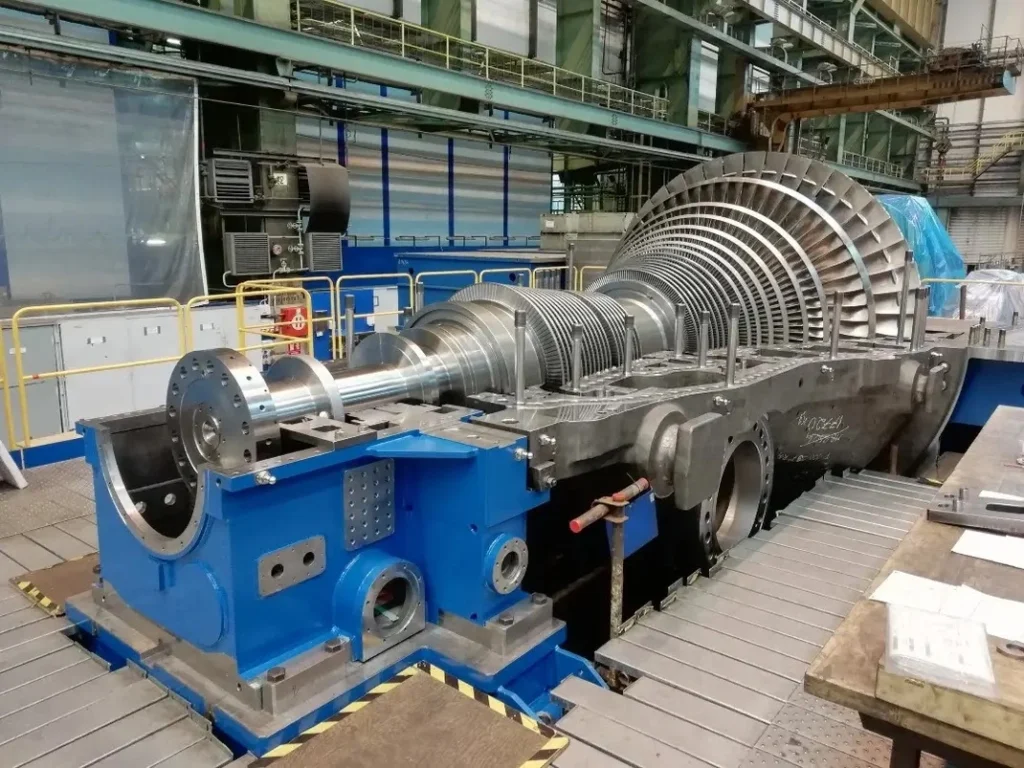
Converting heat energy to electricity is a fundamental process used in various industries and technologies, enabling the efficient use of thermal energy from different sources. This conversion is typically achieved through thermodynamic cycles, heat engines, and specialized devices designed to harness heat and transform it into electrical energy. The most common methods for converting heat to electricity include thermoelectric generators, steam turbines, organic Rankine cycles (ORC), and thermophotovoltaic cells, each with its own set of applications, benefits, and challenges.
Thermoelectric generators (TEGs) are one of the most direct methods for converting heat into electricity. These devices use the Seebeck effect, a phenomenon where a temperature difference between two materials generates an electrical voltage. In a TEG, one side of the device is heated, while the other side is kept cooler. The temperature gradient causes electrons to move from the hot side to the cooler side, creating a flow of electric charge. This principle is similar to the way heat flows naturally from hot to cold areas, but TEGs utilize this flow to generate electricity. TEGs are widely used in applications where heat is available, such as in waste heat recovery systems or in remote, off-grid locations where traditional power sources are impractical. However, their efficiency is relatively low compared to other methods, as the voltage produced depends on the temperature difference and the materials used.
Another well-known method for converting heat into electricity is the steam turbine system, commonly used in power plants. In this system, heat energy is used to generate steam from water. The steam, under high pressure, is directed onto the blades of a turbine, causing it to spin. The turbine is connected to a generator, which converts the mechanical energy of the rotating turbine into electrical energy. Steam turbines are the cornerstone of conventional power plants, including fossil fuel and nuclear plants, where heat from the combustion of coal, gas, or nuclear reactions produces steam. The efficiency of steam turbines is generally high, and they are capable of generating large amounts of electricity. However, they require a significant initial investment in infrastructure and are subject to operational inefficiencies related to heat losses and cooling requirements.
The Organic Rankine Cycle (ORC) is a variation of the steam turbine system, specifically designed to generate electricity from lower-temperature heat sources. Instead of using water as the working fluid, the ORC uses organic fluids that have a lower boiling point. This allows the ORC to operate efficiently with heat sources that might not reach the high temperatures required for traditional steam turbines. In an ORC system, heat is applied to the organic fluid, causing it to vaporize and expand. The expanding gas drives a turbine or a piston, which is connected to a generator. ORC systems are particularly well-suited for waste heat recovery applications, geothermal energy, and biomass power generation, where the available heat is often too low for steam-based turbines. The ORC offers the advantage of being able to recover waste heat from industrial processes, thereby improving overall energy efficiency.
Thermophotovoltaic (TPV) cells represent a more recent technology for converting heat directly into electricity. These cells work by absorbing infrared radiation from a heat source and converting it into electrical power. The heat source emits infrared radiation, which is absorbed by the TPV cell, typically made from semiconducting materials. The absorbed radiation excites the electrons in the semiconductor, causing them to move and generate electricity. TPV cells are still in the experimental and developmental phase for most applications, but they hold promise for use in environments where waste heat is abundant, such as industrial sites, spacecraft, and remote areas. One of the challenges with TPVs is improving their efficiency, as most of the infrared radiation from heat sources is not perfectly matched to the semiconductor’s absorption spectrum, limiting the amount of energy that can be converted into electricity.
In addition to these technologies, there are several other innovative methods for converting heat energy into electricity. One example is the use of piezoelectric materials, which generate an electrical charge when subjected to mechanical stress. While piezoelectric materials are typically used to convert mechanical vibrations into electricity, they can also be employed in systems that use heat-induced mechanical deformation. In this setup, heat causes the materials to expand or contract, creating stress that generates electricity. Though this technology is still being explored, it offers a potential alternative for low-grade heat recovery, particularly in environments with constant vibrations or motion.
Another emerging approach is the use of Stirling engines, which convert heat energy into mechanical energy through the expansion and contraction of a gas within a sealed cylinder. The Stirling engine operates by heating a gas, causing it to expand, and then cooling it, which causes the gas to contract. The pressure changes move a piston, which can be connected to a generator to produce electricity. Stirling engines are highly efficient and capable of using various heat sources, from solar to biomass and waste heat. While they are well-suited for small-scale and off-grid applications, their adoption has been limited by the complexity of maintaining the heat exchange system and the high costs associated with engine components.
Thermal energy storage systems also play a role in the conversion of heat to electricity. These systems store excess heat when it is available and release it when needed, allowing for more flexible use of thermal energy. One common approach is to store heat in materials such as molten salts, which can hold heat for extended periods and release it gradually when needed. This stored heat can then be used to power steam turbines or ORC systems for electricity generation. This combination of thermal energy storage and heat conversion systems can help provide consistent and reliable electricity, particularly in renewable energy applications such as concentrated solar power (CSP) plants.
In addition to direct conversion technologies, heat exchangers and other devices can be employed to improve the efficiency of heat energy conversion. Heat exchangers, by transferring heat from one fluid to another, allow for more effective use of the heat energy in various industrial and power generation processes. The more efficiently the heat is transferred, the more potential there is for converting that heat into usable electricity. In many applications, such as waste heat recovery, heat exchangers are used to increase the thermal efficiency of the system by reducing the temperature of the exhaust gases before they are released into the atmosphere.
The development and improvement of these technologies will continue to be a critical focus in efforts to address global energy challenges, reduce waste, and increase the efficiency of energy conversion processes. As renewable energy sources, such as solar and geothermal, become more prominent, the ability to efficiently convert heat energy into electricity will be essential for maximizing their potential. Moreover, the growing focus on industrial energy efficiency and the reduction of greenhouse gas emissions will drive the adoption of heat-to-electricity conversion technologies across various sectors.
In conclusion, converting heat energy to electricity is an essential process in modern energy systems, offering a method to harness and utilize thermal energy from a variety of sources. The technologies involved in heat-to-electricity conversion, such as thermoelectric generators, steam turbines, ORCs, and thermophotovoltaic cells, each have their advantages and limitations. As industries and technologies evolve, further advancements in materials, system design, and integration with renewable energy sources will continue to improve the efficiency and viability of heat energy conversion. These innovations will be central to reducing energy consumption, minimizing environmental impact, and achieving sustainable energy solutions across the globe.
As the demand for energy continues to grow globally, the need to harness every possible source of energy, including heat, becomes increasingly crucial. The conversion of heat to electricity not only contributes to energy efficiency but also aligns with sustainability goals aimed at reducing carbon emissions and reliance on fossil fuels. This ongoing push for energy innovation has led to the development of advanced technologies and systems that improve the efficiency of heat-to-electricity conversion processes.
One of the most significant challenges in converting heat to electricity is the efficiency of the conversion process. Traditional methods, such as steam turbines, have high efficiency when operating with high-temperature heat sources, but their efficiency decreases with lower temperature heat. To overcome this limitation, researchers are focusing on developing new materials and technologies that can operate effectively at lower temperatures. For instance, new thermoelectric materials with higher Seebeck coefficients, such as bismuth telluride and lead telluride alloys, are being developed to improve the efficiency of thermoelectric generators. These materials are designed to provide a larger voltage output for a given temperature difference, enhancing the performance of TEGs even in lower temperature environments.
In the same vein, the development of new organic fluids for use in ORC systems is also a priority. Organic Rankine Cycles are a promising technology for recovering waste heat from lower temperature sources, such as industrial exhaust gases or geothermal fluids. The organic fluids used in ORCs are being optimized to improve their thermal properties, allowing for more efficient heat absorption and energy conversion. Researchers are also exploring the use of supercritical fluids, which exhibit superior thermodynamic properties and can further increase the efficiency of ORC systems.
For applications that require consistent and reliable heat-to-electricity conversion, hybrid systems combining multiple technologies are being explored. One example is the combination of solar thermal energy and thermoelectric generators or ORC systems. In such systems, concentrated solar power is used to heat a working fluid, which can then be directed to an ORC or TEG system to generate electricity. This hybrid approach not only increases the overall efficiency of the system but also allows for energy production even when sunlight is intermittent, as heat can be stored and used when needed.
Waste heat recovery systems are also undergoing significant advancements. As industries continue to focus on reducing their carbon footprint and improving energy efficiency, the recovery of waste heat is becoming an essential part of industrial energy management. Technologies such as heat pumps, which can upgrade low-grade waste heat to higher temperatures, are being integrated into waste heat recovery systems to improve the conversion efficiency. Heat pumps work by transferring heat from a lower temperature source to a higher temperature one using a refrigeration cycle. By coupling heat pumps with existing heat-to-electricity conversion systems, such as ORCs or steam turbines, the overall efficiency of waste heat recovery systems can be significantly enhanced.
The transportation sector, including electric vehicles (EVs) and shipping, is another area where heat-to-electricity conversion technologies are seeing significant interest. In electric vehicles, waste heat from the vehicle’s motor, battery, and exhaust can be captured and converted into electricity, reducing the load on the vehicle’s battery and extending its range. Similarly, in marine shipping, waste heat recovery systems are being integrated into ship engines to improve fuel efficiency and reduce emissions. In both cases, the use of heat recovery systems allows for more sustainable and energy-efficient operations, reducing fuel consumption and the environmental impact of transportation.
Research into advanced thermophotovoltaic (TPV) systems is also promising. While TPVs have been mostly experimental, they offer the potential to directly convert heat into electricity using semiconductor materials designed to absorb specific wavelengths of infrared radiation. This technology could revolutionize the way heat is converted to electricity, especially in high-temperature environments. TPVs could eventually replace conventional heat engines in certain applications, such as space exploration or remote power generation, where traditional mechanical engines may not be practical. The challenge remains to improve the efficiency of TPVs by optimizing their material properties and better matching the radiation spectrum emitted by the heat source.
As more industries adopt renewable energy sources like geothermal, biomass, and solar, the integration of heat-to-electricity technologies will play a pivotal role in optimizing energy systems. The ability to recover and convert low-grade waste heat into electricity can reduce the need for additional fuel consumption, making renewable energy systems more economically viable and environmentally friendly. For example, geothermal power plants already use heat from the Earth’s interior to generate electricity, but integrating waste heat recovery can further enhance their efficiency. Similarly, biomass power plants, which burn organic materials to generate heat, can use the excess heat from the combustion process for additional electricity generation, improving overall plant efficiency.
In the industrial sector, the adoption of advanced heat recovery technologies is also driven by the desire to comply with increasingly stringent energy efficiency regulations. Many countries have set ambitious goals to reduce their carbon footprints, and industries must find innovative solutions to meet these targets. Waste heat recovery, combined with heat-to-electricity conversion systems, helps industries reduce their reliance on external energy sources and cut down on emissions. In fact, the recovery of waste heat is often one of the easiest and most cost-effective methods for industries to achieve significant energy savings. Industries that use large amounts of heat, such as cement, steel, and chemical manufacturing, can greatly benefit from implementing heat-to-electricity conversion systems, as these technologies can help offset energy costs and improve profitability.
As energy markets become more decentralized, distributed energy systems are expected to play an increasingly important role in electricity generation. Heat-to-electricity conversion technologies, especially those that can operate on small-scale, localized waste heat sources, will be crucial in powering these decentralized energy systems. Whether in urban environments or remote locations, small-scale heat-to-electricity systems can provide a reliable and cost-effective power source, reducing the need for centralized power plants and grid infrastructure.
In conclusion, converting heat energy to electricity is a critical technology that enables more efficient use of available thermal resources. From thermoelectric generators and steam turbines to organic Rankine cycles and thermophotovoltaic cells, various methods exist to convert heat into electricity, each suited to different applications. Advancements in materials science, system design, and hybrid technologies will continue to improve the efficiency of heat-to-electricity conversion processes. As industries, transportation sectors, and renewable energy systems increasingly focus on energy efficiency and sustainability, the role of heat recovery and conversion technologies will become even more significant, contributing to a more sustainable and energy-efficient future.
Can We Turn Heat into Electricity
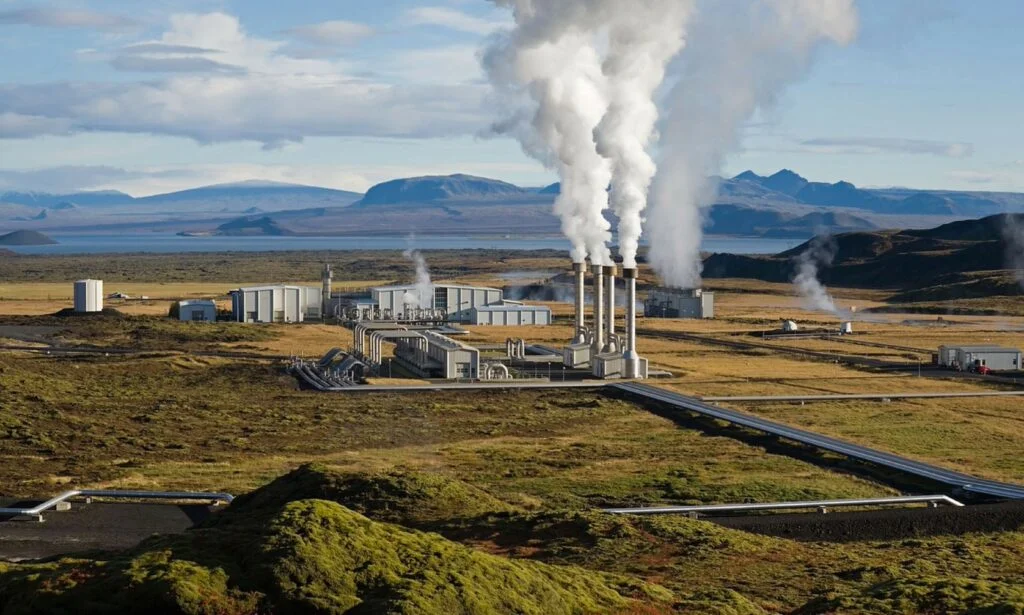
Yes, it is possible to turn heat into electricity, and this process is an essential aspect of many modern energy systems. Heat can be converted into electricity using various technologies that rely on thermodynamic principles. The conversion of heat into electrical energy is achieved through the exploitation of heat gradients, thermodynamic cycles, and specific materials that can generate an electrical charge when subjected to temperature differences. The methods used for turning heat into electricity vary depending on the temperature range, the available heat source, and the efficiency of the technology.
