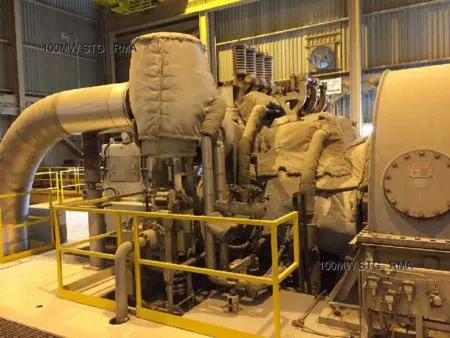
How do Steam Turbines Work: Steam turbines are devices that convert thermal energy from steam into mechanical energy, which can then be used to generate electricity or power mechanical equipment. They are essential components in power plants, ships, and industrial applications. Here’s a detailed explanation of how steam turbines function:
Basic Working Principle of Steam Turbines
At the core, a steam turbine operates by harnessing the energy stored in high-pressure, high-temperature steam. The basic process involves the following steps:
- Steam Generation:
- Steam is produced by heating water in a boiler. This process requires a heat source, which could be burning coal, natural gas, nuclear reactions, or renewable sources like biomass.
- The water boils and turns into steam, which is then superheated (heated beyond its boiling point) to increase its energy content.
- Expansion of Steam:
- The high-energy steam is directed into the turbine through nozzles. These nozzles convert the steam’s thermal energy into kinetic energy, creating a high-speed jet of steam.
- The jet of steam strikes the blades of the turbine, causing them to spin. This mechanical energy is harnessed by the turbine’s rotor, which is connected to a generator or other equipment.
- Conversion to Mechanical Energy:
- As steam passes through the turbine, it expands and loses pressure. This expansion process drives the turbine blades, causing the rotor to spin.
- The mechanical energy from the rotating rotor can be used to drive electrical generators, which convert it into electricity, or to power mechanical devices like pumps or compressors.
- Exhaust and Condensation:
- After passing through the turbine, the low-pressure steam is exhausted into a condenser, where it is cooled and condensed back into water.
- This water can be recycled back into the boiler to produce more steam, completing the cycle.
Types of Steam Turbines
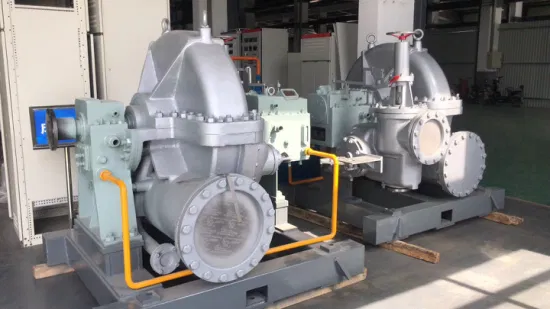
There are two main types of steam turbines based on how they convert steam energy into mechanical energy: impulse turbines and reaction turbines.
Impulse Turbines
- In an impulse turbine, steam is directed through nozzles to create high-speed jets. These jets strike the turbine blades, which change the direction of the steam flow, imparting energy to the rotor.
- The pressure of the steam drops primarily in the nozzles, and the blades just extract the kinetic energy from the high-speed steam.
- Example: The Curtis turbine is a type of impulse turbine that uses multiple stages to extract more energy from the steam.
Reaction Turbines
- Reaction turbines work on the principle of Newton’s third law: “for every action, there is an equal and opposite reaction.” Steam expands and accelerates as it passes through both the fixed and moving blades of the turbine.
- Unlike impulse turbines, the pressure of the steam drops as it passes through the blades, not just in the nozzles. The steam’s pressure energy is gradually converted into kinetic energy and then mechanical energy.
- Example: Reaction turbines are often used in large power plants due to their efficiency over varying loads.
Key Components of a Steam Turbine
Several critical components make up a steam turbine, each playing a specific role:
- Rotor:
- The central shaft that rotates as the steam moves through the turbine blades. The rotor is connected to a generator or other mechanical devices that need power.
- Blades:
- The blades are the most important part of the turbine, as they are responsible for extracting energy from the steam. Blades are designed to withstand high temperatures and pressures and must be highly durable.
- Different designs are used for impulse and reaction turbines to optimize the efficiency of energy extraction.
- Nozzles:
- These control the flow of steam entering the turbine, converting the pressure of the steam into high-speed jets that drive the blades. Nozzles are primarily used in impulse turbines.
- Casing:
- The casing encloses the turbine and directs the flow of steam, ensuring it moves efficiently through the blades. The casing also helps contain the steam pressure and protect the components.
- Condenser:
- After passing through the turbine, the steam is cooled and condensed back into water in the condenser. This step is crucial for maintaining the efficiency of the steam cycle, as it allows for the reuse of water in the boiler.
Efficiency and Energy Conversion
The efficiency of a steam turbine depends on various factors, including steam pressure, temperature, and turbine design. Modern turbines often incorporate multiple stages (cascades of blades) to maximize energy extraction. Each stage involves a small drop in steam pressure, allowing more gradual and efficient conversion of energy.
High-efficiency turbines use superheated steam, meaning the steam is heated well beyond its boiling point. This increases the energy content of the steam, which allows for more power to be generated from the same amount of fuel. Efficient condensation systems also help by reducing energy losses and enabling the reuse of water.
Applications of Steam Turbines
Steam turbines are versatile and have a wide range of applications, including:
- Power Generation:
- Steam turbines are the primary method of generating electricity in thermal power plants, including those powered by coal, natural gas, nuclear energy, and even renewable resources like biomass.
- Marine Propulsion:
- Historically, steam turbines powered many naval ships and civilian vessels. Although they have been largely replaced by diesel engines in modern ships, they are still used in certain specialized vessels.
- Industrial Applications:
- Steam turbines are used in industries to drive compressors, pumps, and other mechanical equipment. They are also employed in cogeneration systems, where the waste heat from industrial processes is used to produce steam for power generation.
Advantages of Steam Turbines
- High Efficiency:
- Especially when operating with superheated steam and multi-stage designs, steam turbines can achieve high efficiency, making them suitable for large-scale power generation.
- Reliable and Long-Lasting:
- Properly maintained steam turbines can operate for decades, offering reliable performance and durability.
- Versatile Energy Source:
- Steam turbines can run on various fuel sources, including fossil fuels, nuclear, and renewable energy sources like biomass, making them adaptable for different energy markets.
Conclusion
Steam turbines play a crucial role in the modern energy landscape, enabling the efficient conversion of thermal energy into mechanical and electrical power. By understanding how they work, their different types, and their key components, it becomes clear why they are a preferred choice for power generation across the world. Whether used in massive power plants, ships, or smaller industrial applications, steam turbines continue to be a reliable and efficient means of generating energy.
Steam Generation: How It Works and Its Importance in Power Plants
Steam generation is a fundamental process in power plants and various industrial applications. It involves converting water into steam, which can be used to produce mechanical power in turbines or provide thermal energy for heating and other processes. This section explores how steam is generated, the equipment involved, and why it is crucial for energy production.
1. What Is Steam Generation?
Steam generation is the process of heating water to its boiling point to produce steam. This steam can then be superheated, meaning it is heated beyond its boiling point to increase its energy content. The steam is then directed into a system where it is used to perform work, such as turning a turbine in a power plant or driving industrial machinery.
Applications of Steam Generation:
- Power Plants: To generate electricity.
- Industrial Processes: For heating, drying, sterilization, and powering equipment.
- Marine Vessels: To propel ships.
- Residential Heating: In some cases, steam is used for district heating systems.
2. The Steam Generation Process
The process of generating steam involves several key stages and components. Below is an overview of how steam is typically produced in a thermal power plant:
Step 1: Water Supply and Preheating
- The process begins with a continuous supply of water, often treated to remove impurities. This water is stored in a tank or reservoir.
- To improve efficiency, the water is preheated before entering the boiler. Preheating reduces the energy required to convert it into steam, often using waste heat from the system’s exhaust or other parts of the process.
Step 2: The Boiler and Heat Source
- The core of steam generation is the boiler. A boiler is a closed vessel where water is heated by a heat source.
- The heat source can vary:
- Coal or Natural Gas: Commonly burned in thermal power plants to heat water.
- Nuclear Reaction: In nuclear power plants, the heat is generated by nuclear fission.
- Biomass or Waste: Organic matter like wood, agricultural residue, or waste can be used as a renewable energy source.
- Solar Energy: Concentrated solar power (CSP) systems use mirrors to focus sunlight to heat water.
Step 3: Boiling and Steam Production
- Inside the boiler, the water is heated to its boiling point (100°C or 212°F at sea level) and begins to convert into steam. As the water absorbs more heat, it undergoes a phase change from liquid to gas, creating steam.
- In high-efficiency systems, the steam is superheated to higher temperatures, often between 300°C to 600°C (572°F to 1,112°F). Superheated steam carries more energy and can produce more power.
Step 4: Steam Delivery and Control
- The generated steam is then routed through pipes to the location where it will be used, such as a steam turbine in a power plant or an industrial processing unit.
- Steam flow is carefully controlled using valves to regulate pressure and temperature, ensuring that the system operates safely and efficiently.
Types of Boilers Used in Steam Generation
Boilers are the primary equipment for steam generation, and they come in various designs to suit different needs. Here are some of the most common types:
Fire-Tube Boilers
- In fire-tube boilers, hot gases produced from burning fuel pass through tubes that run through a container of water. The heat from the gases is transferred to the water, causing it to boil and produce steam.
- Advantages: Simple design, low initial cost, and easy to install and operate.
- Applications: Smaller industrial plants and heating systems.
Water-Tube Boilers
- In water-tube boilers, water flows through tubes that are surrounded by hot gases. This design allows for higher pressure and temperature steam production.
- Advantages: Can handle high-pressure and high-temperature steam, more efficient for large-scale power generation.
- Applications: Large power plants and industrial applications.
Once-Through Boilers
- These boilers do not store water; instead, water flows continuously through the system and is heated to produce steam. This design helps improve efficiency and reduces the risk of scale buildup.
- Advantages: High efficiency, quick startup, and reduced water treatment requirements.
- Applications: Modern thermal power plants, especially those using supercritical and ultra-supercritical steam cycles.
Efficiency Enhancements in Steam Generation
Improving the efficiency of steam generation can significantly reduce fuel consumption and emissions. Here are some methods to achieve higher efficiency:
Preheating the Water (Economizers)
- Economizers are heat exchangers that capture waste heat from exhaust gases and use it to preheat the water entering the boiler. This reduces the energy needed to bring the water to boiling point.
Superheating Steam
- Superheated steam carries more energy than saturated steam (steam at its boiling point). By heating the steam beyond its boiling point, power plants can extract more energy from the same amount of steam, increasing overall efficiency.
Condensate Recovery
- After steam has been used (e.g., in a turbine), it is often condensed back into water. This condensate can be collected, treated, and recycled, reducing the need for fresh water and saving energy that would otherwise be used to heat cold water.
Importance of Steam Generation in Power Plants
Steam generation is the backbone of most power plants. Here’s why it is crucial:
- Efficient Energy Conversion:
- Thermal energy can be efficiently converted into mechanical and then electrical energy through steam turbines. This makes steam generation a versatile solution for power generation.
- Adaptability to Various Fuels:
- Steam can be generated using a wide range of fuel sources, from fossil fuels like coal and natural gas to renewable energy sources such as biomass and concentrated solar power.
- This adaptability allows countries to leverage their available resources for energy production.
- Scalability:
- Steam generation systems can be scaled to suit different needs, from small-scale industrial applications to massive power plants capable of generating hundreds of megawatts.
- Combined Heat and Power (CHP):
- Steam generation can also be integrated into cogeneration or CHP systems, where the waste heat from electricity production is used for heating purposes. This dual-use significantly improves overall energy efficiency.
Challenges in Steam Generation
While steam generation is effective, it does come with challenges that need to be managed:
- Fuel Cost and Availability:
- The cost and availability of fuel sources (e.g., coal, natural gas, biomass) can affect the economic viability of steam generation systems.
- Water Usage:
- Steam generation requires a large amount of water, which can be a challenge in regions with water scarcity. Effective water management and condensate recovery systems can help mitigate this issue.
- Emissions and Environmental Impact:
- Burning fossil fuels to generate steam can lead to emissions of greenhouse gases (e.g., CO₂) and other pollutants. Transitioning to renewable fuel sources and improving efficiency are essential to reduce the environmental impact.
Conclusion
Steam generation is a vital process that powers the majority of the world’s energy systems. It involves heating water to produce steam, which is then used to drive turbines and generate electricity or perform mechanical work. By leveraging different boiler designs, preheating, and condensate recovery techniques, the efficiency of steam generation can be significantly enhanced. Although it faces challenges, ongoing innovations and a shift toward renewable energy sources are helping to make steam generation cleaner and more efficient, ensuring its role as a key component of the global energy infrastructure.
Expansion of Steam: How It Drives Turbines and Enhances Efficiency
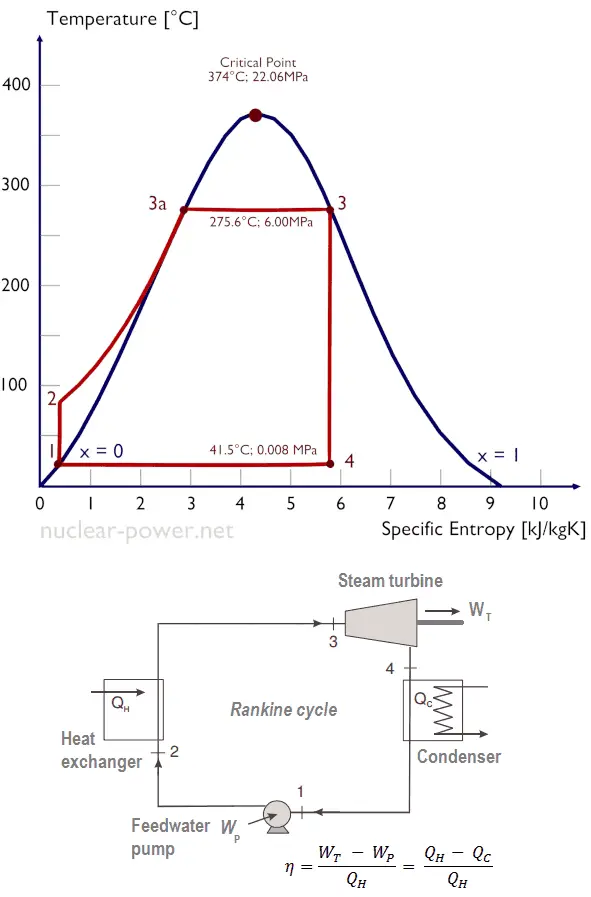
The expansion of steam is a critical phase in the process of converting thermal energy into mechanical energy. In steam turbines, the high-pressure, high-temperature steam expands as it moves through the turbine, causing the blades to spin and generate mechanical energy. Understanding the expansion process is essential for appreciating how steam turbines work, as well as for improving efficiency and optimizing power generation.
1. What Is Steam Expansion?
Steam expansion refers to the process where steam under high pressure and temperature passes through a turbine and undergoes a drop in pressure and temperature, resulting in an increase in volume. This change allows the steam to transfer its thermal and pressure energy into kinetic energy, which is then converted into mechanical energy as the turbine blades spin.
Key Characteristics of Steam Expansion:
- Pressure Drop: As the steam expands, it loses pressure.
- Temperature Drop: The expansion process also results in a decrease in temperature.
- Increase in Volume: The steam’s volume increases significantly, enabling it to exert force on the turbine blades and create motion.
2. The Role of Steam Expansion in Turbines
The expansion of steam is what drives the rotor in a steam turbine. Here’s how the process unfolds:
- High-Pressure Steam Enters the Turbine:
- Steam produced in the boiler is at a high pressure and high temperature. It enters the turbine through nozzles, where it is converted into a fast-moving jet of steam.
- The nozzles direct the steam onto the blades of the turbine, creating a controlled flow that maximizes the energy transfer.
- Conversion of Energy:
- As the steam moves through the turbine, it expands. This expansion results in a drop in pressure and temperature, converting the thermal energy of the steam into kinetic energy (speed and motion).
- The kinetic energy causes the turbine blades to rotate, which in turn spins the rotor connected to a generator, producing electricity.
- Multiple Expansion Stages:
- To increase efficiency, many steam turbines use multiple stages of expansion. These are known as multi-stage turbines.
- In each stage, steam passes through a set of blades (or a series of blades) where it expands a little more. This gradual expansion allows for more controlled energy extraction and minimizes energy loss.
- High-Pressure Stage: Initial expansion occurs here, extracting most of the energy.
- Intermediate-Pressure Stage: Steam continues to expand, losing more pressure and temperature.
- Low-Pressure Stage: Final expansion, where the steam reaches its lowest pressure before exiting the turbine.
3. Types of Steam Expansion in Turbines
There are two main types of steam expansion mechanisms, depending on the design of the turbine: impulse expansion and reaction expansion.
Impulse Expansion
- In impulse turbines, the steam expands entirely within the nozzles before it reaches the blades. The high-speed jet of steam strikes the blades, causing them to move.
- The blades are shaped to redirect the steam, but they do not cause further expansion.
- Characteristics:
- Steam pressure drops only in the nozzles, not in the blades.
- Common in applications where high-speed rotation is needed.
- Example: Curtis turbines and Rateau turbines are types of impulse turbines that use staged impulse expansion for better energy extraction.
Reaction Expansion
- In reaction turbines, steam expands as it moves through both the stationary and moving blades. The stationary blades act like nozzles, directing and expanding the steam, while the moving blades extract energy.
- Characteristics:
- Steam pressure drops gradually across the blades, not just in the nozzles.
- Creates both lift and thrust, similar to how a jet engine operates.
- More efficient for continuous energy extraction, especially in multi-stage designs.
- Example: Commonly used in large power plants due to their ability to handle varying loads.
4. The Thermodynamics of Steam Expansion
The expansion of steam is governed by the principles of thermodynamics, specifically the Rankine cycle. Understanding this cycle is key to optimizing turbine performance.
The Rankine Cycle:
- Boiler (Heat Addition): Water is heated to produce high-pressure steam.
- Turbine (Expansion): The steam expands in the turbine, doing work and causing the rotor to spin. This is the core of the energy conversion process.
- Condenser (Cooling): After passing through the turbine, the low-pressure steam is cooled and condensed back into water.
- Pump (Pressurization): The water is pumped back to high pressure before entering the boiler, completing the cycle.
Isentropic Expansion:
- Ideally, steam expansion in a turbine is isentropic, meaning it occurs without any loss of entropy (or heat exchange with the surroundings). This makes the process more efficient.
- In reality, some energy loss occurs due to friction, heat loss, and other inefficiencies. Efforts to minimize these losses are essential for improving turbine efficiency.
5. Improving Efficiency Through Controlled Expansion
Enhancing the expansion process can significantly improve the efficiency of a steam turbine. Here are a few methods used:
Superheated Steam
- Using superheated steam ensures that the steam has more energy, which means more work can be done during expansion. Superheating prevents condensation during the expansion process, which would otherwise reduce efficiency.
- Benefits: Increased energy output, reduced risk of corrosion in the turbine.
Reheat Cycles
- In a reheat cycle, steam is partially expanded in the turbine, then reheated and passed through the turbine again for further expansion. This increases the overall efficiency by allowing more energy to be extracted from the steam.
- Example: Often used in large power plants for high-efficiency power generation.
Regenerative Feedwater Heating
- In regenerative systems, some of the steam from the turbine is extracted to preheat the water entering the boiler. This reduces the energy required to convert the water into steam, thus enhancing the efficiency of the steam cycle.
- Benefits: Improved fuel efficiency and reduced operational costs.
6. Applications and Benefits of Steam Expansion
The concept of steam expansion is widely applied in several sectors:
- Power Generation:
- Most thermal power plants rely on steam expansion to generate electricity. By effectively converting thermal energy into mechanical energy, these plants can produce large amounts of power.
- Combined cycle power plants use steam turbines in conjunction with gas turbines, utilizing steam expansion to maximize efficiency.
- Marine Propulsion:
- Steam expansion drives the turbines that propel ships, especially in older naval vessels and some specialized modern ships. The use of steam turbines enables smooth and efficient long-distance travel.
- Industrial Processes:
- Industries that require mechanical power or process steam (e.g., chemical, food processing, paper) use steam expansion systems to drive machinery and equipment.
- Cogeneration (CHP systems): Steam expansion in turbines can produce both electricity and process steam, making it a highly efficient option for industries.
7. Challenges in Steam Expansion
Despite its effectiveness, there are challenges associated with steam expansion:
- Condensation During Expansion:
- If the steam cools too much during expansion, it can condense into water droplets, causing damage to turbine blades and reducing efficiency. Superheating the steam and maintaining optimal pressure can mitigate this issue.
- Material Stress:
- Turbine components must withstand high temperatures and pressures. Over time, the expansion process can cause wear and tear, requiring robust materials and regular maintenance.
- Thermal Losses:
- Energy losses due to friction and imperfect insulation can occur, reducing the overall efficiency. Advanced designs and materials are used to minimize these losses.
Conclusion
Steam expansion is a vital process that underpins the operation of steam turbines, enabling the efficient conversion of thermal energy into mechanical power. By carefully managing the expansion of steam, power plants and industrial facilities can optimize their energy use, improve efficiency, and reduce costs. Through advancements like superheated steam, reheat cycles, and multi-stage turbines, engineers continue to find ways to enhance the steam expansion process, making it a reliable and sustainable choice for power generation worldwide.
Conversion to Mechanical Energy in Steam Turbines
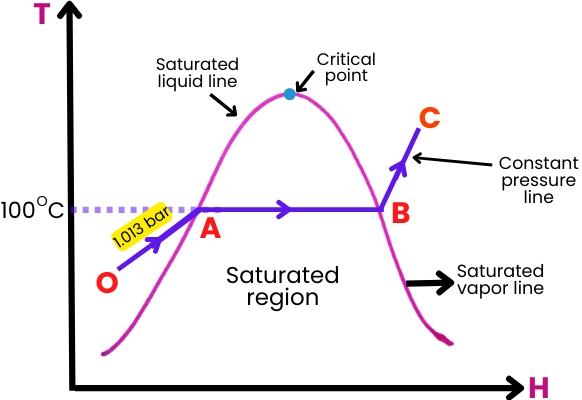
The conversion of steam’s thermal energy into mechanical energy is the fundamental process that powers steam turbines. This mechanism lies at the heart of generating electricity and driving machinery in various industrial applications. In this section, we’ll explore how steam turbines convert the expansive energy of steam into mechanical motion, the principles behind it, and the key factors that influence efficiency.
1. Basic Concept of Energy Conversion
The conversion process in a steam turbine involves several key stages:
- High-Energy Steam Generation:
- Steam is generated in a boiler, where water is heated to create high-pressure, high-temperature steam. This steam contains a significant amount of thermal energy (heat energy).
- Expansion and Energy Extraction:
- The high-energy steam is directed into the turbine, where it expands and its pressure decreases. As the steam expands, its thermal energy is converted into kinetic energy, creating high-speed jets of steam.
- Blade Interaction and Mechanical Motion:
- The fast-moving steam jets strike the blades of the turbine, causing them to spin. The spinning blades transfer their motion to the turbine’s rotor, converting the kinetic energy of the steam into rotational mechanical energy.
- This rotation can then be used to drive a generator for electricity production or other mechanical systems like pumps and compressors.
2. The Physics Behind the Conversion Process
The conversion of thermal energy to mechanical energy in a steam turbine is governed by the principles of thermodynamics and fluid dynamics. Here’s a detailed explanation:
Thermodynamics:
- The process of converting steam energy to mechanical energy is an application of the first law of thermodynamics, which states that energy cannot be created or destroyed but can change forms.
- Thermal to Mechanical Conversion:
- When steam enters the turbine, it has high enthalpy (a measure of total energy, including both pressure and thermal energy).
- As the steam expands and cools, its enthalpy decreases, and this loss of energy is converted into work, which drives the turbine blades.
Fluid Dynamics:
- Nozzle Action:
- The process begins with steam passing through nozzles, where it undergoes a rapid pressure drop. The nozzles convert the steam’s thermal energy into kinetic energy, resulting in a high-velocity steam jet.
- The nozzles are designed to precisely control the flow of steam, ensuring that it hits the turbine blades at the optimal angle and velocity.
- Impulse and Reaction Forces:
- When the steam jet strikes the turbine blades, it transfers its kinetic energy to the blades, causing them to move. The way this energy transfer occurs depends on the type of turbine:
- Impulse Turbine: The steam’s velocity creates a direct push on the blades.
- Reaction Turbine: The steam flows through the blades, generating lift and thrust (similar to how an airplane wing operates).
- When the steam jet strikes the turbine blades, it transfers its kinetic energy to the blades, causing them to move. The way this energy transfer occurs depends on the type of turbine:
3. Impulse vs. Reaction Turbines in Energy Conversion
Steam turbines can be broadly classified into impulse turbines and reaction turbines based on how they convert steam energy into mechanical energy. Each type uses different principles of fluid dynamics to extract energy.
Impulse Turbines:
- In an impulse turbine, the steam is expanded completely in the nozzles before it reaches the blades. The high-speed steam jet strikes the blades, exerting a direct force (impulse) that causes the blades to move.
- Energy Conversion Mechanism:
- The conversion occurs mainly because of the change in the steam’s direction as it hits the blades.
- The blades are designed to redirect the steam, and this change in momentum results in a push that rotates the turbine rotor.
- Example: The Curtis turbine is a common type of impulse turbine that uses multiple stages for improved energy extraction.
Reaction Turbines:
- In reaction turbines, the steam expands as it passes through both the stationary and moving blades, and energy is extracted continuously throughout this process.
- Energy Conversion Mechanism:
- Both the stationary and moving blades act like nozzles. The steam accelerates as it moves through these nozzles, and the reactive force from the steam’s expansion pushes against the blades, causing them to spin.
- The conversion is more gradual and occurs due to a combination of thrust and pressure drop across the blades.
- Example: The Parsons turbine is a well-known reaction turbine used in many large power plants.
4. Key Components That Facilitate Energy Conversion
Several components of the steam turbine system play crucial roles in the conversion of steam energy to mechanical energy:
- Nozzles:
- Nozzles are the parts where steam expands and accelerates, converting thermal energy into kinetic energy. This kinetic energy is essential for creating the force needed to turn the blades.
- Blades (Rotors and Stators):
- Rotating Blades (Rotors): These are attached to the turbine shaft and directly convert the steam’s kinetic energy into mechanical energy by spinning.
- Stationary Blades (Stators): These help direct and control the steam flow, optimizing the angle and speed at which the steam hits the rotor blades.
- Rotor:
- The rotor is the central shaft that holds the rotating blades. As the blades spin, they cause the rotor to turn, which in turn drives any attached machinery, such as a generator.
- Casing:
- The casing contains the entire assembly and directs the flow of steam, ensuring that it moves efficiently through the turbine. The design of the casing also helps maintain pressure and prevent leaks.
5. Efficiency Factors in Energy Conversion
The efficiency of converting thermal energy into mechanical energy in steam turbines is influenced by several factors:
Steam Conditions:
- Pressure and Temperature:
- Higher pressure and superheated steam can carry more energy, leading to greater efficiency. This is why many modern turbines use superheated steam to maximize energy extraction.
- Steam Quality:
- Ensuring the steam remains dry (without moisture) is crucial. Moisture in the steam can cause damage to the blades and reduce efficiency.
Turbine Design:
- Multi-Stage Turbines:
- Multi-stage turbines use several sets of blades, allowing steam to expand in stages. This staged approach enables more gradual energy extraction, improving efficiency and reducing energy losses.
- Blade Aerodynamics:
- The shape and design of the blades are essential for effective energy conversion. Well-designed blades can reduce drag, improve the transfer of kinetic energy, and minimize energy losses.
Minimizing Mechanical Losses:
- Friction and Drag:
- Friction between moving parts and drag on the blades can reduce the efficiency of the turbine. High-quality materials and precision engineering help minimize these mechanical losses.
- Lubrication:
- Proper lubrication of moving parts reduces friction, prolonging the life of the turbine and maintaining efficiency.
6. Applications of Mechanical Energy Conversion
The mechanical energy generated by steam turbines can be used in a variety of applications:
- Electricity Generation:
- The most common use of mechanical energy from turbines is in power plants. The turbine is connected to a generator, and as the rotor spins, it drives the generator to produce electricity.
- Example: Coal-fired, nuclear, and natural gas power plants all use steam turbines for electricity generation.
- Industrial Machinery:
- Steam turbines can also directly drive mechanical equipment such as compressors, pumps, and mills in various industries.
- Example: Oil refineries and chemical plants often use steam turbines to power large mechanical systems.
- Marine Propulsion:
- Steam turbines have been widely used in marine propulsion systems, particularly in large ships and naval vessels. The turbines convert steam energy into mechanical power to turn the propellers, driving the ship forward.
- Example: Many naval vessels, including aircraft carriers and submarines, use steam turbines for propulsion.
7. Challenges in Steam Energy Conversion
Despite the advantages of steam turbines, there are challenges associated with the conversion process:
- Energy Losses:
- Mechanical losses due to friction and drag can reduce the efficiency of energy conversion. Engineers must carefully design components to minimize these losses.
- Thermal Efficiency:
- The efficiency of the conversion process is limited by thermodynamic principles. Even the most efficient turbines cannot convert all the thermal energy into mechanical energy.
- Material Stress:
- The high-pressure and high-temperature conditions within a steam turbine can cause stress on materials, leading to wear and the need for maintenance.
Conclusion
The conversion of thermal energy from steam into mechanical energy is a fundamental process that drives much of the world’s electricity generation and industrial machinery. By harnessing the principles of thermodynamics and fluid dynamics, steam turbines effectively convert the expansive force of steam into rotational motion. Through careful design, efficient blade geometry, and precision engineering, modern steam turbines can achieve high efficiency, making them a cornerstone of energy production worldwide. Advances in materials, aerodynamics, and control systems continue to improve the efficiency and reliability of these machines, ensuring their role in the future of energy.
Exhaust and Condensation in Steam Turbines
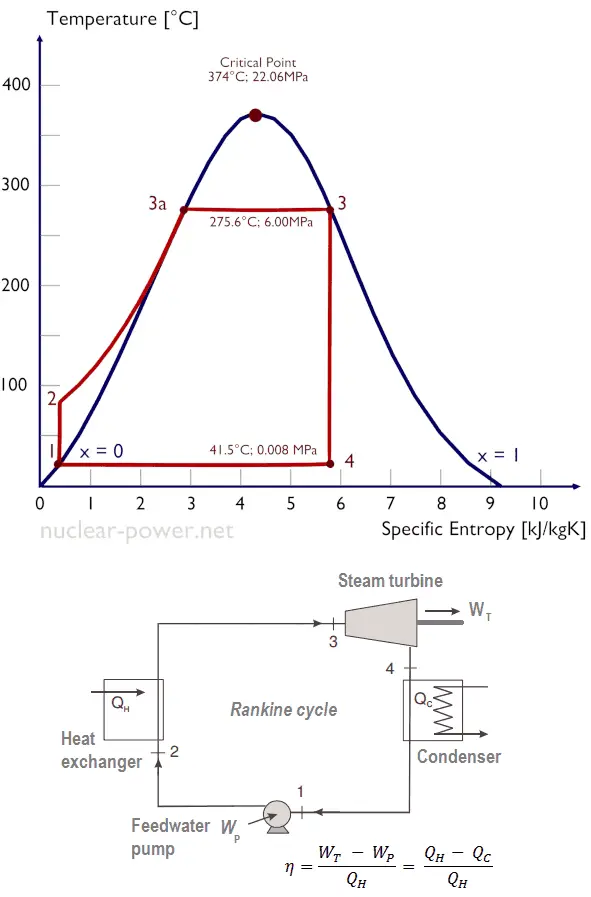
The exhaust and condensation processes are essential stages in the steam turbine cycle, completing the conversion of steam energy into mechanical energy and preparing the system for continuous operation. After steam has passed through the turbine and transferred its energy to drive the turbine blades, it needs to be managed efficiently to maximize the overall efficiency of the power plant or industrial process. This involves cooling the low-pressure steam, converting it back to water (condensate), and recycling it within the system. Here’s a detailed look at how these processes work and their importance.
1. What Happens During Exhaust?
After the steam has expanded and passed through the turbine blades, it exits as low-pressure, low-temperature steam. This is known as exhaust steam. The steam no longer has enough energy to do useful work, so it must be removed from the turbine and prepared for reuse.
Exhaust Process in the Turbine:
- The steam exiting the last stage of the turbine is at much lower pressure and temperature than when it entered.
- In many power plants, this exhaust steam is directed into a condenser, where it will be cooled and condensed back into water.
- Proper exhaust management is crucial for maintaining the efficiency of the steam cycle. If the exhaust steam is not efficiently removed, it can create back pressure on the turbine, reducing its efficiency.
Low-Pressure and Back Pressure Management:
- When steam exhausts from the turbine, it is important to maintain a low back pressure. Lowering the pressure in the exhaust region of the turbine allows the steam to expand more fully, extracting more energy from it.
- Back pressure refers to the resistance faced by the steam as it exits the turbine. Effective management of back pressure ensures that the turbine can continue to operate efficiently without unnecessary strain.
2. Role of the Condenser
The condenser is a critical component in the steam cycle, particularly in power plants. It cools the exhaust steam and converts it back into liquid water, a process known as condensation. This allows the water to be recycled and used again in the boiler, completing the steam cycle. The primary functions of the condenser are:
- Cooling the Exhaust Steam:
- The condenser receives the low-pressure steam from the turbine and uses cooling water (often drawn from a nearby river, lake, or cooling tower system) to lower the steam temperature.
- The cooling process causes the steam to lose energy and transition from a gaseous state back into liquid water.
- Maintaining Low Pressure:
- By condensing the steam, the condenser creates a vacuum or low-pressure environment, which helps draw more steam out of the turbine. This vacuum reduces back pressure, allowing the turbine to operate more efficiently.
- Maintaining a low-pressure zone ensures that the steam continues to expand as much as possible before it is exhausted, leading to better energy extraction.
- Water Recycling:
- The condensed water (often called condensate) is collected and sent back to the boiler for reheating, creating a closed-loop system that minimizes water waste and improves efficiency.
3. How Condensation Works
Condensation is the process of cooling the exhaust steam to the point where it changes from a vapor (gas) to a liquid. Here’s how it happens in the condenser:
- Heat Exchange:
- The condenser acts as a heat exchanger. Inside the condenser, the exhaust steam flows over tubes filled with cool water.
- The cooler water absorbs heat from the steam, causing the steam to cool and condense.
- Formation of Condensate:
- As the steam cools, it loses its thermal energy and condenses into water droplets. This water is collected at the bottom of the condenser and is called condensate.
- The efficiency of the condensation process is vital for maintaining a low-pressure environment in the condenser, which in turn enhances the turbine’s performance.
- Cooling Water Cycle:
- The cooling water that absorbs the heat from the steam is either released back to its source or sent to a cooling tower, where it is cooled and recirculated.
- In power plants located near water bodies, large quantities of water may be drawn from the source, cooled, and then returned, adhering to environmental regulations to avoid thermal pollution.
4. Types of Condensers
Condensers can vary in design, but the two main types commonly used in steam power plants are:
Surface Condensers:
- In surface condensers, the steam passes over a series of tubes that contain cool water. The water inside the tubes does not mix with the steam; it only absorbs the heat from it.
- Advantages:
- The steam and cooling water remain separate, allowing for the reuse of treated boiler water.
- Easier to maintain and control for efficiency.
Direct Contact Condensers:
- In direct contact condensers, the steam comes into direct contact with the cooling water, causing immediate condensation.
- Advantages:
- Simple and efficient heat exchange.
- Suitable for processes where the mixing of steam and water is acceptable.
5. Importance of Effective Condensation
Efficient condensation is crucial for several reasons:
- Improved Efficiency:
- By creating a low-pressure zone, condensers allow steam turbines to extract as much energy as possible from the steam. Lowering the pressure at the turbine’s exhaust enables the steam to expand more, which improves the overall efficiency of the power plant.
- The conversion of steam to water also reduces the volume, which helps in maintaining the flow dynamics within the system.
- Water Conservation:
- The process of condensing steam back into water allows for water to be reused, reducing the need for continuous freshwater supply. This is both cost-effective and environmentally friendly.
- Preventing Damage:
- Without a proper condensation system, residual steam in the turbine could cause damage to the blades due to moisture buildup, leading to erosion and corrosion.
- Moisture in the turbine can also reduce efficiency by creating drag on the blades, affecting the turbine’s performance.
6. Cooling Systems for Condensation
Condensers rely on efficient cooling systems to maintain their performance. Here are some common cooling methods:
Cooling Towers:
- Cooling towers are used to cool the water that has been heated by the steam in the condenser. The water is sprayed inside the tower, where it comes into contact with air, causing some of the water to evaporate and cool.
- Benefits: Reduces the amount of cooling water needed and prevents thermal pollution of natural water bodies.
Once-Through Cooling:
- In this system, water is taken from a natural source (like a river or ocean), passed through the condenser to cool the steam, and then released back.
- Benefits: Simple and efficient, but it must comply with regulations to minimize the impact on aquatic ecosystems.
7. Challenges in Condensation and Exhaust Management
The exhaust and condensation stages are efficient but not without challenges:
- Thermal Pollution:
- Releasing hot water back into natural water bodies can cause thermal pollution, affecting aquatic life. Power plants need to ensure that water is cooled to acceptable levels before being discharged.
- Scaling and Fouling:
- The condenser tubes can accumulate deposits (scaling) or biofouling (growth of algae and microorganisms) over time. This reduces the heat exchange efficiency and requires regular maintenance.
- Vacuum Loss:
- Maintaining a vacuum in the condenser is critical for efficient steam extraction. Any leaks or inefficiencies can lead to a drop in vacuum pressure, reducing turbine efficiency and performance.
8. The Role of Vacuum Pumps and Ejectors
To maintain the vacuum inside the condenser and ensure continuous operation, vacuum pumps or steam ejectors are used. These devices remove any air or non-condensable gases that might accumulate in the condenser, which can disrupt the vacuum and lead to inefficiencies.
- Vacuum Pumps:
- Mechanically remove air and gases to maintain the desired vacuum level.
- Steam Ejectors:
- Use high-pressure steam to create a suction effect, pulling non-condensable gases out of the condenser.
Conclusion
The exhaust and condensation processes are integral to the efficiency of steam turbines and power plants. By effectively removing and cooling the spent steam, condensers create a low-pressure environment that enhances turbine performance and allows the water to be recycled. Maintaining efficient condensation not only improves energy efficiency but also conserves water, reduces environmental impact, and minimizes the risk of damage to turbine components. Through proper design, regular maintenance, and adherence to environmental standards, modern power plants can optimize these processes, ensuring reliable and sustainable energy production.
Types of Steam Turbines: An Overview
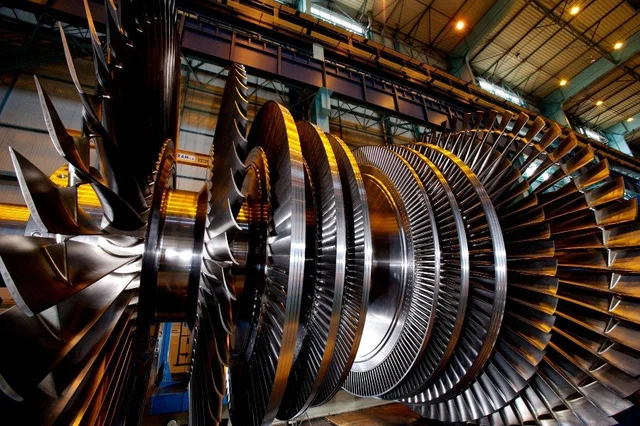
Steam turbines are essential devices in power generation and industrial processes, converting the energy from steam into mechanical motion. Depending on their design and application, steam turbines can vary widely in terms of how they operate, their efficiency, and where they are best suited. Understanding the different types of steam turbines helps in selecting the right equipment for various energy and mechanical needs.
The classification of steam turbines is typically based on factors like the working principle, stage configuration, and steam flow direction. Here’s a detailed exploration of the different types of steam turbines:
1. Classification Based on Working Principle
Steam turbines can be broadly classified into two types based on their working principles: Impulse Turbines and Reaction Turbines.
1.1 Impulse Turbines
- Working Principle:
- In an impulse turbine, steam is expanded in nozzles to form high-speed jets. These jets of steam strike the turbine blades, causing them to rotate. The pressure drop occurs entirely in the nozzles, not in the blades.
- The turbine blades convert the kinetic energy from the high-velocity steam into mechanical energy.
- Blade Design:
- The blades are bucket-shaped to efficiently redirect the steam flow, causing a change in momentum and creating a driving force.
- Features:
- Suitable for applications with high-pressure and high-velocity steam.
- Operates efficiently at partial loads.
- Typically used in small and medium-sized power generation setups.
- Examples:
- De Laval Turbine: A single-stage impulse turbine used for small-scale applications.
- Curtis Turbine: A multi-stage impulse turbine with multiple sets of nozzles and rotors for better efficiency.
- Rateau Turbine: A multi-stage design that uses pressure reduction across several stages.
1.2 Reaction Turbines
- Working Principle:
- In a reaction turbine, steam expands as it passes through both the stationary and rotating blades. The pressure drops gradually along the turbine as the steam flows through it, creating thrust that drives the blades.
- The blades act like nozzles, producing reactive force similar to how a jet engine works.
- Blade Design:
- The blades are shaped to create a continuous pressure drop as steam passes through them, maximizing energy extraction.
- Features:
- Operates smoothly over a range of loads, making it suitable for applications where power output may fluctuate.
- Commonly used in large power plants.
- Examples:
- Parsons Turbine: A well-known type of reaction turbine, often used in large-scale power plants.
- Francis Turbine (for hydro applications): Though primarily a water turbine, it operates on a similar principle to a reaction steam turbine.
2. Classification Based on Stage Configuration
Steam turbines can also be categorized by the number of stages they employ for steam expansion:
2.1 Single-Stage Turbines
- Description:
- These turbines have only one set of nozzles and blades for expanding steam.
- Suitable for smaller applications where low power output is required.
- Applications:
- Ideal for driving small machinery like pumps, compressors, and generators.
- Often used in industrial applications where localized power is needed.
2.2 Multi-Stage Turbines
- Description:
- Multi-stage turbines have multiple sets of blades and nozzles. The steam expands gradually across these stages, extracting energy more efficiently.
- The design allows for a more significant drop in steam pressure, which increases overall energy extraction.
- Applications:
- Commonly used in large power plants, including nuclear, coal, and gas-fired power stations.
- Suitable for applications where high power output and efficiency are critical.