One of the most widely known methods for converting heat into electricity is through the use of thermoelectric generators (TEGs). TEGs operate on the principle of the Seebeck effect, where a temperature difference between two materials creates an electric voltage. In a thermoelectric generator, one side of the device is exposed to a heat source, while the other side is kept cooler. The heat causes the charge carriers (typically electrons) to move from the hot side to the cooler side, generating an electrical current. While TEGs are highly reliable and have no moving parts, their efficiency is relatively low, especially for small temperature differences. However, they are ideal for applications where small-scale, off-grid power generation is needed, such as in remote locations or in waste heat recovery systems.
For higher-temperature heat sources, such as those in power plants, steam turbines are the most common technology used to convert heat into electricity. In a steam turbine system, heat from the combustion of fossil fuels, nuclear reactions, or geothermal energy is used to produce steam from water. This steam, under high pressure, is directed to spin a turbine, which is connected to a generator. As the turbine spins, mechanical energy is converted into electrical energy. This process is highly efficient at larger scales and is the core method for electricity generation in many traditional power plants. The efficiency of steam turbines is closely linked to the temperature of the steam and the effectiveness of the heat exchangers that supply the steam.
Another promising technology for converting heat to electricity, especially from low-temperature heat sources, is the Organic Rankine Cycle (ORC). ORC systems operate similarly to steam turbines but use organic fluids that have lower boiling points compared to water. These organic fluids are vaporized by heat, causing them to expand and drive a turbine or piston, which is then used to generate electricity. ORCs are particularly useful in applications such as waste heat recovery, geothermal power generation, and biomass energy, where heat sources may not reach the high temperatures required for conventional steam turbines. Because the working fluid in ORC systems boils at a lower temperature than water, they are more efficient than steam turbines for recovering low-grade heat.
A more experimental approach to converting heat into electricity is through thermophotovoltaic (TPV) cells. TPV cells convert infrared radiation emitted by a hot surface directly into electricity. These cells operate on the principle that when infrared radiation strikes a semiconductor material, it excites electrons and generates an electric current. While TPVs are not yet widely used, they have the potential to become a revolutionary technology for converting heat into electricity, especially in high-temperature environments. TPV systems are being researched for use in spacecraft, remote sensing devices, and high-temperature industrial processes. The efficiency of TPV cells is still a challenge, as they require specific materials that can absorb a broad spectrum of infrared radiation and convert it efficiently into electricity.
Stirling engines are another heat-to-electricity technology that operates by converting heat into mechanical energy. Stirling engines use a closed-loop thermodynamic cycle in which a working gas is alternately heated and cooled, causing it to expand and contract. This expansion and contraction move a piston, which is connected to a generator that produces electricity. Stirling engines can operate with a variety of heat sources, including solar energy, biomass, or waste heat from industrial processes. Their high efficiency and the fact that they can run on any heat source make them ideal for both small-scale and large-scale applications. However, their complexity and cost are limitations to widespread adoption.
Additionally, heat-to-electricity conversion is also explored in the field of piezoelectric materials, although these are primarily used to convert mechanical energy into electricity. Piezoelectric devices generate an electric charge when subjected to mechanical stress. In the context of heat conversion, piezoelectric materials can potentially harness the mechanical deformation caused by temperature changes (e.g., when a material expands or contracts due to heat) to generate electricity. This technology is still in its infancy for heat-to-electricity applications, and more research is needed to make it a viable alternative.
In many industrial applications, waste heat recovery plays a significant role in improving energy efficiency and reducing overall energy consumption. Many industrial processes generate excess heat that is released into the environment, representing a wasted resource. By employing heat recovery systems that capture this waste heat and convert it into electricity, industries can reduce their reliance on external power sources and lower operating costs. Heat exchangers, thermoelectric generators, and ORC systems are often used in these scenarios to recover heat from exhaust gases, cooling systems, or other thermal processes. In addition to improving energy efficiency, waste heat recovery helps to minimize environmental pollution by reducing the need for additional fuel combustion and greenhouse gas emissions.
In the field of renewable energy, the conversion of heat into electricity is also a key focus, particularly for technologies like concentrated solar power (CSP). CSP systems use mirrors or lenses to focus sunlight onto a receiver, which collects the concentrated heat and uses it to generate steam. This steam drives a turbine connected to a generator, producing electricity in a process similar to conventional power plants but powered by solar energy. CSP systems can be combined with thermal energy storage to provide continuous electricity generation, even when the sun is not shining.
Despite these advances, the efficiency of converting heat to electricity remains a key challenge. Most current technologies for heat-to-electricity conversion are more effective at higher temperatures, and the efficiency of lower-temperature heat sources (such as industrial waste heat) is typically lower. Improving the efficiency of these systems is the focus of ongoing research, which includes developing better materials for thermoelectric generators, optimizing organic fluids for ORC systems, and finding new ways to improve the efficiency of thermophotovoltaic cells.
In conclusion, turning heat into electricity is not only possible, but it is also a vital technology for improving energy efficiency, reducing waste, and contributing to sustainability. From thermoelectric generators and steam turbines to organic Rankine cycles and thermophotovoltaic cells, there are several promising methods to convert heat into electricity. Each method has its own advantages and challenges, but they all share the potential to harness heat energy, reduce reliance on traditional energy sources, and contribute to the global transition to cleaner and more efficient energy systems. As research and development in this field continue, we can expect these technologies to become more efficient, cost-effective, and widely adopted in a variety of applications.
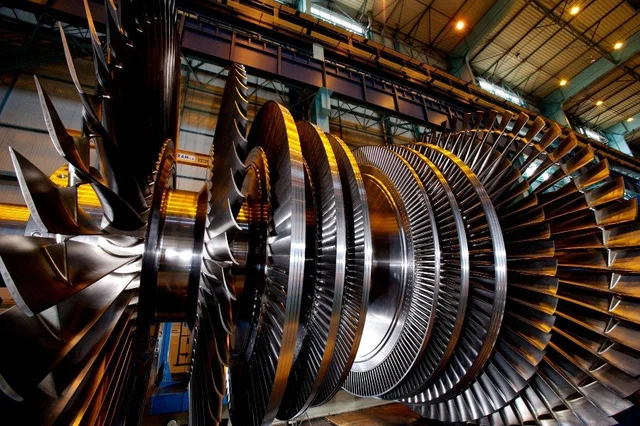
The future of heat-to-electricity conversion is poised to make significant strides, driven by advancements in materials science, engineering, and thermodynamic efficiency. As industries, transportation sectors, and residential applications seek ways to enhance energy efficiency and reduce environmental impacts, the integration of heat recovery and conversion technologies will play a crucial role in addressing the world’s growing energy demands.
One key area of development lies in the advancement of thermoelectric materials. As mentioned, thermoelectric generators (TEGs) rely on materials that can convert temperature gradients directly into electricity. Ongoing research is focused on improving the performance of these materials by increasing their thermoelectric efficiency, which is determined by a property known as the thermoelectric figure of merit (ZT). This figure of merit is a function of the material’s electrical conductivity, thermal conductivity, and Seebeck coefficient, and improvements in any of these factors can enhance the overall efficiency of TEGs. New materials, such as nanostructured thermoelectrics, quantum dot-based systems, and topological insulators, are being explored to push the limits of thermoelectric efficiency, even at lower temperature differences. These innovations could dramatically increase the viability of TEGs for a broader range of applications, from waste heat recovery in factories to powering remote devices in off-grid locations.
In addition to material advancements, there is a growing interest in hybrid systems that combine multiple heat-to-electricity technologies to increase overall energy efficiency. For example, the combination of thermoelectric generators and organic Rankine cycles (ORC) could enable greater flexibility in heat recovery, allowing for efficient electricity generation from both high- and low-temperature heat sources. Such hybrid systems are particularly advantageous in industries with variable heat sources, such as manufacturing, cement production, and power plants. By integrating different technologies, industries can maximize the recovery of waste heat and improve the overall efficiency of their operations.
Hybrid systems are also being explored for renewable energy applications. In solar power, for instance, the combination of concentrated solar power (CSP) and thermoelectric generators could provide a way to harness heat in more flexible and efficient ways. CSP systems are particularly well-suited to regions with abundant sunlight, where they use mirrors or lenses to concentrate solar energy onto a central receiver, which then generates steam to drive turbines. By incorporating thermoelectric generators into the system, the excess heat that would otherwise be lost could be used for further electricity generation. This approach could increase the overall efficiency of solar power plants, making them even more competitive with other forms of renewable energy.
Another promising technology for heat-to-electricity conversion is the integration of heat recovery systems with energy storage solutions. For example, combining waste heat recovery with thermal energy storage (TES) systems could provide a reliable, round-the-clock energy source. In a typical TES system, excess heat is stored during periods of low demand and then used to generate electricity when needed. This setup would be particularly useful in applications where heat sources are intermittent or fluctuate over time, such as in industrial processes or geothermal power plants. By incorporating energy storage, these systems can smooth out fluctuations in energy supply and make the conversion of heat to electricity more stable and efficient.
Moreover, waste heat recovery is gaining traction in the transportation sector, especially in electric vehicles (EVs). As electric vehicles become more widespread, there is an increasing need to optimize their energy consumption, particularly in terms of thermal management. EVs generate a considerable amount of heat during operation, particularly in the battery and motor systems. Some companies are investigating the potential for converting this waste heat into electricity through thermoelectric generators or other heat recovery systems. This approach could help extend the range of electric vehicles by providing a supplementary power source, thereby reducing the need to rely solely on the vehicle’s battery.
The shipping and maritime industries are also exploring ways to convert waste heat into electricity to improve energy efficiency. Large ships, for instance, produce vast amounts of waste heat during their operation, particularly from exhaust gases, engines, and boilers. By integrating waste heat recovery systems onboard, such as ORC systems or thermoelectric generators, shipping companies can improve fuel efficiency, reduce operating costs, and minimize emissions. With increasing regulations on carbon emissions in the maritime sector, the adoption of heat-to-electricity technologies could become a key factor in making the industry more sustainable and compliant with environmental standards.
As the global economy continues to shift toward cleaner energy and more sustainable practices, waste heat recovery and heat-to-electricity conversion will play an essential role in minimizing energy waste. Industries and power plants that rely on large-scale heat generation and processes stand to benefit greatly from these technologies, as they allow for the recovery and utilization of heat that would otherwise be discarded. Additionally, advancements in materials, system design, and integration with energy storage technologies will increase the potential for heat-to-electricity systems to operate efficiently in a broader range of applications.
In conclusion, the conversion of heat into electricity is not only technically feasible, but it is also a critical pathway to improving energy efficiency and sustainability across a wide range of industries. Through the development of advanced materials, hybrid systems, and integrated solutions, the potential for utilizing waste heat to generate electricity is vast. As the technologies continue to evolve, we can expect to see more widespread adoption and integration of these systems, which will contribute to reducing global energy consumption, lowering carbon emissions, and helping industries meet the growing demand for clean, efficient energy solutions. The ongoing research and innovation in heat-to-electricity technologies will shape the future of energy generation, making the most out of the heat that is currently being wasted, and ultimately contributing to a greener, more sustainable world.
Waste Heat to Electricity Conversion Equipment
Waste heat to electricity conversion equipment plays a crucial role in improving energy efficiency across various industries by recovering excess heat that would otherwise be wasted. This process not only contributes to the reduction of energy consumption but also helps industries lower their operational costs and reduce environmental impact. The types of equipment used for waste heat conversion depend on the temperature range of the available heat, the scale of the application, and the efficiency requirements.
One of the most common pieces of waste heat to electricity conversion equipment is the Thermoelectric Generator (TEG). TEGs operate based on the Seebeck effect, where a temperature difference across two different conductive materials generates an electric voltage. When one side of the TEG is exposed to a heat source (such as industrial exhaust gases) and the other side is kept cooler, charge carriers in the materials move, generating a current. The main advantage of thermoelectric generators is their lack of moving parts, making them reliable and low maintenance. However, TEGs generally suffer from low conversion efficiencies, particularly at small temperature gradients. Despite these limitations, they are well-suited for small-scale applications, including powering remote sensors, monitoring devices, and recovering waste heat from low-grade heat sources such as automotive exhausts or electronics.
For higher-temperature heat sources, Organic Rankine Cycle (ORC) systems are widely used to convert waste heat into electricity. ORC systems are similar to traditional Rankine cycles used in steam turbines but use organic fluids with lower boiling points instead of water. This allows ORCs to be more efficient at lower temperatures. The working fluid in an ORC system absorbs heat from an industrial process or other heat sources, vaporizing and expanding to drive a turbine or piston, which in turn powers a generator to produce electricity. ORC systems are commonly used in industries such as cement production, biomass power generation, and waste heat recovery from exhaust gases. One of the major advantages of ORCs is their ability to recover heat from sources with temperatures ranging from 90°C to 400°C, making them suitable for a wide range of industrial applications. The technology is particularly useful for low-temperature heat recovery in applications where steam turbines would be less efficient.
Another widely used waste heat conversion equipment is the Steam Turbine. Steam turbines have long been the workhorse of power generation, and they are still a common method for converting heat into electricity, particularly in larger-scale systems. In waste heat recovery applications, the heat is typically captured from industrial processes, such as the exhaust gases from a furnace or gas turbine, and used to generate steam. The steam is then directed to a steam turbine where it expands, driving the turbine blades. The mechanical energy generated by the turbine is converted into electrical energy by a connected generator. Steam turbines are highly efficient when working with high-temperature heat sources, but their efficiency decreases significantly with lower temperatures. To enhance the effectiveness of steam turbines in waste heat recovery, they are often paired with heat exchangers, economizers, or other systems to optimize the heat recovery process.
Stirling Engines are another type of equipment used for converting waste heat to electricity. These engines operate on a closed-loop cycle, where a working gas (typically air or hydrogen) is alternately heated and cooled, causing it to expand and contract. This movement is used to drive a piston, which is connected to a generator to produce electricity. Stirling engines are known for their high efficiency and ability to use a wide range of heat sources, including solar, biomass, and waste heat from industrial processes. One of the key benefits of Stirling engines is their ability to operate with very low-temperature differences, making them suitable for converting waste heat from relatively low-temperature sources. They are also quieter and have fewer emissions than conventional internal combustion engines. However, their high initial cost and the complexity of the technology can limit their widespread use in certain applications.
Thermophotovoltaic (TPV) Systems are an emerging technology that directly converts heat into electricity through the interaction of light (typically infrared radiation) with semiconductor materials. In a TPV system, a hot surface emits radiation that is absorbed by a semiconductor, which then excites electrons and generates an electrical current. TPV systems can operate at very high temperatures and offer the potential for high conversion efficiencies, especially when using advanced materials that can absorb and convert a broad spectrum of infrared radiation. However, TPV systems are still in the experimental stages and are not yet widely deployed for waste heat recovery applications. Significant research is ongoing to improve the efficiency and scalability of these systems, making them a promising future technology for converting high-temperature waste heat to electricity.
In addition to individual technologies, Waste Heat Recovery Boilers are often integrated into industrial systems to recover heat from exhaust gases or hot fluids. These boilers are designed to capture heat from the exhaust of industrial processes, such as furnaces, gas turbines, or chemical reactors, and use it to generate steam. The steam produced can then be used to drive turbines, which generate electricity, or be redirected to other processes in the facility. Waste heat recovery boilers are commonly used in industries such as cement manufacturing, steel production, and petrochemical plants. The design of these boilers typically includes a combination of heat exchangers and economizers to maximize the amount of heat recovered from exhaust gases.
Heat Pumps are also being incorporated into waste heat recovery systems to improve efficiency. Heat pumps use a refrigeration cycle to transfer heat from a low-temperature source to a higher-temperature sink, effectively “upgrading” the waste heat for use in electricity generation or other applications. By increasing the temperature of low-grade waste heat, heat pumps make it more suitable for conversion into electricity via technologies like ORCs or steam turbines. Heat pumps are particularly effective when coupled with low-temperature waste heat sources, such as industrial cooling systems, or renewable energy systems, where the temperature difference between the source and the sink is not large enough to directly drive an electricity-generating cycle.
For large-scale applications, Waste Heat Recovery Power Plants are being developed to recover waste heat from multiple industrial processes and convert it into electricity. These power plants typically use a combination of the technologies mentioned above, such as ORCs, steam turbines, and heat exchangers, to optimize the recovery and conversion of waste heat. Such systems are often integrated with other energy recovery systems, such as combined heat and power (CHP) plants, to maximize energy efficiency and reduce the overall carbon footprint of the industrial operations.