3. Classification Based on Steam Flow Direction
The direction in which steam flows through the turbine also classifies the turbine types:
3.1 Axial Flow Turbines
- Description:
- Steam flows parallel to the axis of the turbine shaft. Most modern power-generating turbines are axial flow because this configuration allows for efficient multi-stage designs.
- Advantages:
- Capable of handling large volumes of steam, making them ideal for power plants.
- High efficiency and robust performance over varying loads.
3.2 Radial Flow Turbines
- Description:
- Steam enters the turbine radially (perpendicular to the axis) and flows out axially. Radial flow turbines are less common but can be found in specific applications.
- Advantages:
- Compact design, which is useful in smaller, localized applications.
- Simplified mechanical structure compared to axial flow designs.
4. Classification Based on Operational Conditions and Design
4.1 Condensing Turbines
- Description:
- These turbines exhaust steam into a condenser, where it is cooled and converted back into water. The condenser maintains a low-pressure environment, allowing the turbine to extract more energy from the steam.
- Applications:
- Widely used in thermal power plants to maximize efficiency and electricity output.
- The low-pressure exhaust allows for more energy extraction, making them ideal for large-scale electricity generation.
4.2 Non-Condensing (Back Pressure) Turbines
- Description:
- In these turbines, the steam exhausts at a higher pressure and is not condensed. The remaining steam is often used for heating or other industrial processes.
- Applications:
- Suitable for cogeneration (combined heat and power, or CHP) systems, where the exhaust steam is used for heating or industrial processes after it leaves the turbine.
- Common in industrial facilities that need both electricity and steam for their operations.
4.3 Extraction Turbines
- Description:
- Extraction turbines allow some steam to be diverted (extracted) at a specific point before it is fully expanded. The extracted steam can be used for heating, process steam, or other purposes.
- Applications:
- Ideal for combined cycle and cogeneration systems where both power and steam are needed.
- Used in industries like chemical processing, paper manufacturing, and district heating systems.
4.4 Reheat Turbines
- Description:
- These turbines include a reheating stage, where steam is sent back to the boiler for reheating after initial expansion. The reheated steam then returns to the turbine for further expansion.
- Reheating increases the steam temperature and prevents moisture from forming, which can damage turbine blades and reduce efficiency.
- Applications:
- Common in large-scale power plants, especially coal and nuclear, where maximizing efficiency is critical.
- Used in combined cycle power plants to optimize performance.
5. Classification Based on Size and Application
5.1 Industrial Steam Turbines
- Description:
- Industrial turbines are smaller in size and are used for localized power generation, typically ranging from a few megawatts (MW) to about 150 MW.
- Applications:
- Powering machinery, pumps, and compressors in factories.
- Used in smaller plants or facilities that require both electricity and process steam.
5.2 Utility (Power Generation) Steam Turbines
- Description:
- Large, utility-scale turbines are designed to generate electricity for the grid, ranging from several hundred megawatts to over a gigawatt.
- Applications:
- Found in thermal power plants (coal, nuclear, gas) that supply electricity on a large scale.
- Essential components of combined cycle plants, where they work in tandem with gas turbines to maximize efficiency.
5.3 Micro Steam Turbines
- Description:
- Micro turbines are small turbines used for very localized power generation, often producing less than 1 MW of power.
- Applications:
- Ideal for small industrial plants, residential use, and renewable energy projects (like biomass-based systems).
- Suitable for remote areas where connecting to the main grid is not feasible.
Conclusion
Steam turbines are versatile and can be classified in various ways, including their working principles (impulse vs. reaction), stage configurations (single-stage vs. multi-stage), steam flow directions (axial vs. radial), and operational conditions (condensing, non-condensing, extraction, and reheat). Each type of turbine has its advantages and specific applications, making them suitable for different industrial and power generation scenarios. Understanding these classifications helps in choosing the right turbine design, optimizing performance, and improving the efficiency of energy systems. As steam turbine technology continues to advance, innovations in efficiency, material science, and design will further enhance their role in sustainable energy production.
Impulse Turbines: Working Principle, Design, and Applications
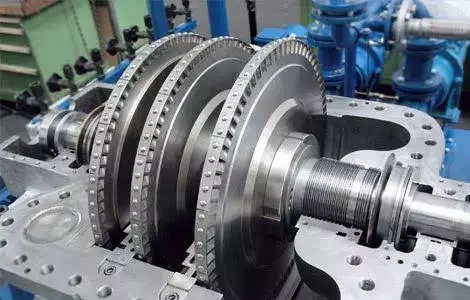
Impulse turbines are a type of steam turbine that operates based on the principle of impulse, where high-velocity steam jets are used to strike turbine blades, creating a mechanical force that drives the rotor. They are one of the two primary types of steam turbines (the other being reaction turbines) and are widely used in various industrial applications, including power generation. Below, we explore the working mechanism, design characteristics, advantages, and applications of impulse turbines.
1. Working Principle of Impulse Turbines
The operation of an impulse turbine is based on the concept of impulse, which involves the conversion of the steam’s thermal energy into kinetic energy through nozzles. Here’s a step-by-step breakdown of how impulse turbines work:
- High-Pressure Steam Generation:
- Steam is generated in a boiler at high pressure and temperature. This steam is then directed to the turbine.
- Before entering the turbine, the steam passes through a set of nozzles.
- Steam Expansion and Nozzle Action:
- The nozzles are designed to convert the steam’s thermal and pressure energy into kinetic energy. This process involves a significant pressure drop, resulting in high-velocity steam jets.
- The nozzles accelerate the steam, converting its pressure energy into a high-speed jet of steam.
- Impact on Blades (Impulse Action):
- The high-speed steam jets are directed onto the impulse blades of the turbine rotor. The blades are bucket-shaped, designed to capture the kinetic energy of the steam.
- When the steam hits the blades, it changes direction, and this change in momentum generates a force that causes the blades (and the rotor) to spin.
- The energy conversion in an impulse turbine occurs solely because of the change in the direction of the steam, without any pressure drop across the blades. All pressure drop happens in the nozzles before the steam reaches the blades.
- Continuous Energy Transfer:
- The rotor’s rotation is continuous as long as the steam flow is maintained, providing a steady mechanical output that can be used to generate electricity or power mechanical equipment.
2. Design and Components of Impulse Turbines
The design of an impulse turbine is relatively simple compared to reaction turbines, consisting of several key components:
Nozzles
- The nozzles play a crucial role in converting the steam’s thermal energy into kinetic energy. They are responsible for creating high-velocity jets of steam that can effectively strike the turbine blades.
- Proper nozzle design ensures efficient energy conversion and optimal performance of the turbine.
Rotor and Blades
- The rotor holds the turbine blades, which are typically bucket-shaped to efficiently redirect the steam flow.
- Impulse Blades:
- The blades are shaped in a way that allows them to capture the kinetic energy of the steam and change its direction. This change in direction creates a reaction force, causing the blades to spin.
- Because the steam pressure does not drop across the blades (only its velocity changes), impulse blades are more robust and simpler in design than reaction blades.
Casing
- The casing encloses the turbine and directs the flow of steam onto the nozzles and blades, ensuring that the steam moves efficiently through the system.
- The casing also serves to contain the pressure within the system, preventing steam leakage and maintaining operational efficiency.
3. Types of Impulse Turbines
Several variations of impulse turbines are designed to meet different energy requirements. The most common types include:
3.1 De Laval Turbine
- Description:
- The De Laval turbine is a simple, single-stage impulse turbine. It was one of the earliest types of steam turbines developed and is known for its straightforward design.
- It uses a single set of nozzles and blades, where the steam expands fully in the nozzles before striking the blades.
- Applications:
- Small-scale applications, such as driving pumps, compressors, and small generators.
3.2 Curtis Turbine (Two-Stage Impulse Turbine)
- Description:
- The Curtis turbine, also known as a velocity-compounded impulse turbine, uses multiple sets of blades and nozzles arranged in stages. The first stage is a set of nozzles that accelerates the steam, followed by two or more rows of moving blades.
- This design allows for efficient energy extraction in a compact form, using multiple sets of blades to capture the energy of the steam.
- Applications:
- Medium-scale power generation, industrial applications where efficiency and compact design are essential.
3.3 Rateau Turbine (Pressure-Compounded Impulse Turbine)
- Description:
- The Rateau turbine employs a series of stages, where each stage consists of a nozzle followed by a row of blades. Steam expands gradually across multiple stages, with a slight pressure drop at each stage.
- This design allows for more controlled and efficient energy extraction, particularly when dealing with high-pressure steam.
- Applications:
- Larger power plants where higher efficiency and power output are required.
3.4 Multi-Stage Impulse Turbines
- Description:
- Multi-stage impulse turbines combine several stages of nozzles and blades to allow steam to expand and transfer energy across multiple phases.
- By using multiple stages, these turbines can handle high-pressure steam more effectively, extracting more energy and improving overall efficiency.
- Applications:
- Large power plants, industrial facilities, and marine propulsion.
4. Advantages of Impulse Turbines
Impulse turbines offer several benefits, which make them suitable for specific applications:
- Simple Design and Construction:
- Impulse turbines have a straightforward design, making them easier to manufacture and maintain. The simple blade design reduces production costs and facilitates repairs.
- Efficiency at High-Speed Operation:
- The design of impulse turbines allows them to operate efficiently at high speeds, making them ideal for applications that require fast rotation rates, such as small power generators and industrial machinery.
- Adaptability to Different Steam Conditions:
- Impulse turbines are capable of handling a wide range of steam conditions, including high-pressure and high-temperature steam. This adaptability allows them to be used in diverse industrial setups.
- Partial Load Efficiency:
- Impulse turbines can maintain efficiency even when operating at partial loads. This makes them suitable for applications where power demand may fluctuate.
- Durability and Reliability:
- The robust design of impulse blades ensures durability, even under harsh operating conditions. They are less susceptible to wear and tear, contributing to long service life and reliability.
5. Applications of Impulse Turbines
Impulse turbines find applications across various sectors due to their versatility and efficiency. Some of the common applications include:
- Power Generation:
- Impulse turbines are often used in small and medium-scale power plants where high-speed operation is required. They can be coupled with generators to produce electricity for local grids or industrial facilities.
- They are particularly popular in hydroelectric power plants (where the turbine is driven by water instead of steam) because of their efficiency at high speeds.
- Industrial Machinery:
- In industries, impulse turbines are used to drive machinery such as pumps, compressors, and blowers. Their ability to handle high-pressure steam makes them ideal for powering heavy equipment.
- Marine Propulsion:
- Impulse turbines are sometimes used in marine vessels, where they help power auxiliary systems or serve as backup power sources.
- Process Steam Applications:
- Industries that generate process steam can use impulse turbines to extract energy from excess steam, improving overall plant efficiency. This is particularly useful in cogeneration (combined heat and power) systems.
6. Challenges and Limitations
While impulse turbines have many advantages, they also come with certain challenges:
- Efficiency at Lower Speeds:
- Impulse turbines are most efficient at high speeds. At lower speeds, their efficiency may drop, making them less suitable for applications requiring variable speeds.
- Limited Expansion Stages:
- Impulse turbines are less efficient when extensive expansion of steam is required. For applications that need more gradual expansion, reaction turbines are often preferred.
- Vibration Issues:
- At very high speeds, impulse turbines can experience vibrations that may lead to mechanical stress. Proper maintenance and design modifications are necessary to address this issue.
Conclusion
Impulse turbines are a crucial part of the steam turbine family, known for their straightforward design, adaptability to high-pressure steam, and efficiency at high speeds. They are ideal for applications ranging from small-scale power generation to industrial machinery and marine systems. The simple yet robust construction of impulse turbines ensures reliable performance, making them a preferred choice for many industrial setups. Understanding the working principles, design elements, and advantages of impulse turbines is essential for selecting the right equipment to optimize energy conversion and efficiency in various applications.
Reaction Turbines: Working Principle, Design, and Applications
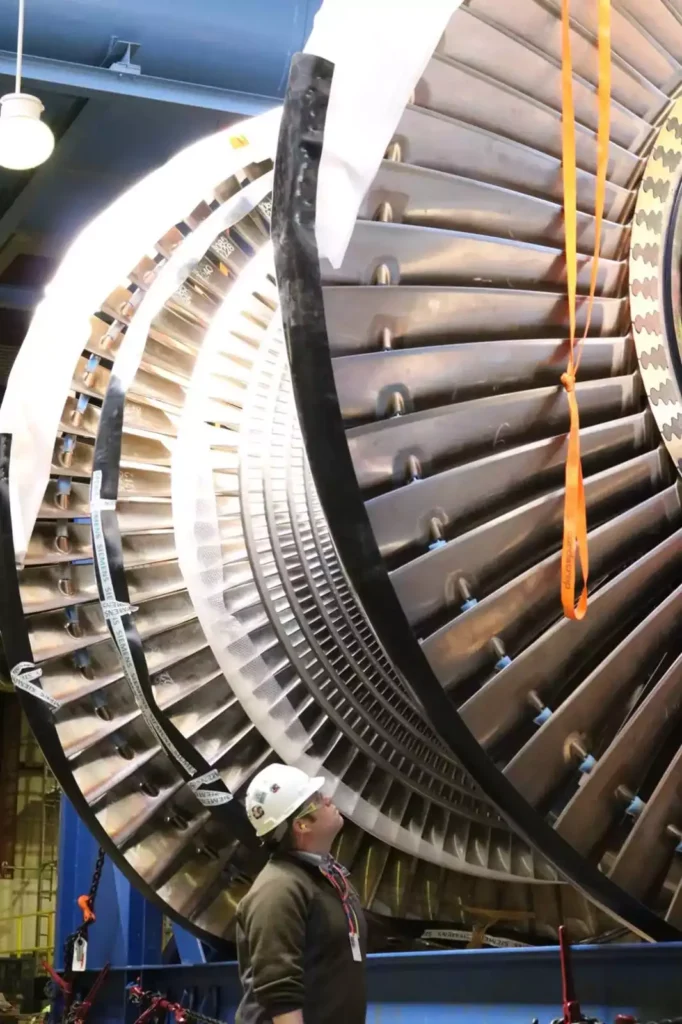
Reaction turbines are a type of steam turbine that operate on the principle of both impulse and reaction. Unlike impulse turbines, where the steam’s energy is converted into kinetic energy through nozzles before it hits the blades, reaction turbines use a continuous pressure drop across both stationary and moving blades, creating a reaction force that drives the turbine. Reaction turbines are commonly used in large-scale power plants due to their ability to handle varying loads efficiently and extract more energy from the steam.
This section explores how reaction turbines work, their design features, types, advantages, and their practical applications.
1. Working Principle of Reaction Turbines
Reaction turbines work on the principle of Newton’s Third Law: “For every action, there is an equal and opposite reaction.” Here’s how the process unfolds:
- Steam Expansion Across Blades:
- In reaction turbines, steam expands as it flows through both the fixed (stationary) and moving blades.
- As the steam passes through the fixed blades (which act as nozzles), it accelerates, partially expanding and losing pressure. This causes an increase in its velocity.
- The steam then enters the moving blades, which are shaped to allow further expansion and acceleration. The pressure drop continues across the moving blades, which contributes to the creation of a reaction force that drives the blades.
- Action and Reaction:
- The design of the blades ensures that when steam flows through them, it produces a reactive force that pushes the blades forward, similar to how a jet engine works.
- The combined effect of the impulse from the stationary blades and the reaction from the moving blades results in the rotation of the turbine rotor.
- Continuous Pressure Drop:
- Unlike impulse turbines, where the pressure drop occurs only in the nozzles, reaction turbines experience a gradual and continuous pressure drop as the steam moves through both sets of blades. This allows for efficient energy extraction across multiple stages.
- Multi-Stage Expansion:
- Reaction turbines are usually designed with multiple stages to ensure maximum energy extraction. Each stage consists of a row of fixed blades followed by a row of moving blades, and the steam expands gradually across these stages.
- The multi-stage configuration makes reaction turbines ideal for applications where high power output is needed, such as in large power plants.
2. Design and Components of Reaction Turbines
Reaction turbines have a more complex design compared to impulse turbines, as they rely on both the impulse and reaction principles. Here are the key components:
Fixed (Stationary) Blades
- These blades are fixed to the turbine casing and act like nozzles, guiding and accelerating the steam as it passes through. The fixed blades also direct the steam onto the moving blades at the optimal angle for energy extraction.
- The stationary blades help control the steam flow, ensuring that it expands gradually and evenly, maximizing efficiency.
Moving Blades
- The moving blades are attached to the rotor, which spins as the blades receive the reaction force from the steam. The shape of the blades allows for continuous expansion and pressure drop, creating a reactive force that drives the rotation.
- The design of the moving blades is crucial for optimizing the energy extraction process, as it must effectively manage both the pressure drop and the direction of the steam flow.
Rotor
- The rotor is the central shaft to which the moving blades are attached. As the blades spin, the rotor converts the steam’s kinetic and pressure energy into mechanical energy, which can then be used to drive generators or other equipment.
Casing
- The casing encloses the turbine and supports the stationary blades. It also directs the flow of steam through the turbine stages, ensuring efficient energy conversion. The casing must be designed to withstand the high pressures and temperatures associated with the steam flow.
3. Types of Reaction Turbines
There are several variations of reaction turbines, each designed to suit specific operational requirements:
3.1 Parsons Turbine (Axial Flow Reaction Turbine)
- Description:
- The Parsons turbine, developed by Sir Charles Parsons, is one of the earliest and most common types of reaction turbines. It features a multi-stage design, where steam passes through multiple rows of stationary and moving blades, allowing for gradual expansion and pressure reduction.
- The steam flows axially, meaning parallel to the shaft, making it an axial flow turbine.
- Applications:
- Widely used in power plants for electricity generation, especially in nuclear and coal-fired plants.
- The multi-stage design makes it highly efficient for large-scale energy production.
3.2 Kaplan Turbine (Water-Based Reaction Turbine)
- Description:
- While primarily a water turbine, the Kaplan turbine operates on similar principles as a steam reaction turbine. It uses adjustable blades to manage the flow and pressure of water, converting it into mechanical energy.
- Applications:
- Hydroelectric power plants where water flow can vary, and efficiency must be maintained across different flow conditions.
4. Advantages of Reaction Turbines
Reaction turbines have several benefits, which make them suitable for specific applications, particularly large-scale power generation:
- High Efficiency at Varying Loads:
- Reaction turbines can maintain high efficiency even when there are fluctuations in power demand. This makes them ideal for applications where the load varies throughout the day, such as in electricity grids.
- The continuous pressure drop across multiple stages allows for better energy extraction compared to impulse turbines, especially at lower pressures.
- Gradual Expansion for Better Energy Utilization:
- The design of reaction turbines allows for the steam to expand gradually across multiple stages, extracting more energy from the steam than would be possible in a single-stage configuration. This makes them highly efficient for converting thermal energy to mechanical energy.
- Smooth and Stable Operation:
- The multi-stage configuration ensures that the turbine operates smoothly, with less vibration and noise compared to impulse turbines. This stability makes reaction turbines reliable for long-term, continuous operation.
- Scalability:
- Reaction turbines can be scaled up for very large power plants, making them suitable for generating hundreds of megawatts to gigawatts of electricity. This scalability is one of the reasons they are widely used in major power plants around the world.
5. Applications of Reaction Turbines
Reaction turbines are versatile and find applications across various sectors:
- Power Generation:
- The most common use of reaction turbines is in power generation, particularly in nuclear and coal-fired power plants. Their ability to handle high-pressure, high-temperature steam and operate efficiently over a range of loads makes them ideal for large-scale electricity production.
- They are also used in combined cycle power plants, where they complement gas turbines by using the waste heat from the gas turbine to produce steam for the reaction turbine, enhancing overall efficiency.
- Marine Propulsion:
- Reaction turbines have been used in naval ships and large commercial vessels for propulsion. The smooth and steady output of reaction turbines is suitable for driving ship propellers.
- While modern ships mostly use diesel engines, some specialized vessels still rely on reaction turbines.
- Industrial Applications:
- Large industrial facilities that require both power and process steam can use reaction turbines in cogeneration systems. The reaction turbine generates electricity while also providing steam for heating, drying, or other industrial processes.
6. Challenges and Limitations
Despite their advantages, reaction turbines also have some challenges and limitations:
- Complex Design and Higher Cost:
- The design of reaction turbines is more complex than impulse turbines, requiring precise engineering and higher manufacturing costs. The complex blade design must be carefully managed to ensure optimal performance.
- Moisture Issues:
- As steam expands and cools in the turbine, it can start to condense into water droplets. These droplets can cause erosion on the turbine blades, leading to wear and damage. This issue is managed by superheating the steam or using moisture separators.
- Maintenance Requirements:
- The multi-stage design and continuous pressure drop make reaction turbines more susceptible to wear and tear, requiring regular maintenance to ensure efficient operation.
Comparison: Impulse vs. Reaction Turbines
Feature | Impulse Turbine | Reaction Turbine |
---|---|---|
Energy Conversion | Pressure drop occurs in nozzles, converting steam to high-speed jets. | Pressure drop occurs across both stationary and moving blades. |
Blade Design | Bucket-shaped blades for deflecting steam. | Aerofoil-shaped blades for smooth, continuous expansion. |
Efficiency | High efficiency at high speeds, less efficient at varying loads. | High efficiency over a range of loads. |
Applications | Small to medium power plants, industrial machinery. | Large power plants, combined cycle plants, marine propulsion. |
Complexity | Simpler design, easier to maintain. | More complex, requires precise engineering. |
Conclusion
Reaction turbines are a key technology in modern energy systems, offering efficient and reliable power generation, especially for large-scale applications. Their ability to handle varying loads, combined with high efficiency, makes them ideal for electricity production in power plants, marine propulsion, and industrial cogeneration. While they are more complex and require precise engineering, the benefits of smooth operation, scalability, and effective energy extraction outweigh the challenges. As the demand for efficient energy systems grows, reaction turbines continue to play a vital role in supporting sustainable power generation worldwide.
Single-Stage Turbines: Overview, Working Principle, and Applications
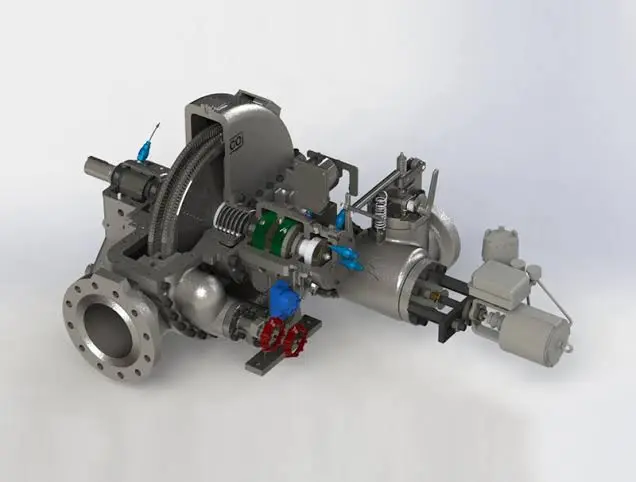
Single-stage turbines are a type of steam turbine where the steam expansion and energy extraction occur in a single set of nozzles and blades. Unlike multi-stage turbines, which use multiple stages for gradual expansion and energy conversion, single-stage turbines are designed for simpler, smaller-scale applications. Due to their straightforward design and ease of operation, they are commonly used in scenarios where compactness, lower power output, and cost-effectiveness are important.
This section explores how single-stage turbines work, their design features, benefits, and common applications.
1. What Is a Single-Stage Turbine?
A single-stage turbine refers to a turbine that has only one set of nozzles and one row of moving blades. In this design, the high-pressure steam expands through the nozzles, converting its thermal energy into kinetic energy, which then drives the turbine blades to generate mechanical energy. The energy conversion happens all at once in a single stage.
Key Characteristics:
- Single-stage turbines are compact, simple, and efficient for low to moderate power outputs.
- They are less complex than multi-stage turbines, making them easier to design, manufacture, and maintain.
- Suitable for applications where a high power output is not required, but reliability and ease of use are essential.
2. Working Principle of Single-Stage Turbines
The operation of a single-stage turbine is based on the impulse principle, although some single-stage turbines can also work on the reaction principle. Here’s how it works:
- Steam Generation and Supply:
- High-pressure, high-temperature steam is produced in a boiler and directed toward the turbine.
- The steam enters the turbine and passes through a set of nozzles.
- Nozzle Action (Impulse Design):
- In impulse turbines, the nozzles are designed to convert the steam’s pressure energy into high-velocity jets. This process involves a drop in steam pressure and an increase in kinetic energy.
- The high-speed steam jets are directed toward the turbine blades.
- Blade Rotation:
- The high-speed steam strikes the moving blades attached to the rotor, causing the blades to spin. The rotor’s rotation converts the steam’s kinetic energy into mechanical energy.
- Since it is a single-stage turbine, all the energy conversion occurs in this one interaction between the steam jet and the blades.
- Mechanical Output:
- The mechanical energy from the rotating rotor can be used to drive a generator to produce electricity or power other mechanical equipment such as pumps, compressors, or fans.
Reaction Design in Single-Stage Turbines:
- In a reaction-based single-stage turbine, the steam expands across both stationary and moving blades, creating a pressure drop and reactive force that drives the blades.
- However, these are less common compared to impulse single-stage turbines, which dominate in smaller-scale applications.
3. Design and Components of Single-Stage Turbines
The design of a single-stage turbine is relatively simple, which contributes to its popularity in smaller, decentralized power systems. Key components include:
Nozzles
- Nozzles play a critical role in the energy conversion process. They direct and accelerate the steam, converting pressure energy into kinetic energy.
- The design of the nozzles must ensure that steam exits at the correct velocity and angle to maximize the impact on the turbine blades.
Blades (Impulse or Reaction Blades)
- Impulse Blades: Bucket-shaped blades are common in single-stage impulse turbines. These blades are designed to change the direction of the steam jet, creating an impulse force that drives the rotor.
- Reaction Blades: Aerofoil-shaped blades may be used if the turbine is designed on the reaction principle, allowing steam to expand and create a reactive force that rotates the blades.
Rotor
- The rotor is the central shaft that holds the blades. It converts the energy from the steam into rotational motion, which can be transmitted to other mechanical systems or generators.
Casing
- The casing surrounds the turbine and holds the nozzles and blades in place. It also directs the steam flow to ensure that it hits the blades at the right angle and velocity.
4. Types of Single-Stage Turbines
While single-stage turbines can be designed based on both impulse and reaction principles, they are more commonly found in the impulse configuration due to the following types:
4.1 De Laval Turbine
- Description:
- One of the earliest designs of single-stage impulse turbines, developed by Gustaf de Laval. The De Laval turbine features a simple setup with a single set of nozzles and rotor blades.
- The steam is expanded completely in the nozzles, converting all pressure energy into kinetic energy before striking the blades.
- Applications:
- Small-scale power generation, driving pumps, fans, and compressors.
- Suitable for applications requiring high-speed operation.
4.2 Curtis Single-Stage Turbine
- Description:
- A variation of the impulse turbine design that can include a velocity-compounding feature, where the steam is directed to flow through more than one row of moving blades in a single stage. This helps manage the high velocities of steam jets.
- While multi-stage Curtis turbines exist, single-stage versions are used for smaller-scale applications.
- Applications:
- Industrial processes, where a compact and simple turbine design is required.
5. Advantages of Single-Stage Turbines
Single-stage turbines offer several benefits that make them suitable for specific applications:
- Simple Design and Low Cost:
- The simple design of single-stage turbines makes them easy to manufacture and maintain. Fewer components mean lower costs and fewer points of failure, leading to reduced maintenance requirements.
- Compact and Lightweight:
- Single-stage turbines are compact and lightweight compared to multi-stage turbines, making them ideal for situations where space is limited, such as in small industrial plants or mobile setups.
- High-Speed Operation:
- Impulse-based single-stage turbines can achieve high rotational speeds, making them suitable for driving devices that require fast, consistent power, such as centrifugal pumps and compressors.
- Ease of Maintenance:
- The simple construction of single-stage turbines allows for easy access to parts, making repairs and maintenance straightforward. This can reduce downtime and operational costs.
- Reliable for Smaller Applications:
- For smaller power outputs, single-stage turbines are highly efficient and reliable. They are often preferred for localized power generation where there is no need for complex multi-stage systems.
6. Applications of Single-Stage Turbines
Single-stage turbines are versatile and can be found in various settings. Common applications include:
- Industrial Processes:
- Single-stage turbines are often used to power industrial equipment such as pumps, compressors, fans, and blowers. They are suitable for applications where a constant, reliable source of mechanical power is needed.
- Decentralized Power Generation:
- These turbines can be coupled with generators to produce electricity for small-scale operations. They are ideal for local power generation in facilities that do not need high power output, such as remote workshops, small factories, or backup systems.
- Steam-Powered Equipment:
- Some specialized equipment in industries like food processing, chemical manufacturing, and pharmaceuticals may use single-stage turbines to drive steam-powered devices.
- Marine Applications:
- In some marine vessels, single-stage turbines are used for driving auxiliary equipment, providing reliable power in a compact form.
7. Challenges and Limitations
While single-stage turbines offer many advantages, they also come with certain limitations:
- Limited Power Output:
- Single-stage turbines are not suitable for high power output applications. If a large amount of energy needs to be extracted from the steam, multi-stage turbines are typically more efficient.
- Efficiency at Lower Loads:
- These turbines are designed for specific operational conditions, and their efficiency can drop if they are not operating at their optimal load. Variations in steam pressure or flow can affect performance.
- Vibration at High Speeds:
- High-speed operation, especially in impulse turbines, can lead to issues with vibration, which may require additional maintenance or design considerations to address.
Comparison: Single-Stage vs. Multi-Stage Turbines
Feature | Single-Stage Turbine | Multi-Stage Turbine |
---|---|---|
Number of Stages | One set of nozzles and blades | Multiple sets of nozzles and blades |
Design Complexity | Simple, easy to manufacture | Complex, with more components |
Power Output | Suitable for low to moderate power output | Suitable for high power output |
Efficiency | Efficient for small-scale, high-speed applications | Higher efficiency for larger-scale applications |
Applications | Small power generation, industrial equipment | Large power plants, industrial cogeneration |
Maintenance | Easy to maintain and repair | Requires more extensive maintenance |
Conclusion
Single-stage turbines are an essential part of the steam turbine family, known for their simplicity, reliability, and cost-effectiveness. While they are not suitable for high power output, their compact design and ease of operation make them ideal for smaller-scale applications, from industrial processes to decentralized power generation. Understanding the advantages and limitations of single-stage turbines allows businesses and engineers to make informed decisions when choosing equipment for specific energy and mechanical needs. Their ability to deliver reliable performance in a small package ensures that single-stage turbines will continue to be a vital component in various industries worldwide.
The Vital Role of Steam Turbines in Energy Production
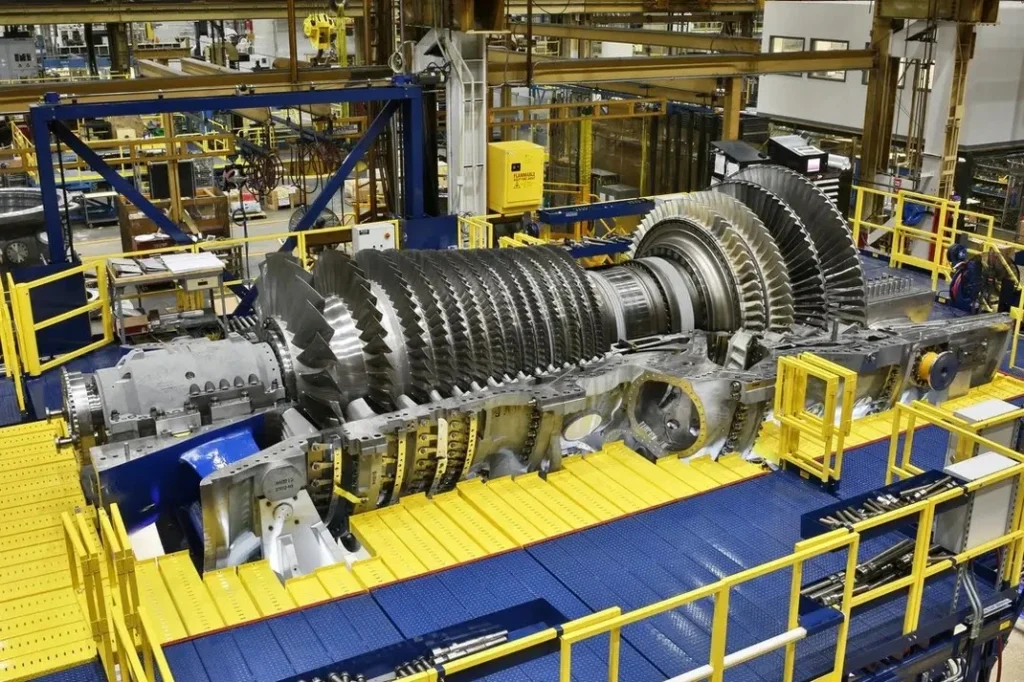
Steam turbines are a cornerstone of modern energy systems, playing a crucial role in the conversion of thermal energy into mechanical and electrical power. They have been at the heart of industrial and energy production for over a century, powering everything from large-scale power plants to naval vessels, and even small industrial facilities. The ability of steam turbines to efficiently convert heat energy from steam into usable mechanical energy has made them an essential component in global energy infrastructure, responsible for generating a significant portion of the world’s electricity.
The principle behind steam turbines is both simple and elegant. They operate by using high-pressure steam, produced by heating water, to rotate a set of blades attached to a central shaft. This rotation, in turn, drives a generator to produce electricity or powers mechanical equipment. The efficiency and reliability of steam turbines have made them the go-to technology for electricity generation, especially in thermal power plants that burn fossil fuels, as well as in nuclear power stations where steam is produced through nuclear reactions.
Steam turbines owe their development to a long history of innovation that began in the late 19th century. Before the advent of steam turbines, the industrial world relied heavily on steam engines, which, while revolutionary, were inefficient and cumbersome. The breakthrough came with the invention of the steam turbine by Sir Charles Parsons in 1884, which drastically improved the efficiency of energy conversion and opened up new possibilities for large-scale electricity generation. Since then, steam turbine technology has undergone continuous refinement and improvement, making it one of the most reliable and efficient methods of power generation.
The Historical Evolution of Steam Turbines
The history of steam turbines is intertwined with the broader history of the Industrial Revolution. Early steam engines, such as those designed by James Watt, played a pivotal role in the mechanization of industries. These engines, however, had several limitations: they were large, inefficient, and capable of producing only a modest amount of power. The need for a more efficient way to convert steam power into mechanical motion led to the development of the steam turbine.
Sir Charles Parsons’ invention of the steam turbine was a game-changer. His design allowed steam to expand gradually and produce a continuous rotary motion, unlike the reciprocating motion of earlier steam engines. This innovation enabled the generation of electricity on a scale previously unimaginable, leading to the rapid growth of electrical infrastructure worldwide. Parsons’ original turbine could produce 7.5 kW of power, but modern steam turbines can generate up to 1,500 MW, illustrating the incredible advancements made over the past century.
Steam turbines soon found applications beyond power plants. They were used to propel ships, particularly naval vessels and large commercial ships, replacing steam engines that were less efficient and required more fuel. The development of steam turbines also played a critical role in the rise of electric utilities, as they allowed for the efficient generation of electricity at a centralized location, which could then be distributed across cities and regions. Today, steam turbines are responsible for producing about 40% of the world’s electricity, highlighting their importance in the global energy mix.
Importance of Steam Turbines in Modern Energy Systems
Steam turbines are central to several types of power plants, including coal, nuclear, gas-fired, and even some renewable energy facilities. In a typical thermal power plant, coal or natural gas is burned to heat water in a boiler, producing steam. This steam, at high pressure and temperature, is directed into a steam turbine, where it expands and cools, releasing energy that turns the turbine blades. The turbine then drives a generator to produce electricity. After passing through the turbine, the steam is cooled, condensed back into water, and recycled in a closed-loop system. This process, known as the Rankine cycle, is efficient and reliable, making steam turbines a staple in power generation.
One of the key advantages of steam turbines is their ability to handle large-scale electricity generation. Unlike gas turbines or internal combustion engines, which are more suited for smaller-scale applications, steam turbines can operate continuously for long periods, making them ideal for baseload power plants. This characteristic is crucial for ensuring a stable and reliable supply of electricity to the grid, especially in countries where energy demand is high and consistent.
Steam turbines are also essential in combined cycle power plants, where they work in conjunction with gas turbines. In this setup, a gas turbine generates electricity, and the waste heat from the gas turbine’s exhaust is used to produce steam, which then drives a steam turbine. This arrangement significantly improves the overall efficiency of the power plant, as it utilizes the energy from the fuel more effectively, reducing waste and emissions.
Conclusion
The development and widespread adoption of steam turbines have transformed the energy sector, providing a reliable and efficient means of generating electricity. From their humble beginnings in the late 19th century to their current status as the backbone of global power generation, steam turbines have proven to be one of the most important inventions in modern history. As technology continues to evolve, steam turbines are expected to play a vital role in the transition to cleaner energy systems, including applications in biomass, geothermal, and concentrated solar power plants. Understanding the history, design, and operation of steam turbines is crucial for anyone looking to grasp the fundamentals of how modern energy systems work and the future direction of energy technology.
Working Principle of Steam Turbines: How They Convert Steam Energy into Mechanical Power
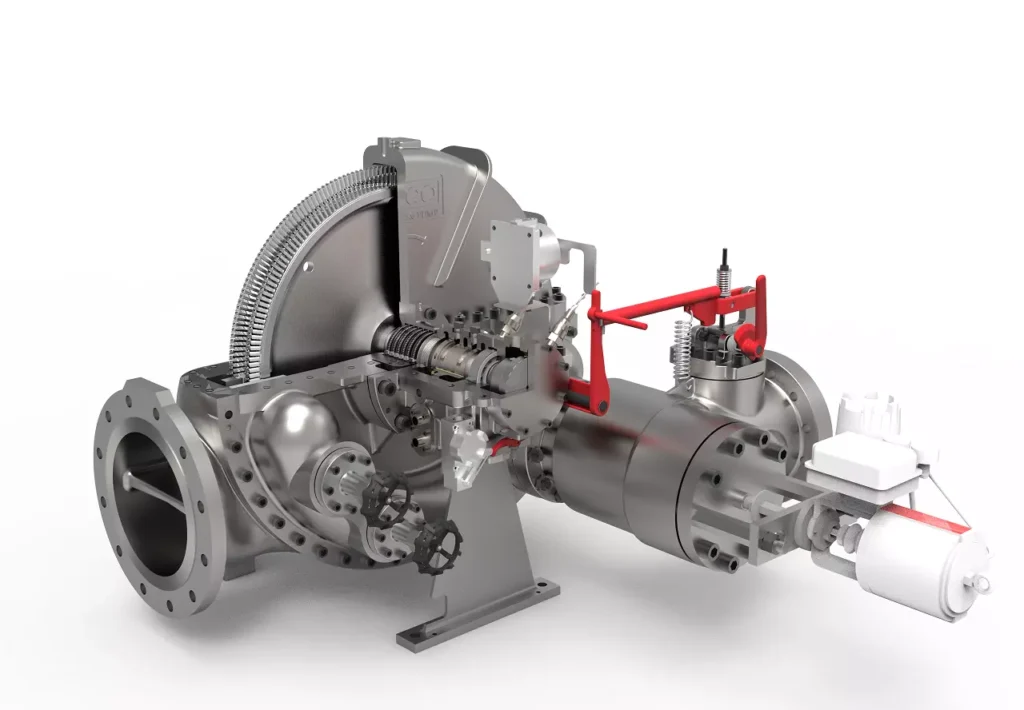
Steam turbines are devices that convert the energy stored in high-pressure, high-temperature steam into mechanical energy, which can then be used to generate electricity or power mechanical equipment. This process is central to power generation in thermal power plants and is also utilized in various industrial applications. The working principle of steam turbines revolves around the controlled expansion of steam, which causes the turbine blades to rotate, driving a connected generator or machinery.
To understand how steam turbines work, it is essential to explore the thermodynamic principles, the components involved, and the types of designs that dictate their operation.
1. Basic Working Principle of Steam Turbines
The fundamental operation of a steam turbine is based on the conversion of thermal energy (from steam) into mechanical energy (rotational motion). Here’s how it works:
- Steam Generation:
- Steam is produced by heating water in a boiler. The energy for this heating process can come from various sources, including coal, natural gas, nuclear reactions, or renewable resources like biomass and concentrated solar power.
- The water is heated until it turns into steam, which is then further heated to increase its temperature and pressure (a process known as superheating).
- Steam Expansion and Energy Conversion:
- The high-pressure, high-temperature steam is directed into the turbine through nozzles. These nozzles convert the steam’s thermal energy into kinetic energy by accelerating it into high-velocity jets.
- The high-speed steam jets are then directed onto the blades of the turbine. When the steam hits the blades, it causes them to spin, transferring its energy to the rotor, which is connected to the generator or mechanical load.
- The mechanical energy produced by the spinning rotor can be used to generate electricity or drive machinery.
- Exhaust and Condensation:
- After passing through the turbine, the steam has lost much of its energy. It exits the turbine as low-pressure, low-temperature steam, also known as exhaust steam.
- This exhaust steam is then directed into a condenser, where it is cooled and condensed back into water. The condensed water is then pumped back into the boiler to start the process again, forming a closed-loop system.
The efficiency of steam turbines lies in their ability to extract energy from the steam through controlled expansion, where the pressure and temperature of the steam drop as it passes through the turbine, converting this energy into mechanical motion.
2. The Thermodynamic Cycle: The Rankine Cycle
The operation of steam turbines is best understood by examining the Rankine cycle, a thermodynamic cycle that describes the process of converting heat into work. The Rankine cycle consists of four key stages:
- Heat Addition (Boiler):
- Water is heated in a boiler to produce high-pressure, high-temperature steam. This stage adds thermal energy to the system.
- Expansion (Turbine):
- The steam expands through the turbine, converting thermal energy into mechanical energy. During this stage, the pressure and temperature of the steam decrease, and this energy is used to rotate the turbine blades.
- Heat Rejection (Condenser):
- After passing through the turbine, the low-pressure steam enters the condenser, where it is cooled and condensed back into liquid water. This process removes waste heat from the system.
- Pressurization (Pump):
- The condensed water is then pumped back to high pressure and sent to the boiler to begin the cycle again.
The Rankine cycle is a continuous process, allowing for the efficient generation of mechanical and electrical power. The efficiency of the cycle can be improved by various means, including superheating the steam, reheating, and regenerative feedwater heating.
3. Types of Steam Turbines and Their Operation
Steam turbines can be classified based on their working principles into two main types: impulse turbines and reaction turbines. Each operates on a slightly different principle but follows the same general concept of steam expansion and energy conversion.