Gas Turbine Exhaust Heat Recovery is another common method for converting waste heat into electricity. In this system, the exhaust gases from a gas turbine, which typically operates in combined-cycle power plants, are used to generate steam. This steam is then used to drive a steam turbine, generating additional electricity. Gas turbine exhaust heat recovery is an effective way to enhance the efficiency of combined-cycle power plants, which already achieve high efficiency by utilizing both gas and steam turbines in tandem.
In summary, there is a wide range of waste heat to electricity conversion equipment, each suitable for different temperature ranges, scales, and applications. Technologies like thermoelectric generators, organic Rankine cycles, steam turbines, Stirling engines, and thermophotovoltaic systems all play vital roles in recovering and converting waste heat into electricity. The choice of technology depends on factors such as the available heat source temperature, the desired efficiency, and the application at hand. With ongoing research and advancements in materials and system design, these technologies are expected to become more efficient, cost-effective, and widely adopted in a variety of industries, leading to a more sustainable and energy-efficient future.
As the demand for energy efficiency continues to rise, particularly in industrial sectors and large-scale power generation, the development and adoption of waste heat recovery systems will become increasingly critical. Innovations in heat-to-electricity technologies aim to expand the range of heat sources that can be used effectively for power generation, whether it’s from industrial processes, transportation systems, or renewable energy operations. These developments are expected to revolutionize how industries handle their energy consumption and contribute to the global shift toward more sustainable energy practices.
One notable trend is the increasing integration of waste heat recovery systems with renewable energy technologies, such as solar and geothermal power. For instance, in solar power plants, waste heat recovery equipment like ORCs or heat pumps can be incorporated to maximize the efficiency of solar thermal systems. These systems concentrate solar energy to produce heat, which can be harnessed by the waste heat recovery equipment to generate electricity or improve system efficiency. Similarly, geothermal plants often have a high potential for waste heat recovery, and integrating technologies like ORCs can help recover additional energy from the geothermal fluids or steam, making the entire system more energy-efficient.
Industries that produce large amounts of waste heat, such as steel manufacturing, cement plants, chemical processing, and refining, stand to benefit significantly from these technologies. The waste heat generated in such industries is often at high temperatures and in significant quantities. By installing waste heat recovery systems, these industries can harness previously discarded thermal energy and convert it into useful electricity, offsetting the need to purchase electricity from the grid and lowering operational costs. In many cases, these systems also provide a secondary benefit by improving the overall thermal efficiency of the plant, reducing fuel consumption, and minimizing greenhouse gas emissions.
Additionally, district heating systems, which distribute heat to residential, commercial, and industrial buildings, are increasingly using waste heat recovery technologies. Instead of relying entirely on traditional energy sources like natural gas or oil, waste heat from industrial processes or power plants can be redirected into district heating networks, providing a more sustainable and cost-effective way of heating large areas. As district heating networks continue to expand, integrating waste heat recovery solutions could offer substantial environmental and economic benefits.
In the transportation sector, the use of waste heat recovery equipment is gaining traction, especially in electric vehicles (EVs) and heavy-duty transport like trucks and ships. For example, heavy-duty vehicles generate significant amounts of heat, especially from exhaust gases. Companies are investigating the use of ORC systems and thermoelectric generators to recover this waste heat, converting it into electricity that could be used to power auxiliary systems or, in the case of electric vehicles, supplement the battery charging. This would reduce fuel consumption and extend the range of EVs and trucks by capturing heat that would otherwise be wasted. Similarly, in shipping, waste heat recovery technologies are used to convert the heat from engine exhausts into electricity, improving fuel efficiency and reducing emissions. As global regulations become more stringent on shipping emissions, such systems will play an increasingly important role in helping companies comply with environmental standards while lowering operating costs.
Another area where waste heat recovery systems are having a significant impact is in data centers. As data centers continue to grow in scale and importance, they are generating an increasing amount of heat from their servers and computing equipment. Many data centers are now using heat recovery technologies to capture this waste heat and either use it internally for heating or redirect it to nearby facilities, helping to reduce the overall energy footprint. In some cases, the heat is used to warm nearby buildings or even contribute to district heating networks, turning waste heat into a valuable resource.
In waste-to-energy (WtE) plants, the integration of waste heat recovery technologies has proven to be particularly valuable. These plants convert municipal solid waste into electricity and heat, and the efficiency of the process can be greatly improved by incorporating waste heat recovery systems. The heat produced from burning waste can be used to generate steam, which can then drive turbines to produce additional electricity. Alternatively, the heat can be used to meet the heating needs of nearby communities, further enhancing the economic and environmental benefits of these plants.
As industries across the globe continue to adopt and refine waste heat recovery systems, the importance of effective system design, optimization, and integration with existing infrastructure cannot be overstated. These systems must be carefully tailored to the specific needs and characteristics of each industry, taking into account factors like the temperature and volume of waste heat, the available infrastructure, and the economic and environmental goals of the organization.
In the future, the evolution of smart grid systems and energy storage solutions will further enhance the potential of waste heat recovery technologies. Waste heat that is captured and converted into electricity can be stored in energy storage systems for later use or fed into the grid to offset demand. This capability will become increasingly important as intermittent renewable energy sources like solar and wind continue to grow in prominence. By integrating waste heat recovery with energy storage and grid systems, it will be possible to create more flexible and resilient energy networks that can balance supply and demand while minimizing energy waste.
Another area for future development is in the field of advanced materials. Materials science has the potential to revolutionize waste heat recovery systems by developing more efficient heat transfer materials and improving the performance of thermoelectric generators, heat exchangers, and other components. For example, the development of nano-engineered materials could lead to more efficient thermoelectric materials that are capable of converting waste heat into electricity at much higher efficiencies. Similarly, the use of graphene and carbon nanotubes in heat exchangers could enhance thermal conductivity, allowing for more effective heat recovery in systems with challenging temperature conditions.
In conclusion, the conversion of waste heat to electricity through various recovery technologies offers significant potential for industries to reduce their energy consumption, lower costs, and minimize their environmental impact. As advancements in materials, system design, and integration continue to evolve, waste heat recovery systems will become even more efficient and versatile, making them a crucial component of the global energy landscape. From manufacturing to transportation and renewable energy systems, the ability to harness and convert waste heat into usable electricity will contribute to a more sustainable, energy-efficient, and environmentally responsible future.
Heat to Electricity Converter
A heat to electricity converter is a device or system designed to capture and convert thermal energy (heat) into electrical energy, typically from waste heat produced by industrial processes, transportation, or other energy-intensive activities. The primary goal of such a converter is to recover otherwise wasted heat and turn it into useful electrical power, improving overall energy efficiency and reducing environmental impact. Several technologies and systems are employed to achieve this conversion, each suitable for different applications, temperature ranges, and scales of operation.
One of the most widely used technologies for heat to electricity conversion is the Thermoelectric Generator (TEG). A thermoelectric generator operates on the Seebeck effect, where a temperature difference between two different conductive materials generates an electric voltage. When heat is applied to one side of the thermoelectric material, it creates an imbalance of charge carriers (electrons or holes) that moves through the material, generating electricity. The advantage of TEGs is that they have no moving parts, making them reliable and low-maintenance. They are especially effective in small-scale applications where heat gradients are small, such as recovering heat from electronics, automotive exhausts, or small industrial processes. However, TEGs generally have low conversion efficiencies, particularly when the temperature difference is small, which limits their widespread use in larger-scale applications.
For applications where waste heat is available at higher temperatures, Organic Rankine Cycle (ORC) systems are commonly used. ORCs are similar to traditional steam turbine systems but instead use organic fluids with lower boiling points than water. These fluids can vaporize and expand at lower temperatures, making ORC systems more suitable for converting waste heat into electricity from lower-grade heat sources (typically in the range of 90°C to 400°C). In an ORC system, heat from an industrial process, engine exhaust, or any other high-temperature source is used to vaporize the organic fluid. The vapor expands and drives a turbine or piston, which is connected to a generator to produce electricity. ORC systems are widely used in applications such as biomass power generation, geothermal energy plants, and industrial waste heat recovery. Their main advantage is their ability to efficiently generate electricity from lower-temperature waste heat, which would be challenging to harness using traditional steam turbines.
Another promising heat to electricity conversion technology is the Stirling engine. Stirling engines operate on a closed-loop thermodynamic cycle, where a working gas (often air or hydrogen) is alternately heated and cooled, causing it to expand and contract. This expansion drives a piston, which is connected to a generator to produce electricity. Stirling engines can be powered by any heat source, including waste heat, and are capable of operating with small temperature differences, making them suitable for a wide range of heat recovery applications. While Stirling engines have relatively high efficiency compared to thermoelectric generators, they are still less common due to their complexity, high initial costs, and mechanical moving parts, which can limit their commercial viability. Nonetheless, their ability to operate in a wide variety of settings, including solar power systems, off-grid applications, and waste heat recovery, makes them a valuable option for certain applications.
Thermophotovoltaic (TPV) cells are another emerging technology for converting heat into electricity. TPV cells work by absorbing thermal radiation, typically in the infrared spectrum, emitted by a hot object or heat source. This radiation excites electrons in the semiconductor material within the TPV cell, generating an electric current. TPV systems offer the advantage of directly converting heat radiation into electricity without needing a mechanical conversion step, which can be advantageous for high-temperature applications. While still in the experimental and development stages, TPV systems hold the potential for highly efficient heat-to-electricity conversion, especially for concentrated heat sources like industrial furnaces, incinerators, or solar thermal power plants.
Heat pumps are another type of system that can be integrated into heat to electricity conversion processes, particularly in systems where low-grade waste heat is available. A heat pump uses a refrigeration cycle to transfer heat from a cooler location to a warmer one, effectively upgrading the temperature of waste heat. This upgraded heat can then be used in systems such as Organic Rankine Cycles (ORC) or steam turbines to generate electricity. Heat pumps can be especially useful in industries where waste heat temperatures are not high enough to drive a conventional electricity-generating cycle. By efficiently raising the temperature of waste heat, heat pumps increase the feasibility of converting low-temperature waste heat into electricity.
The Rankine Cycle, traditionally used in power plants, is also applied in waste heat recovery systems. It operates by converting heat energy into mechanical energy via the expansion of a working fluid (usually water or steam). The steam drives a turbine connected to a generator, producing electricity. In waste heat recovery, an ORC system may be employed instead of a conventional Rankine Cycle, particularly in scenarios where waste heat is at lower temperatures than those typically needed for water to boil.
In some systems, heat to electricity conversion may be achieved through a combination of technologies, including combined heat and power (CHP) systems. These systems capture waste heat produced during electricity generation and use it for heating purposes. In industrial settings, this recovered heat can be used in processes such as drying, heating, or in district heating systems. While CHP systems primarily focus on delivering thermal energy, they can also be integrated with electricity-generating systems like ORCs, steam turbines, or gas turbines, further improving the overall energy efficiency of the facility.
For higher-temperature waste heat, gas turbines and combined cycle plants can be employed. Gas turbines convert high-temperature exhaust gases directly into mechanical energy, which can then drive a generator to produce electricity. Combined cycle plants further enhance efficiency by using the waste heat from the gas turbine exhaust to generate steam, which then drives a steam turbine to produce additional electricity. These systems are widely used in large-scale power generation but are also being adapted for use in industrial waste heat recovery.
Heat to electricity conversion systems are increasingly being applied in diverse industries, including manufacturing, petrochemicals, refining, and mining, where significant amounts of waste heat are produced. By implementing waste heat recovery systems, industries can not only generate electricity but also improve their overall energy efficiency, reduce emissions, and lower operational costs. These systems are particularly beneficial for industries with continuous operations and high energy consumption, as the ability to recover and convert waste heat helps offset the need for external power sources.
District heating systems are another application where heat to electricity converters can be used effectively. These systems distribute heat generated by power plants or industrial facilities to residential and commercial buildings. By integrating waste heat recovery technologies, district heating systems can offer more sustainable, efficient energy solutions, reducing reliance on conventional energy sources and improving overall system efficiency.
The future of heat to electricity converters is promising, with ongoing advancements in materials science, system design, and integration with other technologies. For example, innovations in thermoelectric materials could increase the efficiency of thermoelectric generators, while nanotechnology and quantum dot materials could lead to significant improvements in the efficiency and scalability of thermophotovoltaic cells. The development of hybrid systems, which combine heat to electricity converters with energy storage solutions or renewable energy technologies, is another area that holds great potential. By integrating energy storage, heat recovery systems could provide a steady, reliable power source even when waste heat is not constantly available, further enhancing the viability of heat-to-electricity technologies.
In conclusion, heat to electricity converters are essential components of a sustainable energy future. Technologies like thermoelectric generators, Organic Rankine Cycles, Stirling engines, and thermophotovoltaic cells offer significant potential for recovering waste heat and converting it into useful electricity. As advancements in materials and system integration continue, these technologies will become increasingly efficient, cost-effective, and widely adopted across industries and applications, contributing to a more energy-efficient and environmentally responsible world.
As we look toward the future of heat to electricity conversion, there are several key developments and trends that are likely to shape the evolution of these technologies. One of the main drivers of progress in this field is the global push for sustainability and carbon reduction. As industries and governments seek to reduce their environmental impact, heat recovery systems and electricity generation from waste heat will become increasingly important. Recovering waste heat not only enhances energy efficiency but also contributes to lowering greenhouse gas emissions, which is a crucial goal in combating climate change.
Another significant trend is the increasing integration of smart technologies and advanced control systems into heat-to-electricity conversion processes. For instance, in large industrial facilities or power plants, advanced sensors and control systems can monitor and optimize the performance of heat recovery systems in real-time. These systems can adjust to varying heat sources and environmental conditions, improving the overall efficiency of the energy conversion process. With the rise of the Industrial Internet of Things (IIoT), it’s now possible to remotely monitor and manage these systems, making them more adaptable and cost-effective. Predictive analytics and machine learning could also play a role in forecasting when waste heat is most available, allowing systems to be better synchronized with energy demand and storage.
The expansion of distributed energy systems is also a critical factor in the future of heat-to-electricity conversion. In addition to large-scale applications, there is a growing interest in small and medium-sized energy recovery systems that can be implemented locally. For instance, buildings, industrial sites, and even residential areas can incorporate waste heat recovery technologies, turning their waste energy into usable power. These decentralized systems can be particularly valuable in remote areas or off-grid locations, where traditional power generation methods are not feasible or reliable. Furthermore, with the increasing adoption of microgrids and localized energy systems, integrating waste heat recovery into these smaller-scale systems could help improve energy security, reduce transmission losses, and lower electricity costs for end users.
In terms of material innovation, the development of advanced thermoelectric materials is expected to lead to more efficient thermoelectric generators. Materials such as nanomaterials, carbon nanotubes, and graphene have shown promise in improving the performance of thermoelectric devices by enhancing heat conductivity and electrical properties. These materials could potentially lead to significant improvements in the efficiency of thermoelectric generators, making them viable for use in larger-scale industrial applications and reducing the cost per watt of electricity generated from waste heat. Similarly, research into high-temperature thermoelectrics is enabling the development of thermoelectric materials that can withstand the extreme temperatures often found in industrial and power plant environments, broadening the potential for their use in these applications.
Another exciting area of development is the potential for hybrid systems that combine heat-to-electricity conversion with other renewable energy sources or storage technologies. For example, integrating solar power with waste heat recovery systems could create a more efficient and reliable energy generation system. In such hybrid systems, the waste heat captured from industrial processes could complement the energy produced by solar panels, ensuring a continuous supply of electricity even during periods of low sunlight. Similarly, battery storage systems could be integrated into heat-to-electricity conversion systems, allowing excess electricity generated during periods of high waste heat availability to be stored and used later when energy demand is higher.
The adoption of thermal energy storage is also likely to play a critical role in improving the effectiveness of waste heat recovery systems. Heat storage technologies, such as molten salt or phase-change materials, can store excess heat generated by industrial processes and release it when needed. This can help smooth out fluctuations in energy generation and demand, making waste heat recovery systems more flexible and adaptable to varying conditions. For instance, waste heat stored during low-demand periods can be used to generate electricity during peak hours or when other energy sources are not available. This could be especially beneficial in applications where heat is produced intermittently, such as in manufacturing or power generation.
On a larger scale, the integration of waste heat recovery into district energy systems is another trend that could reshape the future of heat-to-electricity conversion. District energy systems, which provide heating and cooling to multiple buildings or neighborhoods, can benefit from integrating waste heat recovery technologies. For example, industrial plants, power plants, or data centers that produce excess heat can use this energy to supply district heating systems, while also converting some of the waste heat into electricity for the local grid. This type of energy system would not only make use of otherwise wasted thermal energy but also reduce the overall demand for primary energy sources, such as fossil fuels, by recycling waste heat.