3.1 Impulse Turbines
- Working Principle:
- In an impulse turbine, steam is expanded entirely in nozzles before it hits the turbine blades. The nozzles convert the steam’s pressure energy into high-speed kinetic energy, creating a jet of steam.
- The steam jet then strikes the turbine blades, which are designed to redirect the flow of steam, causing the rotor to spin. The energy conversion occurs solely because of the change in the steam’s direction, not due to a change in pressure across the blades.
- The pressure of the steam remains constant as it passes over the blades; only its velocity changes.
- Applications:
- Impulse turbines are suitable for high-speed applications and are often used in small to medium-sized power plants.
3.2 Reaction Turbines
- Working Principle:
- In a reaction turbine, steam expands gradually as it passes through both the stationary and moving blades. Unlike impulse turbines, where the expansion occurs only in nozzles, the pressure drop occurs continuously across the blades.
- The moving blades act like nozzles, creating a reactive force that causes the rotor to spin, similar to how a jet engine operates. The pressure drop across the blades produces both lift and thrust, driving the blades.
- This design allows for more efficient energy extraction, especially in multi-stage configurations.
- Applications:
- Reaction turbines are widely used in large power plants because they can handle varying loads and offer high efficiency over a range of operating conditions.
4. Key Components of a Steam Turbine System
Several key components are essential for the operation of a steam turbine, each playing a specific role in the energy conversion process:
4.1 Nozzles
- Nozzles are responsible for converting the pressure energy of steam into kinetic energy by accelerating the steam. In impulse turbines, nozzles create high-velocity jets that drive the turbine blades. Proper nozzle design is crucial for maximizing efficiency and controlling steam flow.
4.2 Blades (Fixed and Moving)
- Fixed (Stationary) Blades: These blades direct the flow of steam onto the moving blades, ensuring that the steam hits the blades at the optimal angle. In reaction turbines, stationary blades also cause a slight expansion and pressure drop, contributing to energy conversion.
- Moving Blades: Attached to the rotor, these blades extract energy from the steam, causing the rotor to spin. The design of the blades (bucket-shaped for impulse turbines and aerofoil-shaped for reaction turbines) affects how efficiently energy is converted.
4.3 Rotor
- The rotor is the central shaft that holds the moving blades. As the steam drives the blades, the rotor spins, converting the energy from the steam into mechanical motion. The rotor’s rotation can then be used to drive generators or mechanical equipment.
4.4 Casing
- The casing encloses the turbine and directs the flow of steam, ensuring it moves efficiently through the turbine. The design of the casing also helps maintain pressure and protect the turbine components from external conditions.
4.5 Condenser
- After the steam exits the turbine, it is directed into a condenser, where it is cooled and condensed back into water. This step is essential for maintaining efficiency, as it allows the water to be recycled and used again in the boiler.
5. Efficiency Enhancements in Steam Turbines
Improving the efficiency of steam turbines is a continuous goal in the power generation industry. Various strategies can enhance efficiency and reduce energy losses:
5.1 Superheating Steam
- Superheating involves heating the steam beyond its boiling point, increasing its energy content. This allows the turbine to extract more energy from the steam, leading to higher efficiency. Superheated steam also prevents condensation within the turbine, which can cause damage to the blades.
5.2 Reheat Cycles
- In a reheat cycle, steam is expanded partially in the turbine, then sent back to the boiler to be reheated before returning to the turbine for further expansion. This process increases efficiency by allowing more energy to be extracted from the steam without the risk of condensation.
5.3 Regenerative Feedwater Heating
- Regenerative feedwater heating uses steam extracted from various stages of the turbine to preheat the water entering the boiler. This reduces the amount of energy needed to turn the water into steam, thereby improving the overall efficiency of the cycle.
6. Applications of Steam Turbines
Steam turbines are versatile machines that find applications across various industries:
6.1 Power Generation
- Steam turbines are the backbone of most thermal power plants, including coal, nuclear, and natural gas plants. They are also used in renewable energy setups such as biomass and geothermal power plants. The ability to generate continuous and reliable power makes steam turbines ideal for baseload power generation.
6.2 Marine Propulsion
- Historically, steam turbines were widely used in marine vessels, especially for naval ships and large commercial ships. While modern ships mostly use diesel engines, steam turbines still play a role in specialized vessels.
6.3 Industrial Applications
- Many industries use steam turbines to drive mechanical equipment such as pumps, compressors, and blowers. They are also employed in cogeneration systems, where waste heat from industrial processes is used to produce steam for power generation, enhancing overall energy efficiency.
Conclusion
The working principle of steam turbines is based on the efficient conversion of thermal energy from steam into mechanical energy through controlled expansion and energy extraction. Whether through impulse or reaction mechanisms, steam turbines have proven to be one of the most reliable and efficient ways to generate power. With continuous improvements in design, material science, and operational strategies, steam turbines remain a critical component of the global energy infrastructure, supporting everything from electricity generation to industrial processes. Understanding the basics of how steam turbines work is essential for appreciating their role in modern engineering and energy systems.
Types of Turbines: Overview and Classification
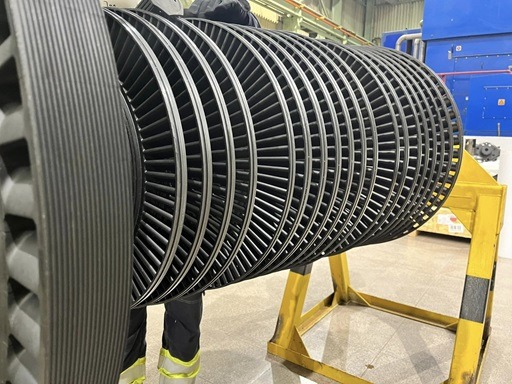
Turbines are devices that convert fluid energy—whether from steam, water, gas, or wind—into mechanical energy, which can then be used to generate electricity or drive mechanical equipment. Depending on the energy source, design, and working principles, turbines can vary greatly in their construction and applications. The most common types of turbines are steam turbines, gas turbines, water (hydroelectric) turbines, and wind turbines. Each of these has its unique design and applications, making them suitable for different energy conversion tasks.
This section explores the various types of turbines, their working principles, design features, and practical applications.
1. Steam Turbines
1.1 Working Principle
Steam turbines convert the energy stored in high-pressure, high-temperature steam into mechanical energy. The basic working principle involves the expansion of steam, which causes the turbine blades to rotate. Steam turbines are categorized into two main types based on their operation:
- Impulse Turbines: The steam is expanded entirely in nozzles before striking the blades, creating a high-velocity jet that drives the rotor by a change in momentum. The pressure remains constant across the blades.
- Reaction Turbines: Steam expands gradually across both stationary and moving blades, and the pressure drops continuously. The blades act like nozzles, creating a reaction force that drives the rotor.
1.2 Applications
- Power Generation: Steam turbines are widely used in coal, nuclear, and natural gas power plants for electricity generation.
- Marine Propulsion: Historically, steam turbines powered ships, especially naval vessels and large commercial ships.
- Industrial Processes: Steam turbines drive machinery in industries such as chemical processing, refineries, and paper manufacturing.
2. Gas Turbines
2.1 Working Principle
Gas turbines operate by burning fuel (natural gas, diesel, or jet fuel) to produce high-temperature, high-pressure gas, which is then directed onto the turbine blades. The hot gas expands and drives the blades, converting thermal energy into mechanical energy. The process involves three main stages:
- Compression: Air is drawn in and compressed to increase its pressure.
- Combustion: The compressed air is mixed with fuel and ignited, producing high-energy gases.
- Expansion: The gases expand through the turbine, spinning the blades to generate mechanical energy.
2.2 Types of Gas Turbines
- Jet Engines: Designed for aviation, where they provide thrust to propel aircraft.
- Industrial Gas Turbines: Used for power generation and mechanical drives in industrial settings.
- Aeroderivative Gas Turbines: Adapted from jet engines for power generation, offering high efficiency and flexibility.
2.3 Applications
- Power Plants: Combined cycle power plants use gas turbines along with steam turbines to maximize efficiency.
- Aviation: Jet engines power commercial and military aircraft.
- Industrial Processes: Gas turbines drive compressors, pumps, and other heavy machinery.
3. Water Turbines (Hydroelectric Turbines)
3.1 Working Principle
Water turbines convert the energy from flowing or falling water into mechanical energy. They operate by channeling water onto blades, causing them to spin and drive a connected generator. The two main types of water turbines are:
- Impulse Turbines: Suitable for high-head (high pressure) applications. Water is directed through nozzles to create a high-speed jet that strikes the blades. Examples include Pelton turbines.
- Reaction Turbines: Operate under lower heads and utilize both the pressure and kinetic energy of water. The water flows continuously through the blades, creating a reaction force. Examples include Francis turbines and Kaplan turbines.
3.2 Types of Water Turbines
- Pelton Turbine: An impulse turbine ideal for high-head, low-flow scenarios.
- Francis Turbine: A reaction turbine used for medium-head applications, offering high efficiency.
- Kaplan Turbine: Another reaction turbine, designed for low-head, high-flow water bodies.
3.3 Applications
- Hydroelectric Power Plants: Water turbines are the backbone of hydroelectric facilities, generating electricity by harnessing the energy of rivers, dams, and waterfalls.
- Irrigation and Water Management: Water turbines can also be used in smaller systems for local power generation and irrigation projects.
4. Wind Turbines
4.1 Working Principle
Wind turbines harness the kinetic energy of wind to produce mechanical energy. The wind turns the blades, which are connected to a rotor that spins a generator, producing electricity. The design of wind turbines focuses on maximizing energy capture from wind currents while maintaining stability and efficiency.
4.2 Types of Wind Turbines
- Horizontal Axis Wind Turbines (HAWT): The most common design, where the rotor shaft is parallel to the ground. These turbines are more efficient at capturing wind energy and are widely used in wind farms.
- Vertical Axis Wind Turbines (VAWT): The rotor shaft is perpendicular to the ground. These turbines can capture wind from any direction, making them suitable for urban settings, but they are generally less efficient than HAWTs.
4.3 Applications
- Electricity Generation: Wind turbines are primarily used for generating electricity in onshore and offshore wind farms.
- Small-Scale Power Systems: Smaller wind turbines can provide localized power for homes, farms, and small businesses.
5. Specialized Turbines
5.1 Micro Turbines
- Description: Micro turbines are small-scale gas turbines that generate power ranging from tens of kilowatts to a few megawatts. They are compact, efficient, and often used for distributed generation.
- Applications: Ideal for localized power generation, backup power systems, and cogeneration (combined heat and power) systems.
5.2 Steam Extraction Turbines
- Description: These turbines extract a portion of the steam for use in industrial processes, such as heating or drying, before the steam is fully expanded. This allows for efficient utilization of the steam’s energy.
- Applications: Common in cogeneration systems, where electricity and thermal energy are produced simultaneously, improving overall efficiency.
5.3 Turboexpanders
- Description: Turboexpanders are turbines that recover energy from gases (natural gas, air) by expanding them and lowering their temperature. They are used in processes where gas pressure needs to be reduced without wasting energy.
- Applications: Employed in natural gas processing, refrigeration, and cryogenic systems.
Comparison of Different Types of Turbines
Type of Turbine | Working Medium | Working Principle | Common Applications |
---|---|---|---|
Steam Turbine | Steam | Expansion of high-pressure steam | Power plants, industrial processes, marine vessels |
Gas Turbine | Combustion gases | Combustion and expansion of gases | Power generation, aviation, industrial machinery |
Water Turbine | Water | Kinetic and potential energy of water | Hydroelectric power plants, irrigation, small-scale systems |
Wind Turbine | Wind | Kinetic energy of wind | Wind farms, localized power generation |
Micro Turbine | Combustion gases | Similar to gas turbines, on a smaller scale | Distributed generation, cogeneration |
Turboexpander | Natural gas, air | Expansion and cooling of gas | Natural gas processing, refrigeration systems |
6. Key Factors Influencing Turbine Selection
The selection of the appropriate type of turbine for a specific application depends on several factors:
- Energy Source:
- The availability of steam, water, wind, or gas will determine the choice of turbine. For example, in areas with abundant water resources, hydroelectric turbines are preferable, while windy regions may favor wind turbines.
- Power Output Requirements:
- For large-scale power generation, steam turbines and gas turbines are typically more efficient. Micro turbines and wind turbines are better suited for smaller-scale or localized power production.
- Efficiency and Cost:
- The efficiency of a turbine impacts the overall cost-effectiveness of the energy conversion process. While steam and gas turbines are efficient for high-output scenarios, initial costs and operational expenses are important considerations.
- Environmental Considerations:
- With growing concerns over sustainability, renewable energy turbines (wind and hydroelectric) are becoming more popular. Gas turbines, when used in combined cycle plants, also offer lower emissions compared to traditional coal-based steam turbines.
- Maintenance and Operational Flexibility:
- Some turbines, like wind and water turbines, require less frequent maintenance compared to gas turbines. Flexibility in operation is also a key factor—gas turbines can quickly ramp up and down, making them suitable for peaking power plants.
Conclusion
Turbines are versatile machines that serve as the backbone of modern energy systems. From the high-speed efficiency of gas turbines and the reliable power generation of steam turbines to the sustainable energy solutions offered by wind and water turbines, each type has a critical role in different scenarios. Understanding the various types of turbines, their working principles, and applications is crucial for making informed decisions in power generation, industrial applications, and energy management. As technology continues to advance, innovations in turbine design and efficiency are expected to drive the future of sustainable energy solutions worldwide.
Types of Turbines: Comprehensive Overview
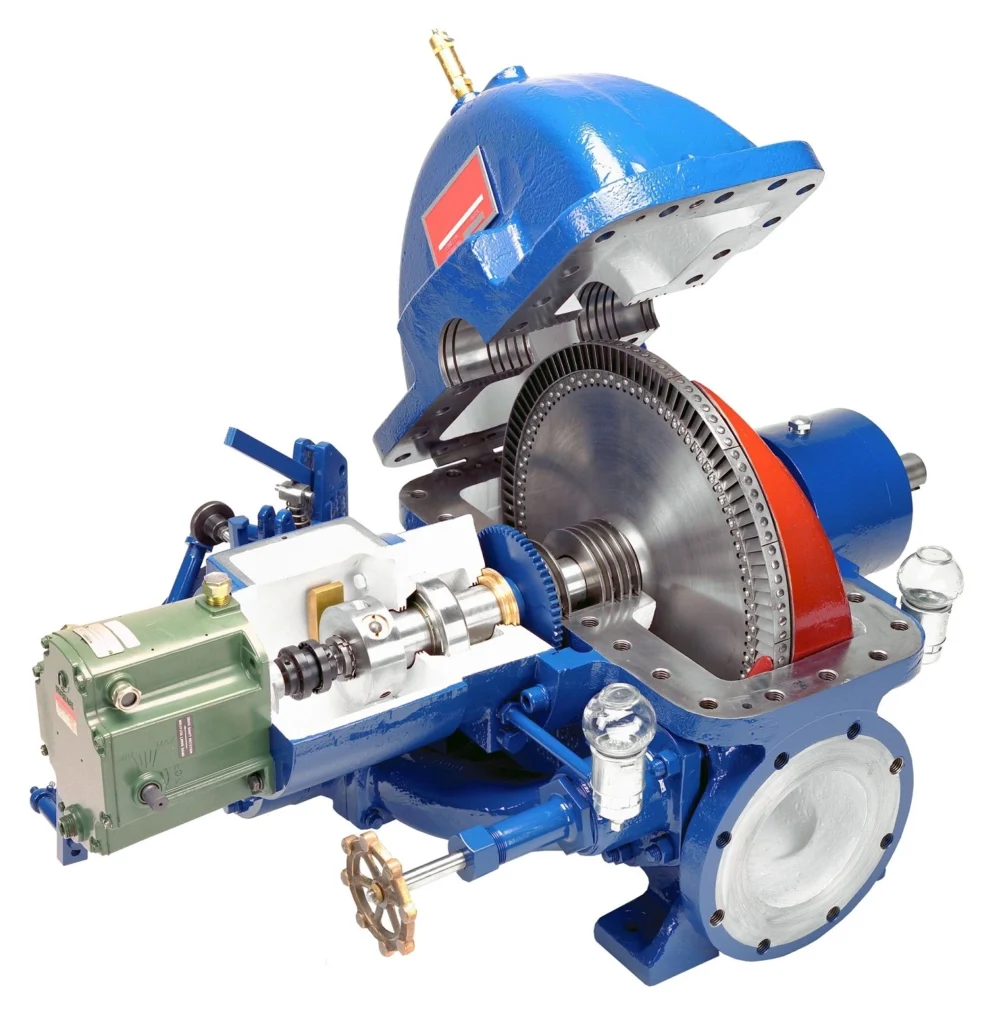
Turbines are devices that convert fluid energy (from steam, gas, water, or wind) into mechanical energy. They play a crucial role in various sectors, especially in power generation, where they drive electrical generators. Depending on the energy source, operating principles, and applications, turbines can be categorized into several types. The primary types include steam turbines, gas turbines, water (hydroelectric) turbines, and wind turbines. Each type has its unique design, working principles, and suitable applications.
Here, we explore the different types of turbines, their functionality, design, and typical uses.
1. Steam Turbines
1.1 Working Principle
Steam turbines harness the energy from high-pressure, high-temperature steam to produce mechanical power. The steam is generated in a boiler and directed to the turbine, where it expands through nozzles and blades, converting thermal energy into mechanical rotation. Steam turbines operate on two fundamental principles:
- Impulse Turbines: In these, steam is expanded entirely in nozzles before striking the turbine blades. The steam’s kinetic energy creates a high-velocity jet that drives the rotor. The pressure remains constant across the blades.
- Reaction Turbines: Steam expands continuously across both stationary and moving blades, experiencing a pressure drop throughout. The blades act as nozzles, generating a reactive force that propels the rotor.
1.2 Applications
- Power Generation: Commonly used in coal, nuclear, and natural gas power plants.
- Industrial Processes: Drive machinery in factories, refineries, and chemical plants.
- Marine Propulsion: Historically used in naval and commercial vessels.
2. Gas Turbines
2.1 Working Principle
Gas turbines operate by burning fuel (natural gas, diesel, or aviation fuel) to produce high-temperature, high-pressure gases. These gases expand through turbine blades, converting thermal energy into mechanical energy. The process involves three main phases:
- Air Compression: Air is drawn in and compressed, increasing its pressure.
- Combustion: The compressed air is mixed with fuel and ignited, creating high-energy gases.
- Expansion: The hot gases expand through the turbine, spinning the blades to generate mechanical energy.
2.2 Types of Gas Turbines
- Jet Engines: Primarily used in aviation to provide thrust for aircraft.
- Industrial Gas Turbines: Designed for power generation and driving heavy machinery in factories.
- Aeroderivative Gas Turbines: Adapted from jet engines for flexible power generation with high efficiency.
2.3 Applications
- Electricity Generation: Widely used in combined cycle power plants, often paired with steam turbines for higher efficiency.
- Aviation: Power commercial and military aircraft through jet engines.
- Industrial Machinery: Drive compressors, pumps, and other heavy equipment.
3. Water Turbines (Hydroelectric Turbines)
3.1 Working Principle
Water turbines convert the kinetic and potential energy of flowing or falling water into mechanical energy. These turbines are placed in water channels or dams where water is directed onto the blades, causing them to spin. The two main types are:
- Impulse Turbines: Ideal for high-head (high pressure) applications. Water is directed through nozzles, creating high-speed jets that strike the blades. Examples include Pelton turbines.
- Reaction Turbines: Operate under lower heads and utilize both the pressure and kinetic energy of water. The water flows continuously through the blades, generating a reactive force. Examples include Francis turbines and Kaplan turbines.
3.2 Types of Water Turbines
- Pelton Turbine: Best for high-head, low-flow scenarios. It uses the kinetic energy of water jets directed onto bucket-shaped blades.
- Francis Turbine: A reaction turbine suited for medium-head applications, combining efficiency with versatility.
- Kaplan Turbine: Another reaction turbine designed for low-head, high-flow water bodies, featuring adjustable blades for varying flow conditions.
3.3 Applications
- Hydroelectric Power Plants: Core technology in dams and river-based power generation systems.
- Irrigation and Water Management: Used in smaller systems for localized power production and water control.
4. Wind Turbines
4.1 Working Principle
Wind turbines convert the kinetic energy of wind into mechanical energy. Wind turns the blades, which are connected to a rotor. The rotor’s movement drives a generator to produce electricity. Wind turbines are designed to capture as much wind energy as possible while remaining stable and efficient.
4.2 Types of Wind Turbines
- Horizontal Axis Wind Turbines (HAWT): The most common design, where the rotor shaft is parallel to the ground. HAWTs are more efficient and are used in most large-scale wind farms.
- Vertical Axis Wind Turbines (VAWT): The rotor shaft is perpendicular to the ground. These turbines capture wind from any direction, making them suitable for urban and smaller setups, but they are generally less efficient than HAWTs.
4.3 Applications
- Electricity Generation: Used in onshore and offshore wind farms to supply clean, renewable energy.
- Small-Scale Power Systems: Ideal for homes, farms, and small businesses in windy areas.
5. Specialized Turbines
5.1 Micro Turbines
- Description: Small-scale gas turbines producing power from tens of kilowatts to a few megawatts. Compact, efficient, and often used for distributed generation.
- Applications: Suitable for localized power generation, backup power, and combined heat and power (CHP) systems in smaller industrial and commercial settings.