Global regulations on energy efficiency and carbon emissions are also expected to drive the adoption of heat-to-electricity technologies. As countries around the world set more ambitious carbon neutrality goals, industries will increasingly be required to minimize their energy consumption and reduce emissions. The incorporation of waste heat recovery systems into industrial processes and power generation will become essential for meeting these regulatory standards. In many cases, governments may offer financial incentives, tax credits, or grants to encourage the installation of heat-to-electricity conversion technologies, helping to lower the upfront costs and accelerate the adoption of these systems.
In the transportation sector, waste heat recovery systems are likely to become more common in both electric vehicles (EVs) and traditional internal combustion engine vehicles. As EVs become more popular, the demand for lightweight and efficient power generation systems will increase. Waste heat recovery systems, such as thermoelectric generators, could be used to power auxiliary systems like air conditioning, lighting, or infotainment systems, improving the overall efficiency of electric vehicles. For internal combustion engine vehicles, recovery systems could capture heat from exhaust gases and convert it into electrical energy to supplement the power supply and reduce fuel consumption.
In shipping, as more stringent regulations on carbon emissions and fuel efficiency are enacted, the integration of waste heat recovery systems will become a key strategy to meet these standards. Technologies like thermoelectric generators, ORC systems, and heat exchangers can be used to recover energy from engine exhausts, reducing fuel consumption and cutting down on emissions. Waste heat recovery could help maritime companies reduce operating costs while complying with international emission regulations, making it an increasingly important technology in the shipping industry.
In conclusion, the future of heat-to-electricity conversion is promising, with advancements in material science, system integration, and global energy policies driving innovation in this field. As industries and governments continue to prioritize sustainability and energy efficiency, heat recovery systems will play an increasingly vital role in transforming waste heat into valuable electricity. The adoption of new materials, smart technologies, and hybrid systems will expand the potential applications of these systems, from industrial manufacturing to transportation and even residential energy use. With continued development, heat-to-electricity converters have the potential to revolutionize the way we recover and utilize thermal energy, contributing to a more sustainable, energy-efficient future.
Most Efficient Way to Convert Heat to Electricity
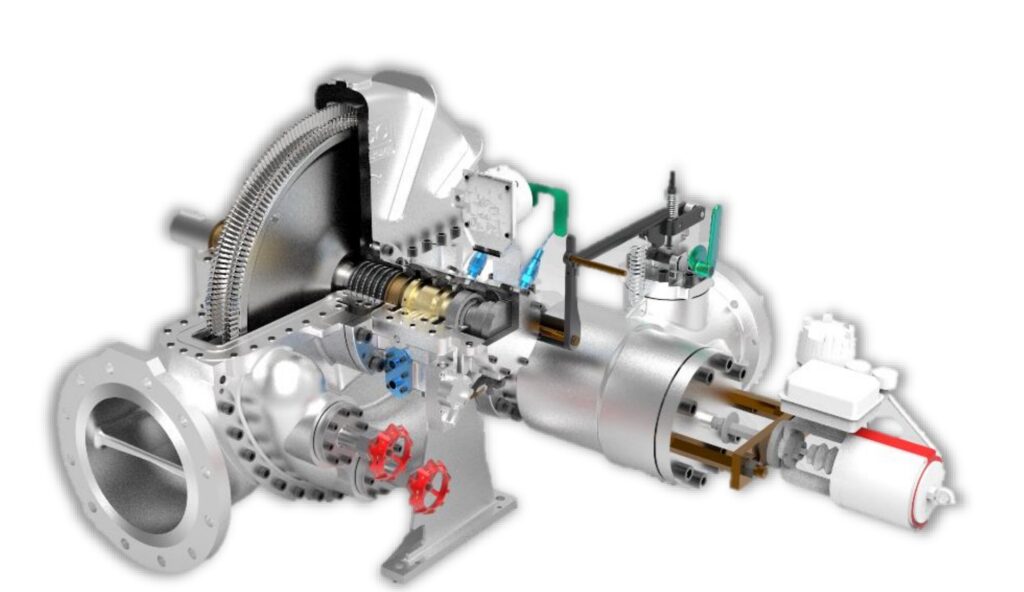
The most efficient way to convert heat to electricity depends on several factors, including the temperature of the heat source, the scale of the application, and the specific requirements of the system. While there are a variety of technologies available, each with its own strengths and limitations, several key methods have emerged as the most efficient for converting heat to electricity across different temperature ranges and applications.
1. Organic Rankine Cycle (ORC): The Organic Rankine Cycle is considered one of the most efficient methods for converting lower to medium-temperature waste heat (typically between 90°C and 400°C) into electricity. ORC systems are based on a similar principle to steam turbines, where a working fluid is heated by waste heat, causing it to vaporize and expand. The vapor then drives a turbine connected to a generator. The main advantage of ORC over traditional steam turbines is that it uses organic fluids (such as refrigerants or hydrocarbons) with lower boiling points than water, enabling efficient operation at lower temperatures. ORC systems are widely used in waste heat recovery applications, geothermal power plants, biomass power generation, and combined heat and power (CHP) systems. The efficiency of ORC systems improves with higher temperature differences and is particularly effective in industrial processes where waste heat is not hot enough for traditional steam turbines.
2. Thermoelectric Generators (TEGs): Thermoelectric generators are solid-state devices that use the Seebeck effect to convert heat directly into electricity. A temperature difference between two materials (typically a semiconductor) generates an electric voltage, which can be harvested as electrical energy. While thermoelectric generators have low conversion efficiencies (typically around 5% to 10%), they are often considered efficient for small-scale applications where other methods may not be feasible. TEGs are suitable for applications where there is a constant or low-grade heat source, such as in automotive exhaust recovery, electronics cooling, or remote sensors. Advances in thermoelectric materials, such as nanomaterials and graphene, are improving the efficiency of TEGs, making them more viable for a broader range of applications in the future. Although they are not the most efficient in terms of raw energy conversion, their ability to operate without moving parts, and their suitability for small-scale, low-temperature applications, make TEGs a useful tool in certain scenarios.
3. Stirling Engines: Stirling engines operate on a closed-cycle thermodynamic process, where a working gas is alternately heated and cooled, causing it to expand and contract. This expansion drives a piston that can generate mechanical power, which is then converted into electricity. Stirling engines are particularly efficient for applications where a small to medium temperature difference exists, as they can operate with both high and low-temperature heat sources. They can achieve higher efficiencies (up to 40%) compared to traditional steam engines in the right conditions. Stirling engines are typically used in solar power plants, remote power generation, and combined heat and power (CHP) systems. The efficiency of Stirling engines increases with larger temperature differences, but their complex design, high costs, and the need for a heat source with significant temperature gradients can limit their widespread use.
4. Thermophotovoltaic (TPV) Cells: Thermophotovoltaic cells convert heat into electricity by using semiconductor materials to absorb infrared radiation emitted by hot surfaces. The absorbed radiation excites electrons in the semiconductor material, generating electricity. TPV cells can operate at high temperatures (greater than 500°C), making them an efficient option for high-temperature heat sources, such as industrial furnaces or concentrated solar power plants. Although TPVs are still in the development stage, they hold the potential for high efficiencies in specific applications. Research in this field focuses on improving the efficiency of the semiconductor materials used in TPV cells, as well as the overall system design. TPVs are still not as widely deployed as other heat-to-electricity technologies, but they show promise for converting waste heat from high-temperature processes into useful electrical power.
5. Combined Cycle Power Plants (Gas and Steam Turbines): Combined cycle plants represent one of the most efficient ways to convert heat to electricity, especially in large-scale power generation. These plants use both gas turbines and steam turbines in a complementary manner. In a combined cycle system, gas turbines burn fuel (such as natural gas) to generate electricity. The hot exhaust gases from the gas turbine are then used to heat water in a heat recovery steam generator (HRSG), producing steam that drives a steam turbine. The steam turbine generates additional electricity, increasing the overall efficiency of the system. Combined cycle plants can achieve efficiencies exceeding 60%, making them one of the most efficient methods for large-scale electricity generation. While these plants require a high initial investment, they are commonly used in modern power stations due to their high efficiency and relatively low operational costs.
6. Waste Heat Recovery Boilers: Waste heat recovery boilers capture excess heat from industrial processes or power plants that would otherwise be wasted and convert it into usable steam. This steam can be used for electricity generation, heating, or even industrial processes. In waste heat recovery boilers, hot gases from processes like cement production, steel manufacturing, or chemical refining pass through a series of heat exchangers that transfer the heat to water, turning it into steam. The steam can then drive a turbine to generate electricity. This method is particularly effective in industries with high levels of waste heat, such as refining, cement manufacturing, and steel production. By recovering and converting this waste heat, companies can reduce fuel consumption, lower energy costs, and decrease their environmental impact.
7. Heat Pumps and Integrated Systems: Heat pumps are devices that transfer heat from a lower temperature source (such as ambient air or waste heat) to a higher temperature sink, using a refrigeration cycle. In heat-to-electricity systems, heat pumps can be used to elevate the temperature of waste heat to a level where it can be efficiently converted into electricity, typically by an Organic Rankine Cycle (ORC) or a steam turbine. In industrial or commercial settings, integrating heat pumps with waste heat recovery systems can enhance the overall efficiency of heat-to-electricity conversion by improving the temperature of the waste heat source. While not directly generating electricity on their own, heat pumps play a crucial role in enabling other systems (like ORCs or steam turbines) to operate more efficiently.
8. Hybrid Systems and Energy Storage: Hybrid systems that combine multiple technologies, such as ORC with thermoelectric generators or heat pumps with Stirling engines, can increase overall efficiency by utilizing various heat recovery methods depending on the temperature of the waste heat available. In addition, integrating energy storage technologies such as batteries or thermal storage systems can help smooth out the intermittent availability of waste heat, ensuring a consistent supply of electricity even when heat is not continuously available. By combining heat recovery with energy storage, these systems can generate electricity on demand and provide backup power during periods of low waste heat availability.
In terms of raw efficiency, combined cycle power plants and organic Rankine cycle (ORC) systems currently stand as the most efficient methods for large-scale heat-to-electricity conversion. However, in smaller or lower-temperature applications, thermoelectric generators and Stirling engines offer promising solutions, with ongoing research improving their efficiency. The choice of technology largely depends on the specifics of the heat source, the temperature range, the scale of the system, and the economic considerations.
Conclusion: The most efficient way to convert heat to electricity depends on the conditions and requirements of the application. For large-scale, high-temperature applications, combined cycle plants and ORC systems are typically the most efficient. For smaller-scale, lower-temperature applications, technologies like thermoelectric generators, Stirling engines, and waste heat recovery boilers are more suitable. Ongoing advancements in materials science, system integration, and hybrid technologies are expected to increase the efficiency and viability of these systems, expanding their use in diverse applications and helping to drive a more sustainable energy future.
As the demand for energy-efficient technologies continues to grow, the development of new and advanced methods for converting heat to electricity is expected to accelerate. In particular, the push for more sustainable and low-carbon energy solutions will likely drive innovation in heat-to-electricity conversion technologies, allowing for greater efficiency, cost-effectiveness, and broader adoption across various industries.
Advancements in Thermoelectric Materials: One of the most promising areas of research is the development of new thermoelectric materials. The efficiency of thermoelectric generators (TEGs) is largely determined by the properties of the materials used to convert heat into electricity. Traditional thermoelectric materials, such as bismuth telluride, are limited in their performance, particularly in high-temperature environments. However, researchers are making significant strides in developing nanostructured materials, quantum dots, and thermoelectric polymers that could dramatically increase the efficiency of TEGs. These materials offer greater flexibility, higher thermoelectric efficiency, and improved performance in both high and low-temperature applications. For example, graphene-based materials are being explored for their potential to improve both electrical and thermal conductivity, enhancing the performance of thermoelectric devices.
Waste Heat Recovery in the Automotive Industry: As the automotive industry shifts towards electric vehicles (EVs) and strives for greater efficiency in internal combustion engine (ICE) vehicles, waste heat recovery systems will become increasingly important. Electric vehicles already benefit from regenerative braking systems that convert kinetic energy back into electricity, but recovery of heat from the motor and other components can further boost efficiency. For ICE vehicles, integrating waste heat recovery systems into the exhaust system, such as using thermoelectric generators to capture heat from exhaust gases, can reduce fuel consumption and improve fuel efficiency. Similarly, hybrid systems that combine heat recovery technologies with electric powertrains in vehicles could offer higher overall performance and lower operating costs.
Integration with Renewable Energy Systems: Another significant advancement will be the integration of heat-to-electricity systems with renewable energy sources such as solar, wind, and geothermal. For instance, solar thermal power plants could use waste heat recovery technologies to capture excess heat during periods of high solar availability and convert it into electricity. This would ensure continuous power generation even when solar radiation is low. Similarly, wind farms could use waste heat recovery to capture and convert thermal energy generated by turbines or electrical components into additional electricity. Geothermal energy, known for its constant heat output, could also benefit from integrating heat-to-electricity conversion systems, particularly with innovations like enhanced geothermal systems (EGS) that seek to tap into deeper, hotter reservoirs.
Increased Efficiency in Industrial and Commercial Applications: Many industries already use waste heat recovery to improve their energy efficiency. For example, cement kilns, steel plants, and refineries are prime candidates for waste heat recovery due to the vast amounts of thermal energy they produce. By using heat recovery systems, these industries can significantly reduce their reliance on external energy sources and lower their carbon footprint. The next step in this evolution will involve the deployment of more integrated systems that combine waste heat recovery with energy storage and advanced control systems. These systems will not only recover and convert heat but also store it for later use, increasing the flexibility and efficiency of the entire energy system.
Thermal Energy Storage and Grid Stability: As renewable energy generation becomes more prevalent, managing the intermittent nature of these sources—particularly solar and wind—becomes crucial. Thermal energy storage systems can help mitigate this problem by storing excess heat generated during periods of high renewable energy production and releasing it when demand is high or when renewable generation is low. Waste heat recovery systems can be coupled with thermal storage systems to provide a reliable, dispatchable source of energy. This integration could play a key role in stabilizing the grid, reducing reliance on fossil fuel-based power plants, and improving the efficiency of energy distribution networks.
Industrial Waste Heat to Power Systems: In industrial manufacturing, waste heat is often a byproduct of high-temperature processes like metal smelting, glass production, and chemical processing. Converting this heat into usable electricity is a key opportunity for reducing operational costs and energy consumption. Modular waste heat recovery systems that can be scaled to fit specific industrial processes will become increasingly common. These systems will incorporate a mix of technologies, such as ORC, thermoelectric generators, and heat exchangers, to maximize the efficiency of heat recovery and electricity generation. The use of modular and flexible heat-to-electricity systems will allow industries to adapt waste heat recovery to their specific needs, making it easier for companies of all sizes to implement these solutions.
Energy-Positive Buildings and Microgrids: The concept of energy-positive buildings—structures that generate more energy than they consume—can be realized by integrating advanced heat-to-electricity conversion systems with microgrids and distributed energy systems. In such buildings, waste heat from HVAC systems, industrial equipment, and even domestic appliances can be captured and converted into electricity, reducing overall energy consumption and enabling buildings to become self-sustaining. By incorporating these systems into smart grids, energy can be distributed and stored more efficiently, optimizing both energy consumption and generation. Energy-positive communities could emerge, where a network of buildings, homes, and businesses work together to produce and consume energy in a more sustainable and efficient manner.
Policy and Economic Incentives: Governments around the world are implementing policies to encourage energy efficiency and the use of waste heat recovery technologies. Policies such as carbon pricing, renewable energy mandates, and incentives for energy-efficient technologies are driving industries to adopt heat-to-electricity conversion systems. Additionally, governments may offer subsidies or grants to offset the initial costs of installing waste heat recovery systems, particularly in industries where energy costs are high and operational efficiency is critical. As these technologies become more cost-competitive, they will likely see increased adoption across various sectors, further pushing the transition to cleaner, more efficient energy systems.
Conclusion: The most efficient ways to convert heat to electricity are continually evolving as technology advances. From large-scale systems like combined-cycle power plants to smaller solutions such as thermoelectric generators and Stirling engines, the range of methods continues to expand. The development of new materials, the integration of energy storage, and the coupling of heat-to-electricity systems with renewable energy sources and smart grids will further improve efficiency and make these systems more versatile. The growing demand for sustainable energy solutions, combined with advancements in technology and favorable economic policies, will continue to drive the adoption of heat-to-electricity conversion methods, helping to meet global energy needs while reducing environmental impact. As innovations in this field progress, we can expect to see more widespread use of waste heat recovery technologies in industrial, commercial, and residential settings, contributing to a cleaner, more efficient energy future.