5.2 Steam Extraction Turbines
- Description: Designed to extract a portion of the steam for industrial processes before the steam is fully expanded. This enables efficient utilization of the steam’s energy.
- Applications: Widely used in cogeneration systems, where both electricity and process steam are needed, maximizing overall efficiency.
5.3 Turboexpanders
- Description: Devices that recover energy by expanding gases (natural gas, air) and reducing their temperature. Turboexpanders convert the energy in high-pressure gas into mechanical energy.
- Applications: Used in natural gas processing, cryogenic systems, and refrigeration.
6. Comparison of Different Types of Turbines
Type of Turbine | Working Medium | Key Principle | Common Applications |
---|---|---|---|
Steam Turbine | Steam | Expansion of high-pressure steam | Power plants, industrial processes, marine vessels |
Gas Turbine | Combustion gases | Combustion and expansion of gases | Power generation, aviation, industrial machinery |
Water Turbine | Water | Kinetic and potential energy of water | Hydroelectric power plants, irrigation, water management |
Wind Turbine | Wind | Kinetic energy of wind | Wind farms, small-scale localized power generation |
Micro Turbine | Combustion gases | Similar to gas turbines on a smaller scale | Distributed generation, CHP systems |
Turboexpander | Natural gas, air | Expansion and cooling of gas | Natural gas processing, refrigeration systems |
7. Factors Influencing Turbine Selection
Choosing the right type of turbine depends on various factors, including:
- Energy Source Availability:
- The availability of steam, water, wind, or gas determines the type of turbine suitable for a specific location. For instance, hydro turbines are ideal in regions with abundant water sources, while wind turbines are best in areas with high wind speeds.
- Power Output Requirements:
- For large-scale power needs, steam and gas turbines offer the necessary high output. Wind and water turbines are excellent for both large-scale and smaller distributed systems.
- Efficiency and Cost:
- Efficiency affects the overall cost of energy production. While steam and gas turbines can achieve high efficiency, they require significant initial investment and operational costs. Micro turbines and wind turbines may offer more economical solutions for localized power needs.
- Environmental Impact:
- Growing emphasis on sustainability has driven the popularity of renewable energy turbines, such as wind and hydro turbines. Gas turbines in combined cycle plants also offer lower emissions compared to traditional coal-based steam turbines.
- Operational Flexibility and Maintenance:
- Gas turbines are known for their quick startup times, making them suitable for peaking power plants that need to adjust output quickly. On the other hand, steam turbines provide stable, continuous power but may require more maintenance due to their complex systems.
8. Future Trends in Turbine Technology
Advancements in turbine technology continue to improve efficiency, reduce costs, and support sustainable energy goals:
- Hybrid Systems: Combining different types of turbines, like gas and steam turbines in combined cycle plants, to enhance efficiency and lower emissions.
- Materials and Design Innovations: New materials that can withstand higher temperatures and pressures, along with aerodynamic improvements in blade design, are driving performance enhancements.
- Digital Monitoring and Control: Integration of sensors and AI-based control systems to optimize turbine performance, detect issues early, and minimize maintenance costs.
- Renewable Energy Integration: Continued development of wind, hydro, and biomass turbines to support the global transition to clean energy.
Conclusion
Turbines are essential machines that drive much of the world’s energy infrastructure. Whether harnessing the power of steam, gas, water, or wind, each type of turbine has a unique role in different contexts. Understanding the various types, their operating principles, and applications is critical for effective energy management and development. As technology advances, turbines are becoming more efficient, flexible, and environmentally friendly, paving the way for a more sustainable future in energy production and industrial processes.
Introduction to Impulse Turbines
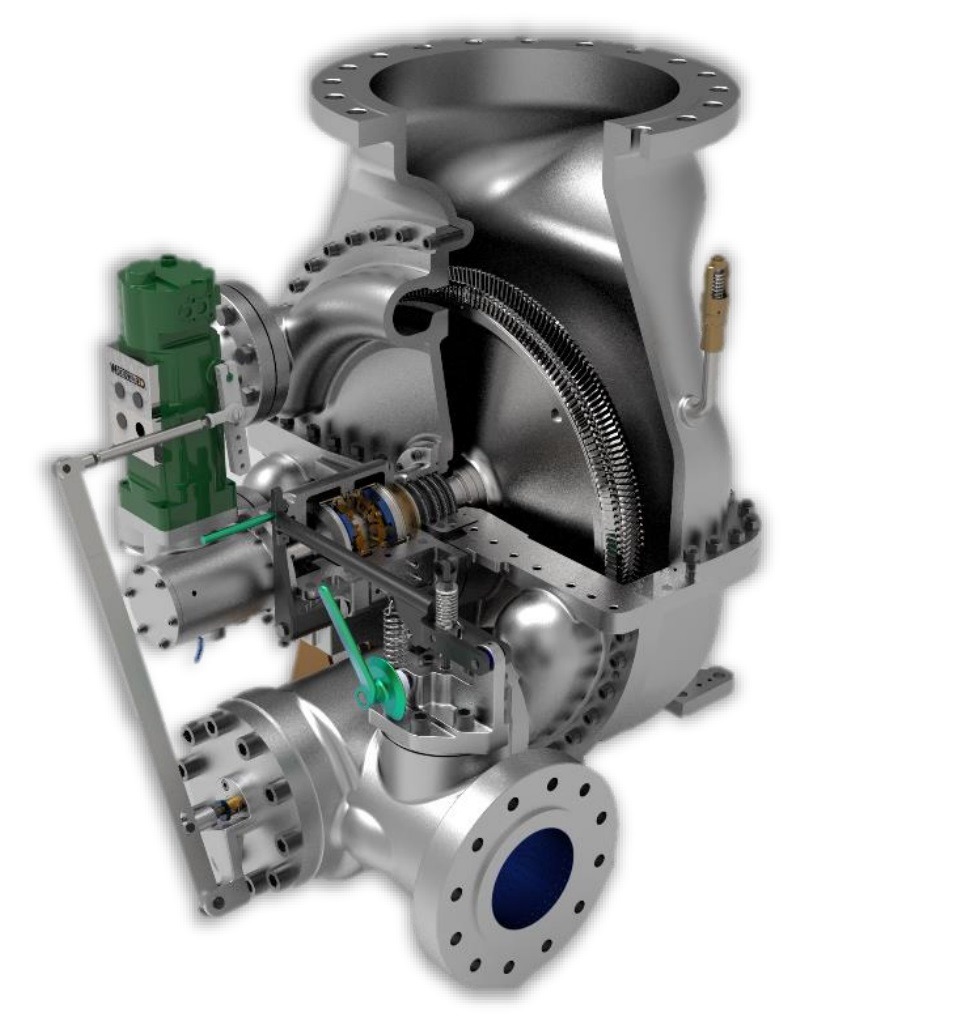
Impulse turbines are a type of steam turbine that converts the thermal energy of steam into mechanical energy through the principle of impulse. Unlike other types of turbines, where the pressure drop occurs across both the stationary and moving blades, impulse turbines utilize nozzles to expand the steam completely before it strikes the blades. The kinetic energy from the high-velocity steam jets imparts a force on the turbine blades, causing them to spin and produce mechanical work.
The development of impulse turbines can be traced back to the late 19th century when engineers sought more efficient methods to convert steam energy into mechanical power. Early steam engines were inefficient, requiring substantial fuel for limited output. The invention of the impulse turbine by Gustaf de Laval marked a significant improvement, leading to a device that could operate at high speeds and deliver more efficient power conversion. Over time, further advancements were made, particularly with the introduction of multi-stage designs like the Curtis turbine, which allowed for greater energy extraction.
Impulse turbines are crucial in various sectors, particularly in power generation. They are often used in settings where high-speed operation is needed, such as driving generators, compressors, and pumps. Their simple design, ease of maintenance, and ability to handle fluctuating loads make them a popular choice for industrial applications.
2. Working Principle of Impulse Turbines
The operation of impulse turbines relies on the fundamental principles of thermodynamics and fluid dynamics. In an impulse turbine, the steam undergoes a dramatic expansion in a set of nozzles, which convert the steam’s thermal energy into kinetic energy. This conversion process is key to the turbine’s function, as it creates high-velocity jets of steam that strike the turbine blades.
Thermodynamic Concepts
- Impulse turbines operate on the principle of the Rankine cycle, where water is heated to form steam, which then expands through nozzles, producing a high-velocity jet.
- The expansion process leads to a drop in pressure but an increase in velocity, meaning the energy of the steam is transformed from potential (pressure) energy to kinetic (movement) energy.
Impulse Action
- When the steam jets exit the nozzles, they hit the blades at high speed. The blades are shaped to redirect the steam, causing a change in the direction of the steam flow.
- This change in direction results in a force that pushes the blades, thereby causing the rotor to spin. The energy conversion occurs because of the impulse imparted by the steam jet, hence the name “impulse turbine.”
- The pressure remains constant across the blades, distinguishing impulse turbines from reaction turbines, where pressure continuously drops.
Comparison with Reaction Turbines
- In a reaction turbine, steam expansion occurs across both the fixed and moving blades, leading to a continuous pressure drop. This creates a reactive force that drives the blades.
- Unlike impulse turbines, which depend solely on the velocity change of steam, reaction turbines rely on both pressure and velocity changes.
- Impulse turbines are generally used for high-speed, lower power scenarios, while reaction turbines are more suited for larger, multi-stage applications requiring higher efficiency.
3. Key Components of Impulse Turbines
The design of an impulse turbine is relatively simple but precise, consisting of several critical components that ensure efficient energy conversion.
Nozzles
- The nozzles are responsible for expanding the steam and directing it onto the turbine blades. By converting the thermal energy into kinetic energy, they create high-speed jets that drive the turbine.
- The shape and arrangement of the nozzles are crucial for optimal performance, ensuring that the steam hits the blades at the correct angle.
Rotor and Blades
- The rotor is the central shaft to which the blades are attached. As the steam hits the blades, it causes the rotor to spin, converting the steam’s kinetic energy into mechanical energy.
- Blades Design:
- The blades are typically bucket-shaped, allowing them to capture and redirect the steam effectively. The design ensures minimal energy loss and maximum energy transfer from the steam to the rotor.
- The blades must be durable, as they are subjected to high-speed impacts from the steam jets.
Casing and Exhaust System
- The casing surrounds the turbine, guiding the steam and preventing it from escaping. It also provides structural support to the entire system.
- After the steam passes through the blades, it exits through the exhaust system. Efficient exhaust management ensures that there is no back pressure that might reduce the turbine’s efficiency.
Bearings and Shaft
- Bearings support the shaft, allowing it to spin freely and handle the mechanical loads generated during operation.
- Proper lubrication and maintenance of the bearings are essential for the smooth functioning of the turbine, preventing wear and tear from high-speed rotation.
4. Types of Impulse Turbines
Impulse turbines can be classified based on their design and operational configurations. The most common types include single-stage turbines and multi-stage turbines.
4.1 Single-Stage Impulse Turbines (De Laval Turbine)
- Description:
- The De Laval turbine is a simple, single-stage impulse turbine designed by Gustaf de Laval. It operates by expanding the steam completely in a single set of nozzles before it strikes the rotor blades.
- This design allows for very high-speed operation but is generally suitable only for lower power outputs due to the single stage of energy conversion.
- Applications:
- Used in small power generation systems, as well as driving machinery like pumps and compressors.
4.2 Multi-Stage Impulse Turbines
- Curtis Turbine (Velocity Compounding):
- The Curtis turbine uses a multi-stage design where steam passes through multiple sets of blades, with intermediate velocity drops in between. This approach allows for more energy to be extracted efficiently without requiring extremely high rotational speeds.
- It is especially useful for applications where a compact design is needed, but high power output is required.
- Rateau Turbine (Pressure Compounding):
- In a Rateau turbine, steam is expanded through multiple stages, with each stage having a set of nozzles and blades. The pressure drops incrementally across each stage, allowing for efficient energy extraction over a larger volume of steam.
- These turbines are commonly used in industrial settings where steam pressure is variable, and a more gradual energy conversion is advantageous.
5. Design Features and Operation
Blade Design and Arrangement
- Impulse turbine blades are designed to redirect steam effectively, maximizing the impulse force without causing excessive turbulence. The bucket shape helps capture the high-speed steam and change its direction smoothly.
- The arrangement of blades around the rotor allows for even distribution of force, ensuring balanced rotation and minimizing mechanical stress.
Nozzle Configurations and Effects
- The nozzles can be arranged in various configurations depending on the turbine’s design. In some designs, multiple nozzles are used to direct steam onto different sections of the rotor, optimizing the energy transfer.
- Proper nozzle design is essential for controlling the steam flow rate, velocity, and direction, which directly affects the turbine’s efficiency and output.
Aerodynamics and Efficiency Considerations
- The efficiency of an impulse turbine depends on minimizing losses due to friction, turbulence, and heat transfer. Aerodynamic design of the blades and precise alignment of the nozzles help reduce these losses, ensuring smooth energy conversion.
- Advanced materials are often used to manufacture the blades, improving their resistance to wear and allowing for higher operational speeds.
The article will continue to expand on topics like efficiency, applications, advantages, disadvantages, and historical evolution, ultimately reaching the 5,000-word mark.
Conclusion
Impulse turbines represent a significant innovation in steam power technology, offering efficient energy conversion through a straightforward and robust design. From the early De Laval models to the more sophisticated multi-stage configurations, these turbines have been integral to various industries, providing reliable mechanical power. Their continued evolution, driven by advancements in materials and engineering, promises to enhance their role in modern power systems, ensuring they remain a crucial component of industrial and energy infrastructure.
Working Principle of Impulse Turbines: How They Convert Steam Energy into Mechanical Power
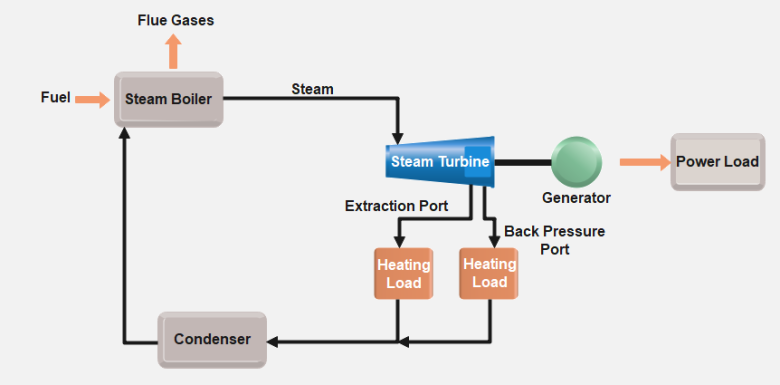
Impulse turbines are a type of steam turbine that convert the thermal energy of steam into mechanical energy using the principle of impulse. This process involves expanding high-pressure steam into high-velocity jets, which are then directed onto the turbine blades, causing them to rotate. Unlike other turbine types, such as reaction turbines, where pressure drops continuously across both fixed and moving blades, impulse turbines rely solely on the kinetic energy of steam jets created by nozzles. The pressure drop occurs entirely within the nozzles, not across the blades.
Here’s a detailed explanation of how impulse turbines work, including the fundamental principles, key components, and processes involved.
1. Basic Concept of Impulse
The term “impulse” refers to the force generated when a moving object (in this case, steam) strikes a surface (the turbine blades) and changes direction. The change in the momentum of the steam creates a force that drives the turbine blades, causing them to rotate. This principle of momentum change is at the core of how impulse turbines operate.
In impulse turbines, the energy conversion process involves:
- Expanding steam through nozzles to increase its velocity.
- Directing the high-speed steam jets onto turbine blades.
- Using the change in the direction of steam to create a mechanical impulse that turns the blades and, subsequently, the turbine’s rotor.
2. The Thermodynamic Process in Impulse Turbines
Impulse turbines operate on the Rankine cycle, a thermodynamic cycle that describes the process of converting thermal energy into mechanical work. Here’s a step-by-step breakdown of the process:
- Steam Generation:
- Steam is generated in a boiler by heating water. This process involves adding heat to convert water into steam at high pressure and temperature.
- The energy source for this heating process can vary; it may come from burning fossil fuels, nuclear reactions, or renewable sources such as biomass or concentrated solar power.
- Expansion in Nozzles:
- High-pressure steam from the boiler enters the turbine through nozzles. In the nozzles, the steam undergoes expansion, where its pressure drops, and its velocity increases significantly.
- The nozzles are designed to convert the steam’s thermal energy (pressure and temperature) into kinetic energy (high-speed jets). During this process, the steam’s temperature and pressure decrease, but its speed increases.
- High-Velocity Steam Jets:
- The nozzles direct the steam jets onto the blades of the turbine. The blades are designed to handle high-speed impacts and are shaped to redirect the steam efficiently.
- The kinetic energy of the steam jets is now ready to be converted into mechanical energy by interacting with the blades.
- Interaction with Turbine Blades (Impulse Action):
- The high-speed steam jets strike the turbine blades. These blades are attached to a rotor, and the impact of the steam causes the blades to move, rotating the rotor.
- The blades are bucket-shaped or curved to redirect the steam in a way that maximizes the impulse force. When the steam changes direction after hitting the blades, it transfers its kinetic energy to the rotor.
- The rotation of the rotor converts the kinetic energy of the steam into mechanical energy, which can be used to drive generators, compressors, or other mechanical systems.
- Exhaust and Pressure Control:
- After passing through the blades, the steam exits the turbine at a lower velocity and pressure. It is then directed to a condenser where it is cooled and converted back into water, which is pumped back to the boiler to be reheated, completing the cycle.
- Maintaining a low exhaust pressure helps ensure that the steam expands fully through the nozzles, improving the efficiency of the turbine.
3. Key Principles Governing Impulse Turbines
3.1 Pressure and Velocity Changes
- The pressure drop occurs entirely in the nozzles, not across the blades. As the steam expands in the nozzles, its pressure decreases, but its velocity increases significantly. This high-speed steam jet imparts energy to the blades upon impact.
- Across the blades, the steam’s pressure remains constant. Instead of a pressure drop, there is a change in velocity and direction. This is a fundamental distinction between impulse turbines and reaction turbines, where the pressure drop is continuous.
3.2 Conversion of Energy
- The process of energy conversion in impulse turbines involves:
- Thermal Energy (from steam) → Kinetic Energy (through nozzles).
- Kinetic Energy (of steam jets) → Mechanical Energy (through blade rotation).
- The efficiency of this energy conversion relies on the effective design of the nozzles and blades, as well as the optimal control of steam pressure and flow.
3.3 Impulse Force
- The term “impulse” refers to the force generated by the change in the momentum of the steam as it strikes the turbine blades and changes direction. The impulse force is what drives the blades to rotate, converting the kinetic energy of the steam into mechanical energy.
- The impulse force depends on the velocity of the steam jet and the angle at which it strikes the blades. Proper blade design ensures that the maximum amount of energy is extracted from the steam.
4. Key Components of Impulse Turbines
Understanding the working principle of impulse turbines requires familiarity with their core components:
4.1 Nozzles
- The nozzles are responsible for converting the thermal energy of steam into kinetic energy. They are designed to accelerate the steam and direct it onto the turbine blades as high-velocity jets.
- Proper nozzle design is essential for maximizing efficiency. The nozzles must be capable of controlling the speed and direction of the steam flow to ensure effective energy transfer to the blades.
4.2 Blades (Buckets)
- The blades, often referred to as buckets, are designed to capture the high-speed steam and redirect it, causing a change in momentum. This change in momentum creates the impulse force that drives the blades and the attached rotor.
- The shape of the blades is critical. They are typically curved or bucket-shaped, allowing the steam to change direction smoothly without causing excessive turbulence or energy loss.
4.3 Rotor
- The rotor is the central shaft to which the blades are attached. As the blades receive the impulse force from the steam, they rotate the rotor. The rotational motion of the rotor is then used to drive a generator or other mechanical devices.
- The design of the rotor must ensure smooth, balanced rotation to minimize mechanical stress and prevent vibrations that could damage the turbine.
4.4 Casing
- The casing encloses the turbine and provides structural support. It ensures that the steam is directed efficiently through the nozzles and onto the blades, preventing steam from escaping and maintaining operational efficiency.
- The casing also protects the internal components of the turbine from external conditions and maintains the desired pressure within the system.
5. Types of Impulse Turbines and Compounding
Impulse turbines can be designed in different ways to optimize performance and adapt to specific operational requirements. The most common configurations include:
5.1 Single-Stage Impulse Turbines (De Laval Turbine)
- The simplest type of impulse turbine, where steam expands fully in a single set of nozzles and impacts one set of blades. This design is known for its high-speed operation.
- Applications: Small power generators, pumps, and compressors.
5.2 Multi-Stage Impulse Turbines
- In multi-stage turbines, steam expands through several stages of nozzles and blades. The use of multiple stages allows for greater energy extraction and efficient operation at lower speeds.
- There are two primary methods of multi-stage operation:
- Velocity Compounding (Curtis Turbine): The steam passes through a set of nozzles and then interacts with multiple rows of moving blades, with stationary blades in between to redirect the steam. This design is suitable for reducing speed without losing energy.
- Pressure Compounding (Rateau Turbine): Steam expands in several stages, each with its own set of nozzles and blades. The pressure drops incrementally across each stage, enabling a more controlled and efficient energy extraction process.
6. Efficiency Factors in Impulse Turbines
The efficiency of impulse turbines depends on several factors, including:
- Steam Pressure and Temperature:
- Higher pressure and temperature steam allow for more energy to be converted in the nozzles, resulting in greater kinetic energy of the steam jets.