Exhaust Heat Recovery System
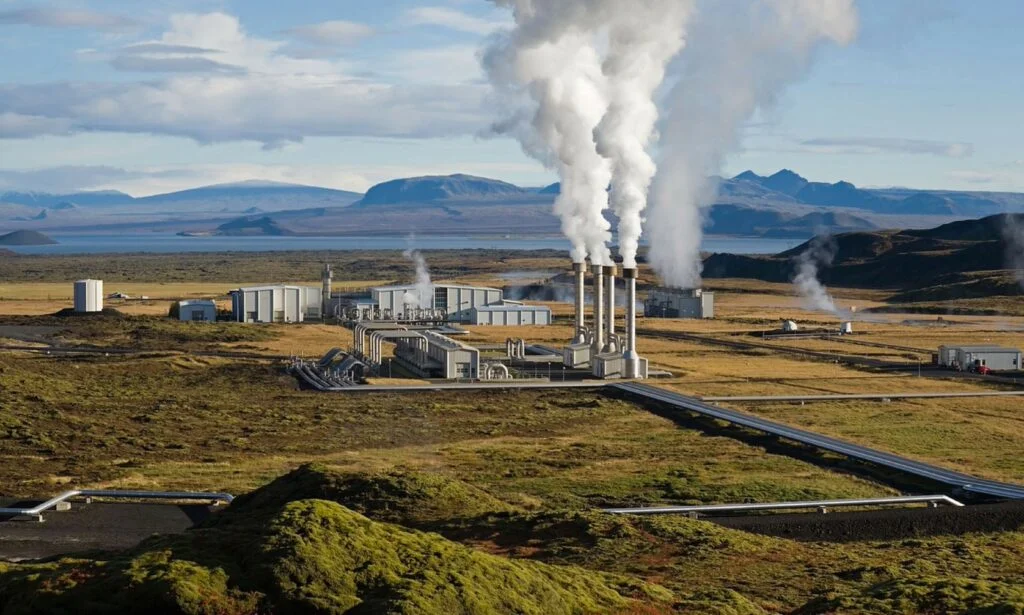
Exhaust heat recovery systems are designed to capture and utilize the thermal energy present in the exhaust gases produced by industrial processes, power plants, and vehicles. This recovered heat is typically wasted, yet it holds significant potential for improving the overall energy efficiency of a system. By capturing this heat and converting it into usable energy, exhaust heat recovery systems can reduce fuel consumption, lower emissions, and increase operational efficiency. These systems are crucial for industries and applications where high-temperature exhaust gases are generated, such as power generation, chemical manufacturing, steel production, and automotive systems.
Exhaust gases, especially from combustion processes, typically contain a large amount of thermal energy. In many industrial and commercial applications, this heat is often released into the atmosphere without any beneficial use. Exhaust heat recovery systems aim to capture this waste heat and repurpose it for useful applications, such as preheating air or water, generating electricity, or even directly powering turbines or other mechanical systems. By integrating such systems into industrial processes or power generation facilities, significant energy savings can be achieved.
One common approach to exhaust heat recovery is the use of heat exchangers, which transfer the heat from the exhaust gases to a fluid (usually water or air). In many cases, these heat exchangers are part of a larger system that includes heat recovery steam generators (HRSGs), which convert the recovered heat into steam that can then be used to drive a turbine for power generation. This process is typically seen in combined-cycle power plants, where a gas turbine and steam turbine are used in tandem to maximize the overall efficiency of the system.
Another common technology is the Organic Rankine Cycle (ORC), which is often used for lower temperature exhaust heat recovery applications. The ORC operates similarly to a traditional Rankine cycle (used in steam turbines), but instead of water, it uses organic fluids with lower boiling points, making it ideal for recovering heat from exhaust gases that are not hot enough to produce steam. The ORC can then convert this heat into mechanical power, which can be used to generate electricity. This method is particularly popular in industries such as biomass energy, geothermal power, and waste-to-energy plants, where exhaust heat recovery is an integral part of the energy production process.
In the automotive industry, exhaust heat recovery plays a significant role in improving vehicle efficiency. In internal combustion engine (ICE) vehicles, the exhaust gases are a major source of energy loss. However, advanced technologies such as thermoelectric generators (TEGs) and Stirling engines are being developed to capture heat from the exhaust system and convert it into electricity. In these systems, the exhaust gases are passed through thermoelectric materials or a Stirling engine, which converts the heat directly into electrical power. This recovered electricity can then be used to charge the vehicle’s battery or power auxiliary systems, reducing the need for additional fuel consumption.
In marine applications, exhaust heat recovery is essential for improving fuel efficiency and reducing the environmental impact of ships and vessels. The heat from the exhaust gases of marine engines can be captured and used to produce steam, which can then be used for propulsion or to generate electricity. This process, often seen in combined heat and power (CHP) systems aboard ships, helps to significantly reduce fuel consumption, lower operating costs, and minimize emissions. Moreover, using exhaust heat recovery in marine vessels can extend the operational range of ships while reducing the need for fuel-intensive auxiliary power systems.
Industrial applications also benefit from exhaust heat recovery systems. For example, in cement production, the heat from the exhaust gases produced during the kiln process can be recovered and used to preheat the incoming raw materials or generate steam. Similarly, in steel manufacturing, exhaust heat recovery can be applied to blast furnaces and electric arc furnaces, reducing energy consumption and improving overall plant efficiency. In these settings, exhaust heat recovery can reduce reliance on external energy sources, lower operational costs, and make these processes more sustainable by reducing their carbon footprint.
One of the key advantages of exhaust heat recovery systems is their ability to reduce the overall environmental impact of industrial processes. By recovering waste heat and converting it into usable energy, these systems help to reduce fuel consumption, lower greenhouse gas emissions, and minimize the need for additional power generation. In many cases, the recovered heat can be used to supplement the system’s existing energy needs, which leads to a more efficient operation overall.
Exhaust heat recovery systems also contribute to operational cost savings. By recovering and reusing heat that would otherwise be wasted, industries can reduce their energy costs and improve the profitability of their operations. In some cases, these systems can pay for themselves over time through fuel savings, tax credits, and other incentives. Moreover, the recovery of exhaust heat can lead to increased process reliability by maintaining more consistent temperatures within critical systems, such as furnaces or kilns.
In addition to the environmental and economic benefits, exhaust heat recovery systems also improve energy resilience. In industries where energy demand is high and the availability of fuel is uncertain, recovering waste heat provides an additional source of energy that can be relied upon during peak demand periods or when external energy supplies are disrupted. This increases the overall stability of the system and allows for greater operational flexibility.
The potential applications for exhaust heat recovery systems are vast and continue to expand. In addition to their traditional use in industrial settings, exhaust heat recovery systems are being deployed in new sectors, such as renewable energy, waste-to-energy plants, and district heating systems. Moreover, the development of new technologies and materials is expected to improve the efficiency and feasibility of exhaust heat recovery in a wider range of applications, making it an essential component of the transition toward more sustainable and energy-efficient systems.
Conclusion: Exhaust heat recovery systems are an integral part of improving energy efficiency in industrial, commercial, and transportation sectors. By capturing and converting waste heat from exhaust gases, these systems reduce fuel consumption, lower emissions, and increase operational efficiency. Whether through technologies like heat exchangers, Organic Rankine Cycles (ORC), thermoelectric generators (TEGs), or Stirling engines, exhaust heat recovery systems are proving to be a critical tool for energy recovery in diverse applications, including power generation, industrial manufacturing, marine industry, and transportation. As new technologies and materials continue to emerge, the efficiency and effectiveness of exhaust heat recovery systems will improve, allowing them to play an even greater role in achieving global energy sustainability goals.
The adoption and implementation of exhaust heat recovery systems are expected to grow significantly as industries and businesses strive for greater energy efficiency, cost savings, and environmental responsibility. With increasing pressure to reduce greenhouse gas emissions and meet stringent regulatory standards, the recovery and reuse of waste heat present an excellent opportunity to cut down on both operational costs and carbon footprints.
One of the key drivers for the widespread adoption of exhaust heat recovery technologies is the growing emphasis on sustainability. Governments around the world are tightening regulations concerning emissions and energy consumption, pushing industries to adopt greener practices. The Paris Agreement and various local environmental policies encourage companies to implement energy recovery solutions that help reduce their overall environmental impact. Companies that invest in these systems are not only improving their bottom line through energy savings but also positioning themselves as responsible and sustainable players in the market. Many countries offer tax incentives, subsidies, or grant programs to businesses that incorporate waste heat recovery systems, which further accelerates the adoption of these technologies.
The integration of exhaust heat recovery with advanced monitoring systems is another area that holds great promise for increasing the overall efficiency of energy recovery processes. By implementing sensors and data analytics, companies can closely monitor the amount of waste heat produced and determine the most efficient ways to capture and reuse it. This real-time monitoring can also help optimize system performance, ensure that the recovery process is running at peak efficiency, and reduce downtime for maintenance. Over time, as businesses become more adept at integrating smart technologies into their operations, the recovery of exhaust heat will become even more seamless and automated, allowing for more flexibility and adaptability to varying operating conditions.
Digitalization and Artificial Intelligence (AI) also offer opportunities to optimize exhaust heat recovery systems. AI algorithms can be used to predict demand for recovered energy and optimize the operation of heat recovery systems in real time. This could involve dynamically adjusting heat exchangers, ORCs, or other components based on environmental conditions, production schedules, and energy demand. The integration of Internet of Things (IoT) devices with these systems also allows for more accurate control, maintenance, and performance monitoring, reducing the risk of system failures and ensuring that recovered heat is put to optimal use.
Energy storage technologies could also work in tandem with exhaust heat recovery systems to further enhance their efficiency. Heat storage solutions, such as phase change materials or thermal energy storage (TES) systems, can store recovered heat during periods of low demand and release it when it is needed. This allows for a more flexible energy system, as recovered heat can be used during peak load periods or when demand for electricity is high. The ability to store excess heat for later use could be particularly beneficial in industries that experience intermittent or fluctuating energy needs, like food processing, chemical manufacturing, and cement production.
As exhaust heat recovery systems become more widespread, there is also an increasing focus on modular designs that can be easily scaled to fit different applications. Many industries, particularly small and medium-sized enterprises (SMEs), are seeking cost-effective and easily deployable heat recovery solutions. Modular systems, which can be adapted to various types of processes, equipment, and energy needs, allow companies to start with small installations and gradually expand them as their operations grow or energy demands increase. These modular solutions also reduce installation costs and minimize the disruption to ongoing operations, making them a more attractive option for businesses.
The marine industry is another area where exhaust heat recovery systems are poised for significant growth. Modern shipping fleets are increasingly focusing on reducing their fuel consumption and carbon emissions. Exhaust gas recirculation systems (EGR), used in combination with exhaust heat recovery systems, can help ships recover heat from exhaust gases to generate electricity or provide additional energy for auxiliary systems. In addition to reducing fuel costs, these systems also contribute to emission reduction, helping ships meet increasingly strict international emissions standards. Additionally, the growth of electrification in maritime vessels could create even greater demand for waste heat recovery systems, as these ships rely on advanced energy storage and power conversion systems that can benefit from recovered exhaust heat.
Waste heat recovery also plays an essential role in district heating systems, especially in urban areas where large amounts of heat are wasted in industrial zones, power stations, or waste-to-energy plants. District heating networks, which provide centralized heating to residential, commercial, and industrial buildings, can significantly benefit from exhaust heat recovery. By using heat recovery systems to capture waste heat from industrial processes or power plants, this recovered energy can be used to heat buildings or even generate additional electricity. This approach contributes to the overall energy efficiency of cities and reduces reliance on fossil fuels for heating purposes.
In waste-to-energy plants, where energy is generated from the combustion of waste materials, the use of exhaust heat recovery systems is critical. The combustion process in these plants generates high-temperature exhaust gases that are often not fully utilized. By recovering this heat, these systems can generate additional power or preheat incoming waste, improving overall plant efficiency. With the global push towards reducing landfill waste and promoting more sustainable waste management practices, waste-to-energy plants are becoming increasingly important, and integrating exhaust heat recovery systems makes these plants even more energy-efficient and environmentally friendly.
The future of exhaust heat recovery is bright, with numerous opportunities for innovation and improvement. As new materials, technologies, and applications continue to emerge, the potential for harnessing waste heat will only expand. The development of next-generation heat exchangers, thermoelectric generators, and advanced heat storage technologies will continue to enhance the efficiency of these systems, allowing for greater energy recovery and less reliance on traditional energy sources. Additionally, as the world transitions towards more sustainable energy solutions, industries will increasingly view exhaust heat recovery not just as a way to improve energy efficiency but as a critical component of their efforts to reduce emissions and achieve long-term sustainability goals.
In conclusion, exhaust heat recovery systems are a vital technology for improving energy efficiency, reducing environmental impact, and increasing sustainability across a wide range of industries. By capturing and utilizing the thermal energy from exhaust gases, these systems offer significant opportunities for reducing fuel consumption, lowering emissions, and enhancing operational performance. Whether in industrial manufacturing, power generation, automotive systems, marine applications, or waste-to-energy plants, exhaust heat recovery systems are becoming an integral part of the global transition to a more energy-efficient and sustainable future. As technological advancements continue to emerge, the efficiency and scope of exhaust heat recovery will expand, making it a key tool for tackling global energy and environmental challenges.
Exhaust Gas Heat Recovery Power Generation System
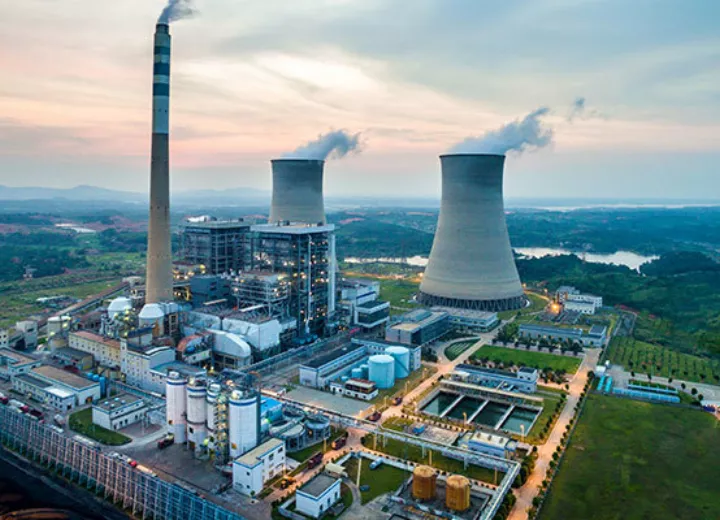
Exhaust gas heat recovery power generation systems are designed to capture and utilize the thermal energy present in exhaust gases, often from industrial processes or power plants, to generate additional power. This system focuses on harnessing waste heat that would otherwise be released into the environment and converting it into usable electrical energy. By recovering exhaust heat, these systems significantly improve overall energy efficiency, reduce fuel consumption, and lower greenhouse gas emissions. Exhaust gas heat recovery power generation is an essential technology in various industries, including power generation, chemical manufacturing, steel production, and transportation, as it helps optimize energy use and supports sustainable practices.
Exhaust gases are typically produced by combustion processes, such as those found in gas turbines, internal combustion engines, or industrial boilers. These gases are hot and contain substantial thermal energy, which, if left unutilized, is wasted to the atmosphere. In an exhaust gas heat recovery system, the exhaust gases are captured and directed through a heat exchanger, which transfers the thermal energy from the gases to a working fluid, such as water or air. The working fluid is then used to generate steam, which can drive a steam turbine or power other energy systems. This heat recovery process not only helps recover otherwise wasted energy but also boosts the overall efficiency of power generation systems by supplementing the energy required for electricity production.
One of the most common technologies used in exhaust gas heat recovery for power generation is the heat recovery steam generator (HRSG). HRSGs are used in combined-cycle power plants, where they recover heat from the exhaust of gas turbines and use it to generate steam. The steam is then fed to a steam turbine, which generates additional electricity. The combination of a gas turbine and a steam turbine in a single system allows for much higher efficiency compared to conventional power plants that only use one type of turbine. In this system, the gas turbine generates electricity by burning fuel, while the steam turbine generates additional power from the heat recovery process.
Another technology commonly used in exhaust gas heat recovery power generation systems is the Organic Rankine Cycle (ORC). The ORC operates similarly to a traditional Rankine cycle, which is used in steam turbines. However, instead of water, it uses organic fluids with lower boiling points, making it more suitable for recovering heat from exhaust gases that are not hot enough to produce steam. The ORC works by circulating an organic fluid through an evaporator, where it absorbs the heat from the exhaust gases and turns into vapor. This vapor is then expanded through a turbine, generating mechanical power that is converted into electricity. ORC systems are particularly useful in applications where exhaust gases are at lower temperatures, such as in biomass energy systems, geothermal power plants, or waste-to-energy plants.
In automotive applications, exhaust gas heat recovery power generation systems can significantly improve the efficiency of vehicles, especially heavy-duty trucks and buses, which produce substantial amounts of exhaust heat. Advanced technologies such as thermoelectric generators (TEGs) and Stirling engines are used to convert exhaust heat into electricity. TEGs utilize the Seebeck effect, where a temperature difference between two materials generates an electric voltage. By attaching these generators to the vehicle’s exhaust system, the exhaust heat can be converted directly into electricity, which can then be used to charge the vehicle’s battery or power auxiliary systems, such as lights and air conditioning, thereby reducing the load on the engine and improving fuel efficiency.
Marine industry applications also benefit from exhaust gas heat recovery power generation systems. Ships and marine vessels rely on large engines that produce significant exhaust heat, which can be captured and used to generate additional power. This is often achieved using heat recovery steam generators (HRSGs) or organic Rankine cycle (ORC) systems to recover heat from the exhaust gases of marine engines. The recovered energy can be used for onboard power generation, helping ships reduce their fuel consumption and emissions. This is particularly important in light of increasing regulations around emissions from maritime vessels, with stringent international standards being imposed to reduce sulfur oxide (SOx), nitrogen oxide (NOx), and carbon dioxide (CO2) emissions from ships.
Waste-to-energy plants are another key area where exhaust gas heat recovery power generation systems play a crucial role. In these plants, waste materials such as municipal solid waste (MSW) are burned to produce electricity. The combustion process generates high-temperature exhaust gases, which are typically sent through a heat recovery system to generate steam. This steam can then be used to drive a steam turbine and generate additional electricity. The incorporation of heat recovery systems in waste-to-energy plants improves energy efficiency by utilizing the thermal energy from exhaust gases and reduces the reliance on external power sources. Additionally, waste-to-energy plants can contribute to the circular economy by diverting waste from landfills while simultaneously producing electricity from otherwise discarded materials.
In industrial applications, exhaust gas heat recovery power generation systems are commonly used in large-scale operations such as cement manufacturing, steel production, and chemical processing. In these industries, high-temperature exhaust gases are generated from furnaces, kilns, and other energy-intensive equipment. By integrating heat recovery systems, these industries can recover a significant portion of the thermal energy and convert it into electricity. For example, in cement plants, the high-temperature exhaust gases produced during the calcination process can be captured by a heat recovery system and used to generate steam or preheat the raw materials, improving the overall energy efficiency of the plant. In steel manufacturing, exhaust heat recovery systems can be applied to blast furnaces and electric arc furnaces, reducing the need for external energy inputs and lowering operational costs.
The overall benefits of exhaust gas heat recovery for power generation are numerous. Firstly, it leads to substantial fuel savings, as the recovered heat reduces the need for additional fuel to generate electricity. This is particularly important for industries with high energy consumption, such as chemical manufacturing and refining, where energy costs constitute a significant portion of operating expenses. By improving the efficiency of power generation, these systems also help reduce the carbon footprint of industrial operations, making them more sustainable and aligned with global climate change mitigation goals.
Moreover, the use of exhaust gas heat recovery systems increases energy resilience by providing an additional source of power generation that is independent of external energy supplies. This is particularly valuable in remote locations or areas where energy reliability is a concern. For example, in industrial plants located in off-grid regions, exhaust gas recovery systems can provide a steady supply of electricity, reducing dependence on external power sources and enhancing operational continuity.
In addition to fuel savings and emission reductions, exhaust gas heat recovery power generation systems can also lead to improved operational efficiency. By utilizing the heat that is naturally generated in industrial processes, these systems help companies operate more efficiently and avoid wasting valuable energy. In many cases, these systems can be integrated with existing infrastructure with minimal disruption to ongoing operations, making them an attractive option for businesses looking to upgrade their energy systems without significant capital investment.
The future of exhaust gas heat recovery power generation systems looks promising as technological advancements continue to improve their efficiency and applicability. New materials, such as high-temperature heat exchangers and advanced thermoelectric materials, are being developed to enhance the performance of these systems, particularly in industries where exhaust gases are at high temperatures. Furthermore, smart grid technologies and advanced control systems are being incorporated into heat recovery systems to optimize their operation, further improving efficiency and allowing for greater integration with renewable energy sources.
In conclusion, exhaust gas heat recovery power generation systems represent a vital technology for improving energy efficiency, reducing fuel consumption, and lowering emissions across a variety of industries. Whether in power generation, chemical manufacturing, automotive applications, or marine vessels, these systems allow for the recovery and conversion of otherwise wasted thermal energy into usable electricity. As technologies evolve, the efficiency of these systems will improve, contributing to more sustainable industrial practices and supporting the transition to a cleaner, more energy-efficient future.
As the demand for sustainable energy solutions continues to rise, exhaust gas heat recovery power generation systems are becoming an increasingly important part of global efforts to reduce reliance on fossil fuels and lower carbon emissions. Industries are under growing pressure to meet environmental regulations and to adopt greener technologies. Integrating heat recovery systems into industrial operations offers one of the most effective ways to meet these challenges while maintaining profitability. The global transition to a low-carbon economy, coupled with the rapid advancement of technologies, promises a significant expansion of exhaust gas heat recovery applications in the coming years.
In the context of smart manufacturing and industry 4.0, exhaust gas heat recovery systems are becoming more interconnected and intelligent. Sensors, data analytics, and machine learning algorithms allow for real-time monitoring of exhaust gas temperatures, flow rates, and system efficiency. This data is used to optimize the recovery process, ensuring that the system is operating at peak performance and that energy is being recovered in the most efficient manner possible. These advances in digitalization not only improve system efficiency but also provide operators with valuable insights that can help in preventive maintenance, reduce downtime, and extend the lifespan of heat recovery equipment.
The integration of energy storage solutions with exhaust gas heat recovery power generation systems is another avenue for increasing efficiency. As industries recover waste heat, there is often a mismatch between when energy is generated and when it is needed. By incorporating thermal energy storage (TES) or battery storage systems, excess recovered heat can be stored for later use, allowing for a more flexible energy management strategy. This is particularly advantageous in industries with fluctuating energy demands or in cases where recovered heat cannot immediately be converted to electricity. By storing heat or electricity, companies can ensure that energy is always available when needed, reducing reliance on external power sources and improving energy independence.
The development of thermoelectric materials is also expected to play a major role in the future of exhaust gas heat recovery systems. Thermoelectric generators (TEGs) convert waste heat directly into electricity by using semiconductor materials that generate voltage when subjected to a temperature difference. These materials, particularly nanostructured and quantum dot-based semiconductors, are being actively researched for their ability to operate efficiently at higher temperatures, enabling their use in more diverse industrial applications. The ability to directly convert exhaust heat into electricity through TEGs presents a promising solution for smaller-scale energy recovery systems, especially in mobile or remote applications such as vehicles or offshore platforms.
Furthermore, exhaust gas heat recovery systems can complement the use of renewable energy sources in certain industries. For example, in combined-cycle power plants that integrate both gas and steam turbines, exhaust gas heat recovery can play a pivotal role in supplementing the power generated from renewable sources like solar or wind energy. This integration of renewable and waste-heat-derived power enhances the overall system’s reliability and stability, helping to mitigate the intermittency issues associated with renewable energy production. As more industries turn to hybrid systems that combine traditional and renewable energy sources, the role of waste heat recovery in maintaining efficient energy use will continue to grow.
In district heating networks, which are used to provide heat to entire neighborhoods or industrial complexes, exhaust gas heat recovery systems can be integrated to maximize energy efficiency. These networks typically rely on centralized heating plants, often fueled by natural gas or waste materials. By using heat recovery systems to capture and reuse exhaust heat, these plants can provide additional heating or even generate electricity, reducing the need for extra fuel consumption and cutting down on emissions. The scalability of these systems makes them an ideal solution for urban areas and communities looking to adopt more sustainable energy practices.
Government incentives and regulatory frameworks continue to encourage the adoption of waste heat recovery technologies. In many countries, tax breaks, grants, or subsidies are offered to industries that implement energy-efficient systems, including exhaust gas heat recovery systems. These incentives make it financially attractive for companies to invest in such technologies, which can lead to a significant return on investment through energy savings over time. Furthermore, as governments tighten their carbon emissions standards, companies that integrate energy recovery solutions will be better positioned to meet these regulations and avoid costly penalties. These government policies are helping to accelerate the transition to energy-efficient systems, making waste heat recovery a key player in global sustainability efforts.
The potential applications of exhaust gas heat recovery extend well beyond traditional industrial sectors. The transportation sector, including automobiles, aviation, and railways, also stands to benefit significantly from these systems. As electric vehicles (EVs) continue to gain popularity, technologies that can help optimize energy use within these vehicles will be crucial. The integration of exhaust gas heat recovery systems in internal combustion engine vehicles could help reduce fuel consumption by converting excess heat into useful energy. In aviation, recovering heat from aircraft engines could allow for more efficient systems onboard, reducing fuel consumption and improving the overall environmental impact of air travel. Similarly, high-speed trains and freight locomotives that rely on combustion engines could also see significant benefits from adopting heat recovery solutions.
In chemical processing, petrochemical plants, and refineries, where high-temperature reactions are often required, exhaust gas heat recovery systems can help companies meet increasing energy demands while reducing operational costs. These industries are major consumers of energy, and optimizing energy use through heat recovery can result in significant savings. Additionally, the use of heat recovery systems helps refineries meet sustainability goals by reducing emissions and minimizing the environmental impact of their operations. For example, heat recovery systems can capture excess heat from cracking furnaces or distillation columns and either reuse it for other processes or convert it to electricity, enhancing energy efficiency and lowering greenhouse gas emissions.
As industries worldwide continue to innovate and prioritize energy efficiency, exhaust gas heat recovery power generation systems will play a crucial role in shaping the future of sustainable energy solutions. The technology is evolving rapidly, and ongoing research into more efficient heat exchangers, materials, and system configurations will further improve the potential for energy recovery. From small-scale applications in vehicles and remote power generation systems to large-scale operations in industrial manufacturing and power plants, exhaust gas heat recovery systems offer a promising avenue for reducing energy consumption, minimizing emissions, and achieving global sustainability targets.
In conclusion, exhaust gas heat recovery power generation systems are an essential component of the global shift towards more energy-efficient, sustainable industries. By capturing and reusing waste heat that would otherwise be released into the atmosphere, these systems significantly improve energy efficiency, reduce fuel consumption, and lower emissions. The wide-ranging applications across sectors, from power generation and industrial manufacturing to automotive and marine industries, demonstrate the versatility and importance of exhaust gas heat recovery in the modern energy landscape. With continued technological advancements, government support, and growing industry demand for sustainability, exhaust gas heat recovery systems are poised to play an increasingly central role in the future of energy generation.
Gas Power Generation from NG Power Plant Exhaust Gas
Gas power generation from natural gas (NG) power plant exhaust gas is a process designed to capture the thermal energy present in the exhaust gases produced by the combustion of natural gas in gas turbines and other equipment. This energy is then used to generate additional power, thus enhancing the overall efficiency of the power plant and reducing environmental impact. The process is primarily based on the concept of combined-cycle power generation, which integrates both gas turbines and steam turbines in a single power generation unit, optimizing the use of exhaust gases to produce electricity.
In a typical natural gas power plant, the fuel is burned in a gas turbine, which produces both electricity and hot exhaust gases. These exhaust gases, while integral to the power generation process, still contain a significant amount of unused thermal energy. Without a heat recovery system, this heat would be vented into the atmosphere, wasting valuable energy. However, with the installation of an exhaust gas heat recovery system, this wasted thermal energy can be captured and used to produce additional power.
The most common method for utilizing the thermal energy from exhaust gases in NG power plants is through a Heat Recovery Steam Generator (HRSG). The HRSG is a crucial component in combined-cycle plants, where it recovers the heat from the exhaust gases and uses it to produce steam. This steam is then directed to a steam turbine, which generates additional electricity. The integration of both gas and steam turbines in a combined-cycle plant allows for more efficient energy use by maximizing the power generated from the same amount of fuel. In essence, the gas turbine produces electricity, and the HRSG recovers waste heat to generate steam, which drives the steam turbine to produce even more electricity.
The combination of a gas turbine and a steam turbine in a single system can achieve efficiency levels of 60% or more, significantly higher than conventional single-cycle plants. This efficiency boost is a key advantage of combined-cycle gas power generation and plays an essential role in reducing operational costs and minimizing the environmental footprint of power plants. The exhaust gas heat recovery process, therefore, not only improves the efficiency of power generation but also helps reduce fuel consumption, lower CO2 emissions, and contribute to meeting global sustainability targets.
In addition to the use of HRSGs, other technologies can also be employed to convert exhaust gas heat into electricity. One such technology is the Organic Rankine Cycle (ORC). The ORC operates similarly to a traditional Rankine cycle, but instead of water, it uses organic fluids that have lower boiling points. This makes the ORC particularly suitable for recovering waste heat from lower-temperature exhaust gases, such as those produced by gas turbines in NG power plants. In the ORC, the heat from the exhaust gases is used to vaporize the organic fluid, which then drives a turbine connected to an electrical generator. ORC systems are commonly used when exhaust gas temperatures are insufficient to produce steam in a conventional HRSG.
Gas power generation from NG power plant exhaust gas can also be enhanced by incorporating combined heat and power (CHP) systems, also known as cogeneration. In a CHP system, the exhaust gas is not only used to generate electricity but also to provide thermal energy for other processes, such as district heating or industrial applications. This makes CHP systems highly efficient, as they provide both power and useful heat from a single fuel source. By recovering exhaust gas heat and using it for multiple purposes, these systems contribute to reducing the overall energy consumption and improving the energy recovery of the plant.
In combined-cycle plants, the gas turbine operates in a simple cycle, where it burns natural gas to generate electricity. The exhaust gases from this turbine still have significant thermal energy, which is typically around 600-700°C. This exhaust heat is passed through the HRSG, where it is used to generate steam. The steam is then directed to a steam turbine, where it drives a generator to produce additional power. The combined-cycle process increases the efficiency of the power plant by utilizing the exhaust heat that would otherwise be wasted. Combined-cycle power plants using natural gas are some of the most efficient fossil fuel power plants due to this ability to extract additional energy from the exhaust gases.
The integration of exhaust gas heat recovery in NG power plants can also contribute to reducing environmental impacts by lowering emissions. The increased efficiency of these plants means that less natural gas is required to produce the same amount of electricity, leading to reduced carbon dioxide (CO2) emissions. Furthermore, the use of HRSGs and ORC systems can help capture and utilize excess heat, reducing the need for additional fuel consumption. This, in turn, lowers the overall carbon footprint of the power generation process, which is critical in addressing climate change and complying with stringent emissions regulations set by governments around the world.
In addition to traditional natural gas power plants, exhaust gas heat recovery systems can also be implemented in natural gas-based combined-cycle plants that integrate renewable energy sources. For instance, solar thermal or geothermal energy can be used in conjunction with gas turbines to create hybrid systems that use both renewable and fossil fuel-derived energy. By integrating these systems, NG power plants can become even more efficient, reducing their reliance on natural gas while still producing reliable and dispatchable power.
Exhaust gas heat recovery systems can also be used to improve the performance of gas turbines in industrial applications. Many industries, such as chemical processing, steel manufacturing, and petrochemical refining, rely on natural gas as a primary energy source. The high-temperature exhaust gases from gas turbines used in these industries can be captured and directed through heat recovery systems, allowing for the generation of additional electricity or steam to meet the energy needs of the facility. This helps reduce energy costs, lower emissions, and enhance overall operational efficiency.
The use of advanced materials and innovative technologies continues to improve the effectiveness of exhaust gas heat recovery in NG power generation. For instance, the development of superalloys and ceramic coatings allows for better performance of heat exchangers and turbines, enabling them to operate at higher temperatures and more efficiently recover heat. Additionally, innovations in heat exchanger design have improved the heat transfer process, increasing the efficiency of energy recovery. The integration of smart technologies, such as sensors and automation, also allows for real-time monitoring and optimization of the heat recovery process, ensuring that the system is running at peak performance and minimizing downtime.