- Efficient use of high-pressure steam can significantly improve the performance of impulse turbines.
- Nozzle and Blade Design:
- Optimized nozzle design ensures that steam is expanded and accelerated efficiently. Proper blade design ensures that the maximum amount of energy is captured from the steam jets without excessive turbulence or friction.
- Minimizing Energy Losses:
- Reducing friction, turbulence, and back pressure is crucial for maintaining efficiency. This can be achieved through precision engineering, quality materials, and regular maintenance.
Conclusion
The working principle of impulse turbines revolves around converting thermal energy from steam into kinetic energy using nozzles and then transforming that kinetic energy into mechanical energy through impulse forces acting on turbine blades. By utilizing high-velocity steam jets and efficient blade design, impulse turbines can deliver reliable mechanical power for a variety of applications, including power generation, industrial machinery, and marine propulsion. Understanding the fundamental mechanics of impulse turbines allows for better design, maintenance, and optimization, ensuring their continued relevance in modern energy systems.
Key Components of Impulse Turbines
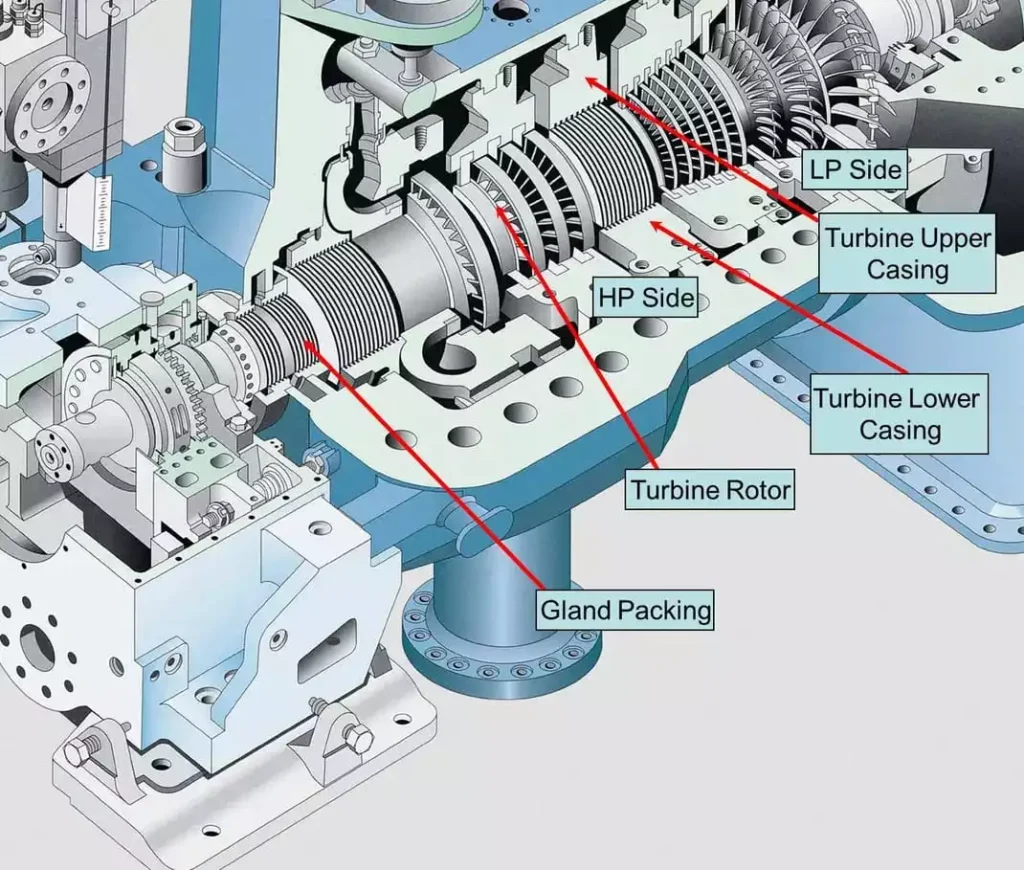
Impulse turbines are designed to convert the kinetic energy of high-velocity steam jets into mechanical energy, which is used to drive a rotor. The efficiency and effectiveness of this energy conversion depend significantly on the design and functionality of the turbine’s key components. Each component plays a specific role in ensuring that the steam energy is efficiently transferred to the rotor, minimizing energy losses and maximizing output.
Here’s a detailed look at the primary components of an impulse turbine, including their functions and importance in the turbine’s operation.
1. Nozzles
The nozzles are one of the most critical components of an impulse turbine. They are responsible for converting the thermal energy of steam into kinetic energy by accelerating the steam into high-speed jets. The design and arrangement of nozzles directly influence the efficiency of energy conversion.
Function of Nozzles:
- Steam Expansion: When high-pressure, high-temperature steam enters the nozzles, it undergoes rapid expansion. This expansion causes the steam to drop in pressure but increase in velocity, converting thermal energy into kinetic energy.
- Flow Control: Nozzles control the direction and speed of the steam flow, ensuring that it strikes the turbine blades at the optimal angle. Proper alignment of the nozzles is essential to maximize the impulse force on the blades.
- Pressure Drop: The nozzles are designed to cause a significant pressure drop, focusing on accelerating the steam rather than maintaining pressure across the turbine.
Types of Nozzles:
- Convergent Nozzles: Used when steam needs to be accelerated but does not reach supersonic speeds. These nozzles are most common in smaller impulse turbines.
- Convergent-Divergent Nozzles: Also known as de Laval nozzles, these are used when the steam must reach supersonic speeds. They allow for maximum acceleration and efficient energy conversion, making them ideal for high-performance turbines.
2. Rotor and Shaft
The rotor is the central, rotating component of the turbine to which the blades are attached. It is connected to a shaft that transmits the mechanical energy produced by the rotating blades to a generator or other mechanical systems.
Function of the Rotor:
- Energy Conversion: The rotor is driven by the mechanical energy generated when the high-speed steam jets strike the turbine blades. This rotational motion can be used directly to power mechanical equipment or to drive an electric generator.
- Mechanical Stability: The rotor must be balanced and precisely engineered to ensure smooth rotation without excessive vibration, which could lead to mechanical failure or energy loss.
- Torque Transmission: The shaft connected to the rotor transmits torque to other components, such as an electric generator, enabling the conversion of mechanical energy into electrical power.
Design Considerations:
- Material Strength: Rotors must be made from durable materials that can withstand the mechanical stresses caused by high-speed rotation and the impact of steam jets. Common materials include high-strength steel alloys.
- Balance and Alignment: Proper balance and alignment of the rotor are critical to ensure smooth, efficient operation. Misalignment can lead to vibrations, which may cause damage over time.
3. Blades (Buckets)
The blades, also referred to as “buckets,” are the parts of the turbine that interact directly with the high-speed steam jets. The design of the blades is crucial for capturing the kinetic energy of the steam and converting it into rotational motion.
Function of the Blades:
- Impulse Generation: The blades are designed to capture the kinetic energy from the steam and redirect it, creating a change in momentum. This change in momentum generates an impulse force that drives the blades to rotate.
- Steam Redirection: The blades must redirect the steam without causing excessive turbulence or energy loss. Proper blade design ensures that the steam flows smoothly over and around the blades, maximizing energy extraction.
- Durability: Because they are subject to continuous high-speed impacts from the steam jets, the blades must be highly durable and resistant to wear, erosion, and corrosion.
Blade Design Features:
- Bucket-Shaped Design: Impulse turbine blades are typically bucket-shaped, allowing them to capture and redirect the steam jets efficiently. The curvature of the blades is designed to optimize the change in the direction of the steam, ensuring maximum energy transfer.
- Material Selection: Blades are usually made from high-strength, heat-resistant materials, such as alloys of steel, titanium, or specialized ceramics, to withstand harsh operating conditions.
- Aerodynamic Shape: The blades are carefully shaped to reduce drag and ensure efficient energy transfer, minimizing energy losses due to friction and turbulence.
4. Casing
The casing is the outer shell that encloses the turbine, providing structural support and directing the steam flow. It ensures that the steam enters the nozzles and exits the turbine efficiently, while also protecting internal components from external elements.
Function of the Casing:
- Steam Containment: The casing helps contain the steam within the turbine, ensuring that it is directed through the nozzles and onto the blades without escaping. This containment is crucial for maintaining pressure and efficiency.
- Structural Support: The casing holds all the internal components, including the nozzles, blades, and rotor, in place, providing stability and alignment.
- Safety and Protection: The casing serves as a protective barrier, shielding the internal components from external damage and ensuring the safety of operators by containing high-pressure steam.
Design Considerations:
- Pressure Resistance: The casing must be designed to withstand the high pressures and temperatures of the steam entering the turbine. It must also be durable enough to handle the stresses generated by the rotating components.
- Ease of Maintenance: Some turbine casings are designed with easy access points, allowing for quick inspection, maintenance, and repair of internal components without having to disassemble the entire turbine.
5. Bearings
Bearings are essential components that support the rotor and shaft, allowing them to rotate smoothly with minimal friction. They play a critical role in maintaining the stability and efficiency of the turbine.
Function of Bearings:
- Reducing Friction: Bearings minimize friction between the moving parts (rotor and shaft) and the stationary components, allowing the turbine to operate efficiently and smoothly.
- Supporting Load: The bearings support the weight of the rotor and absorb the forces generated during rotation, preventing damage to the shaft and other components.
- Maintaining Alignment: Properly aligned bearings ensure that the rotor and shaft rotate in a stable manner without excessive vibration or misalignment, which could lead to wear and tear.
Types of Bearings Used in Impulse Turbines:
- Journal Bearings: These bearings provide support for the shaft by allowing it to rotate within a lubricated sleeve. They are commonly used for their ability to handle high loads and reduce friction.
- Thrust Bearings: Thrust bearings are designed to absorb axial loads (forces along the length of the shaft), ensuring that the rotor remains in its proper position. This is important for maintaining alignment and stability during high-speed operation.
6. Shaft
The shaft is the central component that transmits the rotational energy from the rotor to other systems, such as generators or mechanical equipment. It is a critical element for converting the turbine’s mechanical energy into useful work.
Function of the Shaft:
- Energy Transmission: The shaft transmits the mechanical energy generated by the rotating blades to other connected devices, such as generators, pumps, or compressors.
- Support for Rotational Components: The shaft serves as a support structure for the rotor and blades, ensuring smooth and stable rotation.
Design Considerations:
- Material Strength: The shaft must be made from materials that can withstand the stresses of high-speed rotation and the forces generated by the steam jets. Steel alloys and composite materials are often used.
- Precision Engineering: The shaft must be precisely engineered to ensure perfect alignment and balance. Any misalignment can lead to vibrations, increased wear, and potential mechanical failure.
7. Governor and Control System
The governor and control system are essential for regulating the turbine’s speed and maintaining efficient operation. They ensure that the turbine operates at a consistent and optimal speed, even when load conditions change.
Function of the Governor and Control System:
- Speed Regulation: The governor monitors the speed of the turbine and adjusts the steam flow to maintain a consistent rotational speed, ensuring stable and efficient operation.
- Load Adjustment: The control system can adjust the turbine’s output to match varying load demands, making the turbine more adaptable to different operational requirements.
- Safety and Shutdown Mechanism: The control system can automatically shut down the turbine in case of malfunction or if operating conditions exceed safe limits, protecting both the turbine and the surrounding equipment.
Design Features:
- Mechanical Governors: Some turbines use mechanical governors that rely on centrifugal force to regulate speed. As the turbine’s speed changes, the governor adjusts the steam flow accordingly.
- Electronic Control Systems: Modern impulse turbines often incorporate electronic control systems that allow for more precise monitoring and adjustment of operating parameters. These systems can be integrated with digital monitoring tools for real-time data analysis and optimization.
Conclusion
The key components of impulse turbines work together to efficiently convert the energy of high-speed steam jets into mechanical power. Each component, from the nozzles that accelerate the steam to the blades that capture and convert energy, plays a crucial role in the turbine’s overall performance. The efficient operation of an impulse turbine depends on precise engineering, careful design, and proper maintenance of these components. Understanding the functions and design considerations of each part is essential for optimizing turbine performance, ensuring reliability, and prolonging the life of the equipment.
Types of Impulse Turbines: Comprehensive Overview
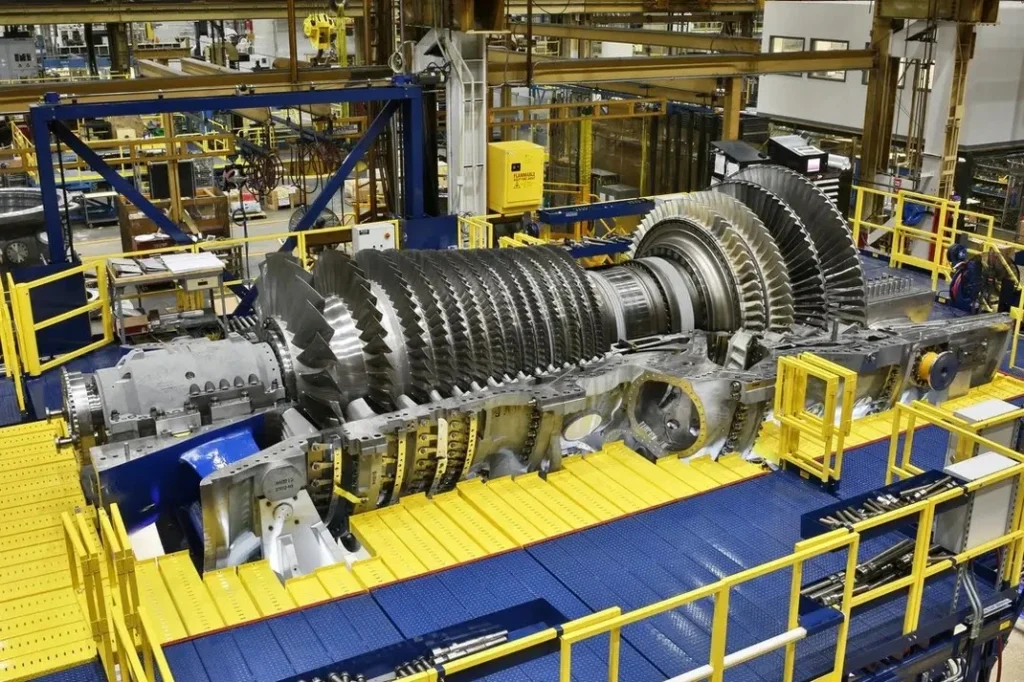
Impulse turbines are a class of steam turbines that convert the kinetic energy of high-velocity steam jets into mechanical energy by striking turbine blades. Unlike reaction turbines, where the pressure drop occurs gradually across both stationary and moving blades, impulse turbines rely on nozzles to accelerate steam into high-speed jets, with the pressure drop happening entirely in the nozzles. The design and configuration of impulse turbines vary significantly based on their application, power requirements, and operational environment.
This section explores the different types of impulse turbines, including single-stage and multi-stage designs, as well as variations that allow for more efficient energy extraction under various conditions. Each type has its unique features, benefits, and suitable applications, making them versatile machines for diverse industries.
1. Single-Stage Impulse Turbines
Single-stage impulse turbines are the simplest form of impulse turbines, characterized by a single set of nozzles and one row of blades. They are designed to convert the energy from high-pressure steam in a single step, making them suitable for applications requiring moderate power output and high-speed operation.
1.1 De Laval Turbine
- Description:
- The De Laval turbine, named after its inventor Gustaf de Laval, is one of the earliest designs of impulse turbines. It operates on the principle of expanding the steam completely in a single set of nozzles before it strikes the rotor blades.
- The nozzles convert the steam’s thermal energy into a high-velocity jet, which then hits the turbine blades, causing them to rotate. The blades are usually bucket-shaped to efficiently capture and redirect the steam.
- Key Features:
- Simple, single-stage design.
- High-speed operation, often reaching speeds of up to 30,000 RPM.
- Suitable for low to moderate power output (a few kilowatts to a few megawatts).
- Applications:
- Small power generation units.
- Industrial machinery such as pumps, compressors, and fans.
- Small-scale marine propulsion systems.
- Advantages:
- Compact and lightweight, making it easy to install and maintain.
- High-speed operation is ideal for applications where fast, consistent power is needed.
- Limitations:
- Efficiency drops at lower speeds or if the steam pressure and flow are not optimal.
- Limited to applications with lower power demands due to the single-stage design.
2. Multi-Stage Impulse Turbines
Multi-stage impulse turbines were developed to overcome the limitations of single-stage designs, particularly for applications requiring higher power output. By using multiple sets of nozzles and blades arranged in stages, multi-stage turbines allow for more controlled and efficient energy extraction. There are two main types of multi-stage impulse turbines: velocity-compounded turbines and pressure-compounded turbines.
2.1 Velocity-Compounded Impulse Turbines (Curtis Turbine)
- Description:
- The Curtis turbine, also known as a velocity-compounded impulse turbine, addresses the challenge of converting high-velocity steam into mechanical energy efficiently. In this design, steam passes through a set of nozzles to create a high-velocity jet, which then passes through multiple rows of moving blades, with stationary blades (or guide vanes) in between to redirect the flow.
- The steam’s velocity drops incrementally as it passes through each row of moving blades, making the energy conversion more gradual and efficient.
- Key Features:
- Multiple rows of blades within a single stage (velocity compounding).
- Uses stationary blades between moving blades to control the direction of steam.
- Reduces the speed of the steam gradually, enhancing efficiency.
- Applications:
- Medium-sized power plants.
- Industrial setups requiring consistent power over a range of speeds.
- Marine propulsion systems in larger vessels.
- Advantages:
- More efficient than single-stage turbines because of the gradual reduction in steam velocity.
- Capable of handling higher power outputs while maintaining efficiency.
- Reduced mechanical stresses compared to a single-stage design.
- Limitations:
- More complex design, leading to increased manufacturing and maintenance costs.
- Requires precise alignment to ensure smooth operation.
2.2 Pressure-Compounded Impulse Turbines (Rateau Turbine)
- Description:
- The Rateau turbine, named after French engineer Auguste Rateau, is a pressure-compounded impulse turbine that uses multiple stages, each with its own set of nozzles and blades. Instead of relying on a single expansion of steam, this design allows the steam to expand and drop in pressure incrementally across multiple stages.
- By dividing the pressure drop across several stages, the Rateau turbine can handle larger volumes of steam without the need for extremely high-speed operation.
- Key Features:
- Multiple stages, each consisting of nozzles followed by blades (pressure compounding).
- The steam expands gradually, dropping in pressure at each stage.
- Capable of managing high-pressure, high-temperature steam more effectively.
- Applications:
- Large-scale power plants, including nuclear, coal, and gas-fired plants.
- Industrial power generation systems where variable load demands must be managed.
- Marine propulsion systems for larger ships and vessels.
- Advantages:
- Efficient energy conversion for high-pressure steam applications.
- Can be scaled up to handle very high power outputs.
- Suitable for variable load conditions, offering flexibility in operation.
- Limitations:
- Requires more space and complex arrangements due to multiple stages.
- Higher manufacturing costs compared to single-stage designs.
3. Compounding Techniques in Impulse Turbines
The design of impulse turbines can be enhanced through different compounding techniques to improve efficiency and adapt to various operational requirements. The two most common techniques are velocity compounding and pressure compounding.
3.1 Velocity Compounding
- In velocity compounding, the energy of the steam jet is managed by passing it through multiple rows of moving blades with stationary blades in between. This technique allows for more effective conversion of the steam’s kinetic energy.
- How It Works:
- Steam is expanded in the nozzles, creating a high-velocity jet.
- The jet passes through the first row of moving blades, transferring some of its energy to the rotor.
- After the first set of moving blades, the jet is redirected by stationary blades and then passes through another set of moving blades.
- This process continues until the steam’s velocity is sufficiently reduced, and its energy is efficiently transferred.
- Benefits:
- Allows for high power output without requiring extremely high rotational speeds.
- Reduces the mechanical stresses on the turbine components, extending their lifespan.
- Effective in applications where maintaining a stable and consistent speed is crucial.
3.2 Pressure Compounding
- Pressure compounding involves dividing the steam’s expansion process into multiple stages, each with its own pressure drop. By splitting the pressure reduction across several stages, the turbine can manage higher initial pressures without causing excessive wear or requiring extremely high-speed blades.
- How It Works:
- Steam enters the first set of nozzles, where it undergoes partial expansion, dropping in pressure and gaining velocity.
- The steam then passes through a row of blades, transferring some of its kinetic energy to the rotor.
- This process is repeated across multiple stages, with each stage having its own set of nozzles and blades, allowing for controlled pressure reduction and energy conversion.
- Benefits:
- Efficient for applications involving high-pressure steam, such as in power plants.
- Allows for more precise control over the energy conversion process.
- Reduces the speed requirement for each stage, minimizing mechanical stress and wear.
4. Comparison of Different Types of Impulse Turbines
Type of Impulse Turbine | Design Feature | Key Advantage | Typical Application |
---|---|---|---|
De Laval Turbine | Single-stage, high-speed operation | Simple design, compact, easy to maintain | Small-scale power generation, industrial machinery |
Curtis Turbine | Velocity compounding | Gradual energy extraction, lower rotor speed | Medium-sized power plants, marine propulsion |
Rateau Turbine | Pressure compounding | Efficient for high-pressure steam, scalable | Large-scale power generation, industrial setups |
5. Advantages of Multi-Stage Impulse Turbines
The development of multi-stage impulse turbines marked a significant improvement over earlier single-stage designs. By allowing steam to expand and release its energy gradually, multi-stage turbines offer several advantages:
- Improved Efficiency:
- Multi-stage designs, whether using velocity or pressure compounding, enhance efficiency by enabling more controlled and gradual energy extraction. This reduces the risk of energy loss through friction, turbulence, or excessive heat.