The economic and environmental benefits of gas power generation from NG power plant exhaust gas are evident. By recovering waste heat, power plants can reduce fuel consumption, lower operational costs, and meet increasingly strict environmental regulations. The ability to generate additional power from the exhaust gases not only boosts the efficiency of natural gas power plants but also contributes to reducing the overall carbon footprint of the energy sector. As the demand for more sustainable and efficient energy solutions grows, the role of exhaust gas heat recovery in NG power plants will continue to expand, driving advancements in technology and contributing to the global effort to reduce carbon emissions and combat climate change.
In conclusion, gas power generation from natural gas power plant exhaust gases is an essential technology for improving the efficiency of power plants, reducing fuel consumption, and lowering emissions. By recovering the thermal energy from exhaust gases and converting it into additional electricity, power plants can achieve significantly higher efficiency levels. The integration of heat recovery systems such as HRSGs and ORC systems allows for more sustainable energy production, reducing the reliance on natural gas while still meeting the energy needs of the grid. These technologies, along with the incorporation of CHP and hybrid renewable systems, are pivotal in the transition toward a more efficient and environmentally friendly energy system.
The evolution of gas power generation from natural gas (NG) power plant exhaust gases is being propelled by innovations in system efficiency and sustainability. As global energy demands continue to grow, so does the need for more effective and eco-friendly power generation solutions. One key area of development in this space is the integration of energy storage technologies into gas power generation systems. By pairing gas turbines and heat recovery systems with thermal energy storage (TES) or battery storage, excess energy generated from exhaust gases can be stored and later used when demand peaks. This is particularly beneficial for combined-cycle plants, which are capable of handling fluctuations in power demand more efficiently. The ability to store recovered energy allows power plants to optimize fuel use and reduce greenhouse gas emissions by decreasing reliance on supplementary fuel sources during peak periods.
In the context of distributed energy systems, exhaust gas heat recovery is also gaining traction in smaller-scale applications. For example, small to medium-sized combined heat and power (CHP) systems are becoming increasingly popular for commercial and industrial facilities that need both heat and electricity. These systems often rely on natural gas to generate power, and waste heat recovery from the exhaust gases can help increase the overall system efficiency, making them ideal for industrial zones, district heating, and large commercial facilities. The use of gas power generation combined with heat recovery in these scenarios provides businesses with a cost-effective and environmentally friendly alternative to traditional grid-based power.
Additionally, the growing demand for grid stability and dispatchable power has led to the adoption of gas power generation systems with exhaust gas heat recovery in areas that rely on intermittent renewable energy sources. Wind and solar power, while crucial in the push toward decarbonization, are subject to variability. Natural gas power plants with combined-cycle systems and waste heat recovery can provide backup power and load balancing, ensuring a stable energy supply when renewable resources are not available. By utilizing exhaust gas heat recovery to produce additional electricity, gas power plants can better integrate with renewable energy systems, helping to stabilize the grid and reduce the need for fossil-fuel-based backup plants.
Moreover, there is increasing interest in using digital twin technology and artificial intelligence (AI) in the optimization of gas power generation systems with exhaust gas heat recovery. A digital twin is a virtual replica of a physical system that can simulate its behavior and performance. In power plants, digital twins can be used to monitor and analyze exhaust gas heat recovery systems, identifying inefficiencies and predicting when maintenance is required. AI algorithms can further improve the decision-making process by analyzing large datasets to determine the most efficient operating conditions for the system, thus maximizing the energy recovered from exhaust gases.
In terms of environmental benefits, gas power generation with exhaust gas heat recovery is an essential tool in the transition to a low-carbon economy. It enables power plants to reduce their carbon emissions by generating more electricity from the same amount of fuel. This is critical in light of the increasing pressure on industries to meet climate change mitigation goals. In fact, combined-cycle gas turbine (CCGT) plants with exhaust gas heat recovery can achieve efficiencies of up to 60% or higher, which is a significant improvement compared to traditional single-cycle plants. The reduction in fuel consumption leads to a corresponding decrease in CO2 emissions, contributing to the broader objective of achieving net-zero emissions in the power sector.
Furthermore, as the push for green hydrogen continues to gain momentum, gas power generation with exhaust gas heat recovery may also play a role in supporting hydrogen production. Hydrogen can be produced through a process called steam methane reforming (SMR), which generates hydrogen from natural gas and produces large amounts of exhaust gas. By integrating exhaust gas heat recovery systems into hydrogen production facilities, plants can recover thermal energy from the exhaust and use it to support the hydrogen production process. This would not only make the process more energy-efficient but also help mitigate the carbon footprint of hydrogen production, especially in the early stages when hydrogen may still be derived from fossil fuels.
Another emerging application of exhaust gas heat recovery is in the hydropower sector. Although traditionally used in fossil fuel-based power plants, gas power generation technologies, including heat recovery systems, are now being explored in hydropower facilities, particularly those in remote regions where natural gas or biomass is more accessible than other forms of fuel. In these regions, exhaust gas heat recovery could provide a way to diversify the energy mix, improving efficiency and reducing reliance on intermittent renewable sources like hydro, which may be affected by seasonal variations in water availability.
Looking ahead, the future of gas power generation with exhaust gas heat recovery is likely to be shaped by continued advancements in materials science, efficiency technologies, and integrated power systems. Advanced heat exchangers, superconducting materials, and next-generation turbines will further enhance the performance of these systems, allowing for greater energy recovery from exhaust gases and even better efficiency in power generation. Moreover, the continued development of hybrid power systems, which combine natural gas with other renewable energy sources like wind, solar, and even nuclear, will further increase the role of exhaust gas heat recovery in ensuring a reliable and low-carbon energy supply.
In conclusion, gas power generation from NG power plant exhaust gases, particularly through the use of combined-cycle systems, heat recovery steam generators, and emerging technologies, is at the forefront of improving energy efficiency and sustainability in the power sector. By recovering waste heat from exhaust gases and converting it into additional power, these systems significantly boost the efficiency of natural gas power plants and contribute to reducing carbon emissions. The integration of digital technologies, energy storage, and hybrid systems will further optimize the performance of these systems, supporting global efforts to transition to more sustainable and reliable energy sources. As technology continues to advance, the role of gas power generation with exhaust gas heat recovery will remain critical in shaping the future of energy production.
Heat Recovery Heat Exchanger
A Heat Recovery Heat Exchanger (HRHE) is a critical component in various industries that helps recover thermal energy from a fluid stream, typically exhaust gases, and uses it to heat another fluid stream. This process allows for increased energy efficiency by reducing the need for external heating sources, leading to cost savings, enhanced operational efficiency, and a reduction in environmental impact. HRHEs are designed to capture waste heat, which would otherwise be lost, and reuse it in a productive manner, making them an essential element in energy recovery and sustainability efforts across different sectors.
The basic principle behind a heat recovery heat exchanger involves the transfer of heat from one fluid to another without the two fluids physically mixing. Heat is transferred through the walls of the heat exchanger, and depending on the design, it may either be a counterflow (where the two fluid streams flow in opposite directions), parallel flow, or shell and tube configuration. The type of heat exchanger used depends on factors such as the temperature difference, the nature of the fluids involved, and the required heat transfer rate.
In industrial applications, HRHEs are widely used to recover energy from exhaust gases produced by processes such as combustion, chemical reactions, and manufacturing operations. These gases often contain significant amounts of thermal energy that can be harnessed and used to preheat incoming air, water, or process fluids. By capturing and reusing this waste heat, companies can reduce their energy consumption and improve overall system efficiency.
In many industrial heating systems, HRHEs are integrated with other components like boilers, turbines, and chillers to maximize energy recovery. For example, in a combined-cycle power plant, the HRHE captures the waste heat from the gas turbine exhaust gases and uses it to generate steam, which in turn drives a steam turbine to produce additional electricity. This combination of technologies, known as combined-cycle generation, can achieve efficiencies greater than 60%, significantly improving the energy output of the plant.
In the HVAC (Heating, Ventilation, and Air Conditioning) industry, HRHEs are also used to improve the efficiency of air handling systems. They are typically installed in commercial and industrial buildings to recover heat from exhaust air and transfer it to incoming fresh air. This process, known as heat recovery ventilation (HRV) or energy recovery ventilation (ERV), is especially useful in buildings with strict energy efficiency standards. By preheating the incoming air with exhaust heat, HRHEs reduce the energy required to heat the air to comfortable temperatures, leading to lower operational costs and improved building performance.
The design of a heat recovery heat exchanger takes several factors into account to ensure optimal performance. One of the key considerations is the temperature gradient between the hot and cold fluid streams. The greater the temperature difference, the more efficient the heat exchange process will be. Other factors include the flow arrangement (counterflow or parallel flow), the type of heat transfer surface used, and the overall size of the heat exchanger. Additionally, the properties of the fluids involved, such as their specific heat capacity, viscosity, and corrosiveness, are also important when selecting materials and designing the heat exchanger to ensure its longevity and performance.
One of the challenges in the design of heat recovery heat exchangers is managing the fouling that can occur over time. Fouling refers to the accumulation of unwanted deposits on the heat transfer surfaces, which reduces the efficiency of heat transfer. These deposits can result from dirt, dust, or other particulate matter in the fluid streams, as well as from chemical reactions that take place during the process. To mitigate fouling, regular maintenance and cleaning are required, and in some cases, specialized coatings or materials that resist fouling may be used to extend the operational life of the heat exchanger.
The use of HRHEs is essential in improving energy efficiency and reducing environmental impact. In industrial plants, HRHEs can help companies meet stricter environmental regulations by reducing the overall energy consumption and emissions of harmful pollutants such as carbon dioxide (CO2), nitrogen oxides (NOx), and particulate matter. By recovering waste heat, plants can reduce their reliance on additional fuel sources, thereby decreasing the carbon footprint of their operations. The incorporation of HRHEs in industrial and power generation facilities helps companies meet sustainability goals while also reducing operational costs associated with fuel consumption and energy procurement.
HRHEs are also gaining traction in sustainable building designs and renewable energy projects. As the world moves toward a more sustainable energy future, the adoption of HRHEs in buildings, industrial facilities, and power plants is increasing. These systems are key in optimizing energy usage, promoting energy independence, and reducing the reliance on non-renewable energy sources. For example, in solar thermal systems, HRHEs can be used to store excess thermal energy for use during cloudy periods or at night, helping to maintain a consistent energy supply and improve the system’s efficiency. Similarly, HRHEs are being explored in geothermal energy systems, where they can recover heat from geothermal fluids and use it for electricity generation or district heating.
Another area of growing interest is the integration of HRHEs with waste-to-energy (WTE) plants. WTE facilities, which convert waste materials into energy, often generate large amounts of exhaust heat during the combustion process. By installing HRHEs, WTE plants can recover this heat and use it to preheat incoming waste, improve combustion efficiency, or generate additional power through steam turbines. This approach not only improves the plant’s energy efficiency but also contributes to reducing the environmental impact of waste disposal by recovering energy that would otherwise be lost.
The integration of HRHEs with carbon capture technologies is another area being actively explored. Carbon capture and storage (CCS) systems are designed to capture carbon dioxide emissions from industrial processes and store them underground to prevent them from entering the atmosphere. However, these systems require significant energy to operate. By utilizing heat recovery from exhaust gases, HRHEs can provide the necessary thermal energy for the CO2 capture process, reducing the overall energy consumption and improving the economics of CCS projects.
Technological advancements in HRHEs are also driving innovation in heat exchanger materials and designs. Modern HRHEs incorporate advanced materials, such as composite metals, ceramics, and high-performance alloys, which allow for higher heat transfer rates and resistance to corrosion and high temperatures. These materials enable HRHEs to perform more efficiently in extreme conditions, such as those found in gas turbines and chemical reactors, where temperatures can reach several hundred degrees Celsius.
In conclusion, heat recovery heat exchangers are an essential technology for improving energy efficiency, reducing operational costs, and mitigating environmental impact across a wide range of industries. From power generation and industrial manufacturing to HVAC systems and sustainable building designs, HRHEs play a vital role in recovering waste heat and converting it into useful energy. With ongoing advancements in materials, design, and system integration, HRHEs are poised to become even more efficient and versatile, contributing to a more sustainable energy future. As industries and governments continue to focus on energy conservation, carbon reduction, and sustainable development, the importance of heat recovery systems will only continue to grow.
The continued development of heat recovery heat exchangers (HRHEs) is particularly important in the context of the global energy transition. As nations and industries alike focus on reducing carbon emissions and improving energy efficiency, the role of HRHEs in cutting down fuel consumption and decreasing greenhouse gas emissions becomes even more crucial. Beyond improving the energy efficiency of individual processes, HRHEs help meet broader environmental targets by enabling industries to maximize the use of available energy. In many cases, waste heat recovery has been identified as one of the most effective and economically viable strategies for reducing overall energy use.
As part of the broader shift toward decarbonization, HRHEs are also being integrated into more advanced energy systems like hydrogen production, carbon capture, and bioenergy systems. In green hydrogen production, for example, HRHEs can recover excess heat from the steam reforming process (used to produce hydrogen from natural gas) and other stages of hydrogen generation. This thermal energy can then be used to drive the chemical reactions more efficiently, minimizing the need for external energy inputs and improving the overall sustainability of the hydrogen production process.
Furthermore, smart technology integration is another area where HRHEs are evolving. The increasing use of sensor networks and Internet of Things (IoT) devices allows for real-time monitoring and optimization of heat recovery systems. By analyzing operational data, operators can adjust the flow rates, temperatures, and pressures to maximize energy recovery and system efficiency. Artificial intelligence (AI) and machine learning (ML) algorithms can also play a significant role in predictive maintenance, reducing downtime by forecasting potential issues with the heat exchanger before they result in a failure. This proactive approach ensures that the HRHE operates at peak efficiency for longer periods and extends its service life.
Additionally, the integration of HRHEs with energy storage systems can further enhance their value. By pairing heat recovery systems with thermal energy storage (TES) technologies, waste heat can be stored and used during periods of low demand or high energy prices, effectively decoupling energy consumption from demand fluctuations. This integration has the potential to improve the flexibility of industrial processes and power plants, allowing for more consistent energy output and reduced reliance on grid-based electricity. This is particularly useful for industries with intermittent energy demands or those located in areas with limited access to a stable grid.
In district energy systems, HRHEs are being used to improve the efficiency of heating and cooling networks in urban environments. By capturing waste heat from nearby industrial plants, data centers, or other heat-generating sources, HRHEs can significantly reduce the need for additional heating fuels, such as natural gas or oil. In cities aiming to achieve net-zero carbon emissions, such systems are vital in providing both heating and cooling in an efficient, environmentally friendly manner.
HRHEs are also playing a pivotal role in enhancing the energy efficiency of waste-to-energy (WTE) facilities. In WTE plants, waste materials such as municipal solid waste (MSW), industrial waste, and biomass are burned to generate power. The exhaust gases produced in this process contain a considerable amount of heat that can be recovered and used to improve the efficiency of the overall plant. This recovered energy can be used to preheat air, steam, or water, improving the overall combustion process and the energy output of the plant. Moreover, heat recovery systems in WTE facilities are critical for reducing the need for supplementary fuel inputs and making the waste-to-energy process more economically viable.
The role of HRHEs in industrial decarbonization is also gaining increasing importance. Heavy industries like steel manufacturing, cement production, and chemical processing are major contributors to global CO2 emissions due to their high energy demands. By incorporating HRHEs into these operations, companies can reduce the need for additional fuel inputs and optimize their energy use, helping them meet stricter environmental regulations and carbon reduction targets. In steel manufacturing, for instance, HRHEs can recover heat from the hot exhaust gases produced during the steelmaking process and use it to preheat blast air or to generate electricity, contributing to lower fuel consumption and fewer emissions.
As renewable energy sources such as solar and wind continue to proliferate, integrating HRHEs into energy systems that rely on these intermittent resources can help stabilize grid operations. These renewable sources are often subject to fluctuations in availability, which can create challenges in balancing supply and demand. HRHEs can play a significant role in hybrid systems, where waste heat from gas turbines, biomass, or even geothermal energy systems is used in conjunction with renewable energy sources to provide a steady and reliable power output. By storing and recovering heat energy from waste gases, the system can provide dispatchable power, acting as a backup for renewables during times of low output.
In high-temperature applications like gas turbines and reactors, HRHEs are vital for recovering heat from exhaust gases that would otherwise be wasted. The use of advanced materials like ceramics and superalloys is making it possible for HRHEs to withstand extreme conditions, including high temperatures and corrosive exhaust gases. This is crucial for applications in sectors such as aviation, space exploration, and nuclear power, where waste heat recovery is essential for improving fuel efficiency and reducing energy costs.
Finally, the future of heat recovery heat exchangers lies in the development of next-generation designs and materials that can handle more extreme environments, improve heat transfer efficiency, and reduce fouling. Researchers are exploring the use of nanotechnology and microscale heat exchangers to increase the surface area for heat transfer, improving the overall efficiency of heat recovery systems. These advanced materials could revolutionize the way HRHEs are used in various industries, making them even more efficient and adaptable to different applications.
In conclusion, heat recovery heat exchangers are crucial for improving energy efficiency and reducing environmental impact across a wide array of industries. Their role in recovering waste heat and converting it into usable energy is indispensable in efforts to reduce fuel consumption, lower carbon emissions, and optimize industrial processes. As technology continues to advance, the efficiency of HRHEs will improve, furthering their contribution to sustainable energy solutions and decarbonization efforts worldwide. With the increasing focus on energy conservation, cost reduction, and environmental sustainability, HRHEs will remain a key component in the future of energy recovery systems.
Waste Heat Recovery Boiler
A Waste Heat Recovery Boiler (WHRB) is a vital component in energy recovery systems that harnesses and recycles heat from exhaust gases or other waste heat sources to generate steam or hot water. These systems are designed to capture heat that would otherwise be wasted, particularly from industrial processes, and convert it into useful thermal energy. Waste heat recovery boilers are widely used across a range of industries, including power generation, petrochemical, steel manufacturing, and chemical processing, due to their ability to improve energy efficiency and reduce operational costs.
The fundamental operation of a WHRB involves the transfer of heat from hot exhaust gases or liquids to water or another fluid in a heat exchanger system. This recovered heat can then be used to generate steam, which may be utilized for driving turbines, preheating processes, or even providing heat for industrial or heating purposes. By recovering and reusing waste heat, these systems reduce the need for additional fuel consumption, leading to significant savings in energy costs and a reduction in carbon emissions.
The design of a waste heat recovery boiler is highly dependent on the characteristics of the waste heat source, including the temperature, flow rate, and chemical composition of the exhaust gases. It typically consists of a heat exchanger that captures the thermal energy from the exhaust gases and transfers it to water or another fluid. In some designs, multiple heat exchangers are used to recover heat at different stages of the exhaust flow, improving the overall efficiency of the system.
One common application of waste heat recovery boilers is in combined-cycle power plants. In these plants, the exhaust gases from a gas turbine are directed through a WHRB, where the heat is recovered and used to produce steam. This steam then drives a steam turbine, generating additional electricity. This process, known as combined-cycle generation, allows power plants to achieve efficiency levels of 60% or higher, which is significantly higher than conventional single-cycle power generation methods.
In industrial manufacturing settings, WHRBs are used to recover heat from high-temperature exhaust gases, such as those produced by furnaces, kilns, or combustion engines. This heat is often used to preheat air, water, or process fluids, reducing the energy required for these tasks. For example, in a cement plant, WHRBs can capture the waste heat generated by rotary kilns used for cement production and use it to generate steam for power generation or process heating. Similarly, in refining or petrochemical plants, WHRBs can recover heat from crude oil distillation or gas cracking processes and use it to power boilers or provide heat to other stages of production.
A significant advantage of WHRBs is their ability to improve energy efficiency and reduce operational costs. By capturing and reusing waste heat, these systems reduce the need for external energy inputs, such as natural gas or electricity, thus lowering overall energy consumption. Furthermore, by reducing the demand for fuel, WHRBs help lower operating costs and contribute to achieving sustainability goals, particularly in industries that have high energy requirements.
The benefits of using a waste heat recovery boiler extend beyond cost savings and energy efficiency. By reducing fuel consumption, these systems also help reduce greenhouse gas emissions and other environmental pollutants. This is particularly relevant in industries like power generation and manufacturing, which are significant contributors to global CO2 emissions. By implementing WHRBs, companies can make substantial progress in their efforts to decarbonize their operations and meet increasingly stringent environmental regulations.
In some industries, the recovered heat from a WHRB can be used not only to generate steam but also to support district heating systems. In district energy systems, hot water or steam generated by the WHRB is distributed through a network of pipes to multiple buildings or facilities in a localized area. This is a highly efficient way to provide heating to commercial, industrial, and residential buildings while simultaneously reducing the demand for fossil fuel-based heating sources. The ability to harness waste heat in this way is becoming increasingly important as cities and municipalities aim to reduce their carbon footprints and meet climate targets.
Another key application of WHRBs is in cogeneration (CHP) systems, which simultaneously generate electricity and useful thermal energy from a single energy source. In these systems, the waste heat recovered by the WHRB can be used for space heating, industrial processes, or other applications, improving the overall efficiency of the system. Cogeneration is particularly valuable in industries that have both electrical and thermal energy demands, such as food processing, chemical manufacturing, and paper mills.
The efficiency of a WHRB depends largely on the temperature of the exhaust gases and the heat recovery potential. High-temperature waste heat, such as that from a gas turbine or furnace, typically results in a more efficient heat recovery process. To maximize the heat recovery potential, modern WHRBs are often equipped with advanced features such as economizers, superheaters, and reheaters. These components are designed to further increase the temperature of the recovered heat, improving the steam generation process and enhancing the overall efficiency of the system.
Despite their many advantages, waste heat recovery boilers also face some challenges, particularly in terms of design complexity and maintenance. The efficiency of a WHRB can be affected by factors such as fouling, where deposits build up on the heat exchange surfaces, reducing the overall heat transfer efficiency. Regular cleaning and maintenance are required to prevent this buildup and ensure the system operates efficiently over time. Additionally, the chemical composition of the exhaust gases must be carefully considered when designing the system, as certain compounds may corrode the materials used in the boiler or heat exchangers. To mitigate these issues, manufacturers often use corrosion-resistant materials and coatings that extend the life of the equipment and reduce maintenance costs.
The ongoing development of materials and design improvements for WHRBs is helping to address some of these challenges. For example, the use of ceramic coatings or composite materials in high-temperature applications is increasing, as these materials are more resistant to corrosion and can withstand higher temperatures. Similarly, modular designs for WHRBs allow for easier installation and maintenance, enabling better integration with existing infrastructure and facilitating more flexible applications.
The integration of advanced control systems and sensor technology is also improving the efficiency and performance of waste heat recovery boilers. Real-time monitoring of key performance parameters, such as exhaust gas temperature, flow rate, and pressure, allows operators to optimize the heat recovery process and ensure that the system is operating at peak efficiency. Automation and predictive maintenance technologies also help reduce downtime and increase the lifespan of the WHRB, minimizing operational disruptions and reducing overall maintenance costs.
In conclusion, waste heat recovery boilers are an essential technology for improving energy efficiency, reducing operating costs, and minimizing environmental impact. By capturing waste heat and converting it into useful thermal energy, these systems play a critical role in a variety of industries, including power generation, manufacturing, and refining. With their ability to recover and reuse thermal energy, WHRBs help reduce the demand for external fuel sources, lower emissions, and enhance overall system efficiency. As industries and governments continue to focus on sustainability and energy conservation, waste heat recovery boilers will remain a key component in the pursuit of a more energy-efficient and environmentally responsible future.
As the world transitions toward more sustainable and energy-efficient technologies, the role of Waste Heat Recovery Boilers (WHRBs) will continue to evolve and expand across various industries. The increasing demand for energy efficiency, resource optimization, and carbon footprint reduction has made WHRBs an essential part of industrial decarbonization strategies.
One of the significant trends in the field of waste heat recovery is the development of integrated systems that combine WHRBs with other energy recovery technologies. For example, combined heat and power (CHP) systems that incorporate WHRBs allow industries to produce both electricity and useful thermal energy from a single energy source, making them highly efficient. In many industries, these integrated systems are being optimized to serve multiple purposes simultaneously, including power generation, space heating, and process heating. This holistic approach helps industries reduce their overall reliance on fossil fuels while improving both operational efficiency and sustainability.
The push for circular economies is also influencing the demand for WHRBs. Circular economy models emphasize the reuse and recycling of materials and energy, and WHRBs align perfectly with this approach. By recovering and reusing waste heat, industries can maximize the utility of their energy inputs, minimizing waste and promoting more sustainable practices. This principle is particularly relevant in energy-intensive industries such as cement production, metal smelting, and paper manufacturing, where energy costs account for a significant portion of operational expenses.
The development of advanced heat recovery technologies also plays a crucial role in improving the performance of waste heat recovery boilers. For instance, the integration of high-efficiency heat exchangers, such as plate heat exchangers or fin-tube heat exchangers, enables higher heat transfer rates and more effective energy recovery. These innovations increase the overall efficiency of WHRBs, making them even more attractive for industries looking to maximize energy savings.
Another area of development is the use of smart monitoring systems and artificial intelligence (AI) in the operation of WHRBs. These technologies allow for real-time data analysis and optimization, ensuring that waste heat recovery systems operate at peak efficiency. For instance, AI algorithms can be used to predict maintenance needs and detect potential issues before they lead to failures, reducing downtime and extending the lifespan of the system. Additionally, AI can assist in adjusting operational parameters, such as flow rates and temperatures, to maximize heat recovery efficiency based on the specific needs of the plant at any given time.
In the context of low-carbon technologies, WHRBs are becoming increasingly important in the production of green hydrogen. As hydrogen production moves towards greener methods, such as electrolysis powered by renewable energy or biomass-based hydrogen production, WHRBs can help recover heat from the processes involved. This recovered heat can be used to power various stages of hydrogen production, reducing the overall energy consumption and making the process more efficient and environmentally friendly. Additionally, the integration of WHRBs into carbon capture systems helps reduce the energy requirements of CO2 capture processes, contributing to the overall decarbonization of industrial activities.
The global push toward sustainability has also led to an increase in government incentives and regulations that promote the adoption of energy-efficient technologies like WHRBs. Many countries and regions are setting ambitious carbon neutrality and net-zero emissions targets, which require industries to significantly reduce their carbon emissions. Waste heat recovery systems offer a cost-effective solution for industries to meet these targets while simultaneously reducing operating costs. Furthermore, government support for energy-efficient technologies, such as grants, subsidies, and tax incentives, is making the adoption of WHRBs more financially feasible for companies, even those with significant capital investment constraints.
Decentralized energy systems are another area where WHRBs are seeing increased application. As industries, businesses, and even entire cities transition to more localized energy production and distribution models, waste heat recovery boilers can play a key role in these microgrids. By recovering heat from industrial exhaust gases, WHRBs can provide reliable and affordable energy for localized areas, reducing the dependency on centralized grid systems. This decentralization can help improve energy security, reduce transmission losses, and enhance the resilience of energy infrastructure.
In the context of waste-to-energy (WTE) plants, WHRBs are particularly important in maximizing the energy recovery potential from non-recyclable waste materials. Waste-to-energy facilities, which convert municipal solid waste (MSW) into electricity and heat, produce a significant amount of exhaust heat during the combustion process. By integrating WHRBs, these plants can significantly improve their efficiency, reducing the amount of waste that needs to be landfilled while simultaneously providing cleaner energy. The use of waste heat in these plants supports a more sustainable waste management strategy, helping to close the loop on waste disposal and energy production.
The continuous innovation in material science also plays a significant role in advancing the capabilities of waste heat recovery boilers. The development of heat-resistant materials, such as superalloys, ceramics, and composites, allows WHRBs to handle increasingly higher temperatures and more aggressive exhaust gases without degradation. These materials not only improve the longevity and reliability of the boilers but also enable them to capture heat from more extreme sources, such as exhaust gases from high-efficiency turbines or industrial furnaces. This flexibility broadens the scope of potential applications for WHRBs, further enhancing their value as a sustainable energy recovery solution.
Moreover, the continued global adoption of clean energy solutions, such as solar, wind, and geothermal energy, presents opportunities for WHRBs to complement these renewable sources. For example, in solar thermal power plants, WHRBs can be used to recover heat from concentrated solar power systems and improve the overall efficiency of the plant. In geothermal energy systems, waste heat from geothermal wells can be captured and utilized to generate additional electricity or heat. This integration of WHRBs with renewable energy technologies helps optimize energy production while reducing reliance on non-renewable resources.
In conclusion, waste heat recovery boilers (WHRBs) represent a crucial technology in the push for a more energy-efficient and sustainable future. Their ability to recover and reuse waste heat provides industries with a cost-effective and environmentally friendly solution to improve energy efficiency, reduce operational costs, and minimize carbon emissions. With ongoing advancements in design, materials, and integration with other energy systems, WHRBs are set to play an even greater role in global decarbonization efforts, helping industries and power plants transition toward cleaner, more sustainable energy practices. As industries continue to focus on energy conservation, WHRBs will remain a key player in the future of energy recovery and environmental sustainability.
EMS Power Machines
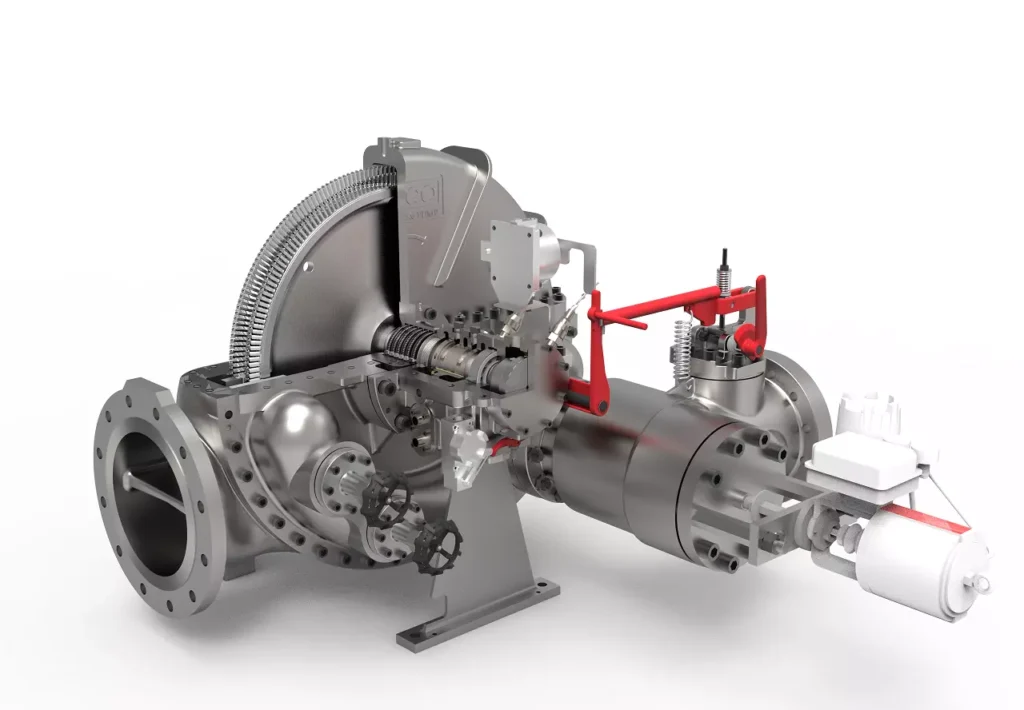
We design, manufacture and assembly Power Machines such as – diesel generators, electric motors, vibration motors, pumps, steam engines and steam turbines
EMS Power Machines is a global power engineering company, one of the five world leaders in the industry in terms of installed equipment. The companies included in the company have been operating in the energy market for more than 60 years.
EMS Power Machines manufactures steam turbines, gas turbines, hydroelectric turbines, generators, and other power equipment for thermal, nuclear, and hydroelectric power plants, as well as for various industries, transport, and marine energy.
EMS Power Machines is a major player in the global power industry, and its equipment is used in power plants all over the world. The company has a strong track record of innovation, and it is constantly developing new and improved technologies.
Here are some examples of Power Machines’ products and services:
- Steam turbines for thermal and nuclear power plants
- Gas turbines for combined cycle power plants and industrial applications
- Hydroelectric turbines for hydroelectric power plants
- Generators for all types of power plants
- Boilers for thermal power plants
- Condensers for thermal power plants
- Reheaters for thermal power plants
- Air preheaters for thermal power plants
- Feedwater pumps for thermal power plants
- Control systems for power plants
- Maintenance and repair services for power plants
EMS Power Machines is committed to providing its customers with high-quality products and services. The company has a strong reputation for reliability and innovation. Power Machines is a leading provider of power equipment and services, and it plays a vital role in the global power industry.
EMS Power Machines, which began in 1961 as a small factory of electric motors, has become a leading global supplier of electronic products for different segments. The search for excellence has resulted in the diversification of the business, adding to the electric motors products which provide from power generation to more efficient means of use.