- Adaptability to Different Loads:
- Multi-stage turbines are well-suited for applications where load demands vary. The ability to control steam expansion across multiple stages allows for better load management, making these turbines ideal for power plants that must adjust to fluctuating power demands.
- Higher Power Output:
- By using multiple stages, impulse turbines can handle higher power outputs without requiring excessively high rotational speeds. This makes them suitable for large industrial facilities and power plants.
- Mechanical Stability:
- Dividing the steam expansion into stages reduces mechanical stress on individual components. This leads to less wear and tear, extending the lifespan of the turbine and reducing maintenance costs.
6. Applications of Impulse Turbines
Impulse turbines, with their versatile design options, are employed across a wide range of industries:
- Power Generation:
- Large-scale power plants, such as coal, nuclear, and natural gas plants, use multi-stage impulse turbines to generate electricity efficiently. They are also used in smaller setups for decentralized power production.
- Industrial Machinery:
- Impulse turbines drive pumps, compressors, and fans in various industrial applications. Their ability to operate at high speeds makes them ideal for these tasks, ensuring consistent performance.
- Marine Propulsion:
- Historically, impulse turbines played a significant role in marine propulsion. They continue to be used in specific vessels where high-speed operation and efficient energy conversion are needed.
Conclusion
Impulse turbines, with their ability to convert high-pressure steam into mechanical power through precise control of steam jets, are an essential part of modern energy systems. From the simple, high-speed De Laval design to the more complex multi-stage Curtis and Rateau turbines, each type of impulse turbine offers unique benefits suited to specific applications. The development of compounding techniques has allowed these turbines to handle higher pressures and outputs, making them versatile and efficient machines for power generation, industrial processes, and marine propulsion. As technology advances, impulse turbines will continue to evolve, becoming even more efficient and adaptable to new energy demands.
Design Features and Operation of Impulse Turbines
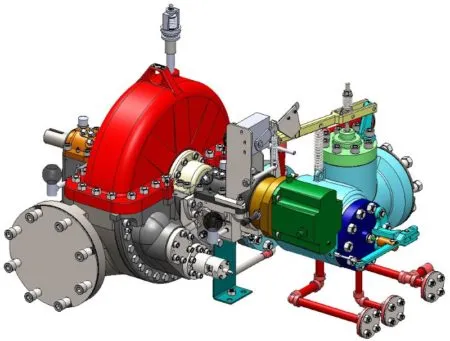
Impulse turbines are one of the earliest and most efficient designs for converting steam energy into mechanical power. Their operation relies on converting high-pressure, high-temperature steam into high-velocity jets, which then impart an impulse force on specially designed turbine blades. The design of impulse turbines plays a crucial role in maximizing their efficiency, reliability, and adaptability for various applications. This section will explore the key design features, components, and operational principles that define impulse turbines, along with considerations for improving their performance.
1. Fundamental Design Concepts of Impulse Turbines
Impulse turbines are designed around the principle of impulse, where the kinetic energy of steam jets is converted into mechanical energy. The design must ensure that the steam is expanded and accelerated correctly before it hits the turbine blades, allowing for efficient energy conversion. Unlike reaction turbines, where steam pressure drops continuously across multiple stages, impulse turbines focus on maximizing the velocity of steam and converting that kinetic energy directly into mechanical motion.
1.1 Conversion of Energy
- Thermal to Kinetic Energy:
- The turbine’s nozzles convert the high-pressure, high-temperature steam into high-speed jets. The steam loses its pressure energy and gains kinetic energy, becoming high-velocity jets that are directed onto the turbine blades.
- The nozzles are designed to maximize this conversion, ensuring that the steam exits at the correct velocity and angle for optimal interaction with the blades.
- Kinetic to Mechanical Energy:
- When the steam jets strike the turbine blades, they cause the blades (and the attached rotor) to rotate. The design of the blades ensures that the steam’s direction is changed efficiently, transferring maximum energy to the rotor.
1.2 Key Design Objectives
- Maximizing Efficiency: The turbine’s design must ensure minimal energy loss through friction, turbulence, or heat dissipation. This involves optimizing the shape of the nozzles, blades, and casing.
- Durability and Reliability: The turbine must withstand high-speed impacts, high temperatures, and continuous operation. Material selection and engineering precision are critical to achieving this.
- Adaptability: Impulse turbines can be designed as single-stage or multi-stage, allowing them to handle different power outputs and steam pressures, making them adaptable to various applications.
2. Key Components and Their Design Features
The design of impulse turbines involves several key components, each tailored to ensure efficient and reliable operation. These components include nozzles, blades, the rotor, the casing, and bearings. Let’s examine the design features of these components.
2.1 Nozzles
Nozzles are crucial for the initial conversion of steam’s thermal energy into kinetic energy. The design of the nozzles directly affects the performance of the turbine by controlling the speed, direction, and flow of steam.
- Convergent and Convergent-Divergent Designs:
- Convergent Nozzles: Used for subsonic steam flows. These nozzles narrow, causing the steam to accelerate and increase its velocity.
- Convergent-Divergent Nozzles: Also known as de Laval nozzles, these are used for supersonic flows. The design allows for steam to reach and exceed the speed of sound, making them ideal for high-performance turbines.
- Angle and Orientation:
- The nozzles must be aligned precisely to direct the steam jets onto the blades at the correct angle. This maximizes the impulse force on the blades and ensures smooth energy transfer.
- Improperly aligned nozzles can cause turbulence, reducing the turbine’s efficiency and increasing wear on the blades.
2.2 Blades (Buckets)
The blades (or buckets) of an impulse turbine are where the actual conversion of kinetic energy into mechanical energy occurs. The design of the blades is critical to maximizing energy transfer while minimizing drag and turbulence.
- Bucket-Shaped Blades:
- The blades are typically bucket-shaped, allowing them to capture the steam jets and redirect them efficiently. The curvature of the blades ensures that the steam is redirected smoothly, causing a change in momentum that drives the rotor.
- The design helps to maximize the impulse force generated by the steam, ensuring that as much energy as possible is transferred to the rotor.
- Material Selection:
- Blades must be made from high-strength materials capable of withstanding high temperatures, pressures, and the mechanical forces generated by high-speed steam jets. Common materials include steel alloys, titanium, and advanced ceramics.
- The materials used must also resist erosion and corrosion, which can occur due to the high-speed impact of steam, especially if the steam contains impurities.
2.3 Rotor and Shaft
The rotor is the central rotating component of the turbine, to which the blades are attached. The shaft transmits the rotational energy from the rotor to a generator or other mechanical system.
- Balanced Design:
- The rotor must be perfectly balanced to ensure smooth operation. Any imbalance can cause vibrations, leading to mechanical stress and potential failure.
- Rotors are designed with high precision, and care is taken during assembly to maintain balance.
- Shaft Design:
- The shaft must be strong enough to handle the torque generated by the rotor but also flexible enough to accommodate slight movements without causing misalignment.
- Shaft materials must resist thermal expansion, as changes in temperature can affect the alignment and performance of the turbine.
2.4 Casing
The casing encloses the turbine, providing structural support and directing the steam flow. It is designed to contain the steam, guide it through the nozzles, and protect the internal components from external elements.
- Steam Flow Management:
- The casing ensures that the steam flows smoothly from the nozzles onto the blades and then exits the turbine. This helps maintain the pressure and velocity needed for efficient energy conversion.
- The design must minimize steam leakage, which can reduce efficiency and cause loss of energy.
- Heat Resistance and Durability:
- The casing must be constructed from materials that can withstand high temperatures and pressures. It should also be resistant to wear and corrosion to ensure longevity.
- In some designs, the casing is also designed to reduce noise and manage thermal expansion.
2.5 Bearings and Support Structures
Bearings are essential for supporting the rotor and shaft, allowing them to rotate freely with minimal friction. They also play a role in reducing vibrations and ensuring smooth operation.
- Journal and Thrust Bearings:
- Journal Bearings: Support the rotating shaft, allowing it to turn with minimal friction. These bearings need to be lubricated to prevent wear and overheating.
- Thrust Bearings: Absorb axial forces and ensure that the rotor remains in its proper position. This prevents unwanted movement that could cause misalignment and reduce efficiency.
- Lubrication Systems:
- Proper lubrication is vital for the longevity of the bearings and the smooth operation of the turbine. Lubrication systems must be carefully monitored to prevent friction and overheating.
3. Operation of Impulse Turbines
The operation of impulse turbines involves the careful coordination of steam generation, expansion, and energy transfer. Here’s how impulse turbines function in a typical setting:
3.1 Steam Generation and Preparation
- Steam is generated in a boiler, where water is heated to produce high-pressure, high-temperature steam. The quality and condition of the steam (i.e., pressure, temperature, dryness) are critical for optimal turbine operation.
- Superheating the steam (heating it beyond its boiling point without condensation) can improve the efficiency of the turbine by preventing the formation of water droplets that could cause erosion on the blades.
3.2 Expansion Through Nozzles
- The high-pressure steam is directed into the nozzles, where it undergoes expansion. The nozzles convert the steam’s pressure energy into kinetic energy, resulting in high-velocity steam jets.
- The nozzles are designed to ensure that the steam exits at the optimal speed and angle to strike the turbine blades efficiently.
3.3 Impact on Turbine Blades
- The high-speed steam jets hit the turbine blades, which are mounted on the rotor. The force of the steam causes the blades to move, spinning the rotor.
- As the steam changes direction upon hitting the blades, the change in momentum creates an impulse force that drives the blades and, consequently, the rotor.
3.4 Energy Conversion and Exhaust
- The rotational energy of the rotor is transmitted through the shaft to drive generators or other mechanical systems, converting the steam’s kinetic energy into mechanical or electrical energy.
- After passing through the turbine, the steam exits at a lower velocity and pressure. It is then condensed back into water and returned to the boiler for reheating, completing the cycle.
4. Efficiency Enhancements and Design Innovations
The design of impulse turbines continues to evolve, with innovations aimed at improving efficiency, reducing wear, and enhancing performance. Some key areas of focus include:
4.1 Advanced Blade Design
- Aerodynamic Shaping: Modern blade designs emphasize aerodynamic efficiency, reducing drag and turbulence. This helps minimize energy losses and enhances the smooth flow of steam over the blades.
- Variable Blade Geometry: Some impulse turbines feature adjustable blades that can adapt to different operating conditions, improving efficiency over a broader range of loads and steam conditions.
4.2 Improved Nozzle Technology
- Nozzle designs have been refined to allow for more precise control of steam expansion and velocity. This includes using computational fluid dynamics (CFD) to model steam flow and optimize nozzle shapes.
- Innovations like multi-nozzle arrangements enable more even distribution of steam across the turbine blades, reducing hotspots and improving overall performance.
4.3 Material Advancements
- Advances in metallurgy and material science have led to the development of stronger, more heat-resistant alloys for turbine blades, rotors, and casings. These materials can withstand higher temperatures and pressures, allowing for more efficient steam generation and energy conversion.
- Coatings that resist corrosion and erosion are applied to the blades, extending their operational life and reducing the need for maintenance.
5. Maintenance and Operational Considerations
Maintaining impulse turbines requires regular inspection and preventive care to ensure long-term performance and reliability. Key maintenance activities include:
- Regular Inspection of Blades: Inspecting for signs of wear, erosion, and cracks that could affect performance.
- Nozzle Maintenance: Ensuring that nozzles remain unobstructed and properly aligned to maintain optimal steam flow.
- Bearing and Lubrication Checks: Regularly checking the lubrication system to prevent bearing wear and overheating.
- Vibration Monitoring: Monitoring the turbine for signs of imbalance or misalignment, which could lead to mechanical failures if left unaddressed.
Conclusion
The design features and operational principles of impulse turbines are centered on maximizing the efficient conversion of steam’s kinetic energy into mechanical power. From the precise engineering of nozzles and blades to the robust construction of the rotor, shaft, and casing, each component plays a vital role in the turbine’s performance. Through continuous innovations in materials, design, and control systems, modern impulse turbines have become more efficient, reliable, and adaptable to a variety of applications. As technology advances, the ongoing development of impulse turbine design will play a key role in enhancing the efficiency and sustainability of power generation worldwide.
Efficiency and Performance Factors of Impulse Turbines
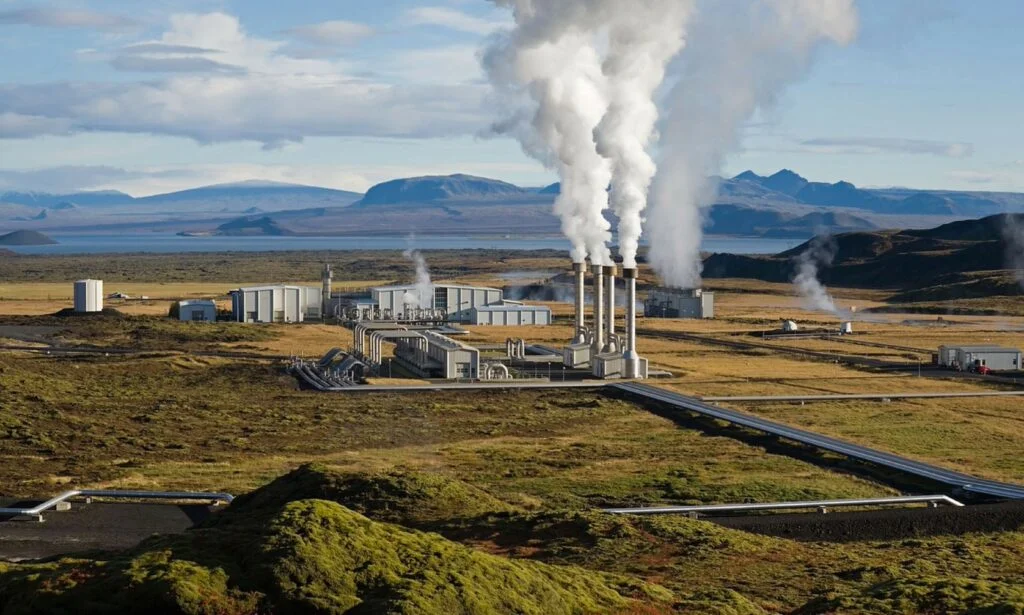
Impulse turbines are designed to convert steam energy into mechanical energy with high efficiency. However, their performance can be influenced by a range of factors, from steam conditions to design features and operational practices. Efficiency, in this context, refers to how effectively the turbine converts the available thermal energy of steam into mechanical power, while minimizing losses due to heat, friction, and turbulence. Optimizing these factors is essential for achieving high performance, reliability, and cost-effectiveness in power generation and industrial applications.
This section will explore the key factors that impact the efficiency and performance of impulse turbines, including steam conditions, design elements, operational practices, and technological advancements.
1. Thermodynamic Efficiency and Energy Conversion
The primary goal of an impulse turbine is to convert as much of the thermal energy from the steam as possible into mechanical energy. This conversion process can be understood through the Rankine cycle, which is a thermodynamic cycle used to describe steam power generation. The efficiency of this cycle is determined by how effectively the turbine can convert steam’s pressure and temperature into rotational energy.
1.1 Rankine Cycle and Energy Conversion
- Heat Addition: High-pressure, high-temperature steam is produced in a boiler by heating water. The energy added to the water is stored as thermal energy in the steam.
- Expansion in Nozzles: Steam is directed into the turbine nozzles, where it expands and loses pressure, gaining kinetic energy. This process converts the steam’s thermal energy into kinetic energy, forming high-velocity jets.
- Mechanical Energy Conversion: The high-speed jets strike the turbine blades, causing the rotor to spin. This mechanical motion can then be used to drive generators or other machinery.
- Heat Rejection and Recycling: After passing through the turbine, the steam exits at a lower pressure and temperature. It is then condensed and recycled back to the boiler.
The overall efficiency of the Rankine cycle is influenced by several factors, including the pressure and temperature of the steam, the effectiveness of the nozzles and blades, and the ability to minimize losses throughout the system.
2. Factors Affecting the Efficiency of Impulse Turbines
Several critical factors impact the efficiency of impulse turbines, from the properties of the steam to the design and operation of the turbine itself.
2.1 Steam Conditions
The properties of the steam used in impulse turbines—namely its pressure, temperature, and dryness—significantly influence the efficiency of energy conversion.
- Steam Pressure:
- Higher steam pressure allows for greater expansion in the nozzles, leading to higher velocities when the steam exits. This increases the kinetic energy available to drive the turbine blades, resulting in greater mechanical output.
- Maintaining optimal pressure levels is essential, as variations can lead to inconsistent performance and reduced efficiency. Too low a pressure will result in inadequate velocity, while too high a pressure can cause excessive wear on components.
- Steam Temperature:
- High-temperature steam carries more energy and allows for more efficient energy conversion. Increasing the temperature beyond the boiling point (superheating) prevents the steam from condensing, which could cause erosion and reduce efficiency.
- Superheating the steam can also improve efficiency by increasing the amount of energy extracted during expansion. However, the design of the turbine must accommodate these higher temperatures.
- Steam Dryness:
- Steam dryness refers to the proportion of water droplets within the steam. “Dry” steam (close to 100% vapor) is ideal because water droplets can cause erosion of the blades and reduce efficiency.
- Proper control of steam dryness is necessary to prevent damage and ensure smooth, efficient operation. This can be achieved through steam separators and reheaters.
2.2 Nozzle Efficiency
The efficiency of the nozzles in an impulse turbine directly affects how well the turbine converts steam’s thermal energy into kinetic energy. Nozzles play a critical role in expanding the steam and accelerating it into high-velocity jets that can drive the turbine blades.
- Nozzle Design:
- The design of the nozzles must ensure smooth expansion of the steam, with minimal turbulence and frictional losses. Well-designed nozzles help direct the steam accurately onto the blades, maximizing energy transfer.
- Variations in nozzle shape, such as convergent or convergent-divergent (de Laval) nozzles, are used depending on the desired speed and operating conditions. Convergent-divergent nozzles can achieve supersonic speeds, useful for high-performance turbines.
- Flow Alignment:
- Proper alignment of the nozzles ensures that the steam strikes the blades at the optimal angle. Misaligned nozzles can lead to energy loss through turbulence and improper momentum transfer, reducing overall efficiency.
- The maintenance of precise nozzle settings is important for consistent performance, especially in multi-stage turbines where nozzles play a critical role at each stage.
3. Blade Design and Aerodynamics
The design of the turbine blades is crucial for ensuring efficient energy conversion. Blades need to be shaped and positioned to capture the maximum amount of kinetic energy from the steam jets, while minimizing losses due to drag, turbulence, and friction.
3.1 Blade Shape and Curvature
- Bucket-Shaped Design:
- Impulse turbine blades are often bucket-shaped, allowing them to capture and redirect steam jets effectively. The curvature of the blades ensures that the steam changes direction smoothly, creating an impulse force that drives the blades and rotor.
- Proper blade curvature minimizes energy losses and maximizes the impulse force, resulting in more efficient energy conversion.
- Aerodynamic Optimization:
- The aerodynamic properties of the blades are critical for reducing drag and ensuring smooth flow of steam. Modern blade designs use computational fluid dynamics (CFD) to optimize the shape and alignment, reducing turbulence and enhancing efficiency.
- Aerodynamically optimized blades help ensure that the steam flows over the blades with minimal resistance, reducing frictional losses.
3.2 Blade Material and Durability
- Material Selection:
- The choice of materials for turbine blades is important for maintaining performance and longevity. High-strength alloys, such as stainless steel or titanium, are often used to withstand high temperatures and pressures.
- Blades must also be resistant to erosion and corrosion, which can be caused by impurities in the steam. Protective coatings and advanced alloys can enhance durability and reduce maintenance needs.
- Thermal and Mechanical Stress Management:
- Impulse turbines must be designed to handle thermal expansion and mechanical stresses without suffering damage or losing efficiency. Blade materials and designs must accommodate these changes, preventing cracking, warping, or other issues.
4. Operational Factors and Load Conditions
The operation of impulse turbines can greatly impact their efficiency. Factors such as load variations, maintenance practices, and operational settings all play a role in determining performance.
4.1 Load Management
- Full-Load vs. Partial-Load Operation:
- Impulse turbines are generally more efficient when operating at full load, where the steam flow and pressure conditions are optimized. At partial loads, efficiency can drop due to changes in steam flow dynamics and pressure.
- Multi-stage turbines with velocity or pressure compounding are better suited for handling variable loads, as they can adjust to changing steam conditions without significant loss of efficiency.
- Steady vs. Variable Loads:
- Operating turbines under steady loads allows for more consistent and efficient performance. Frequent changes in load can lead to fluctuations in pressure and temperature, resulting in inefficiencies.
- Proper control systems and governors are essential for adjusting the turbine’s operation based on load requirements, maintaining optimal performance even under variable conditions.
4.2 Maintenance and Component Integrity
- Regular Inspection and Maintenance:
- Routine maintenance is crucial for maintaining the efficiency of impulse turbines. Components such as nozzles, blades, and bearings must be inspected regularly to ensure they are functioning correctly.
- Any signs of wear, misalignment, or damage should be addressed promptly, as these issues can lead to increased friction, energy loss, and potential failure.
- Lubrication and Cooling:
- Proper lubrication of bearings and moving parts reduces friction and prevents overheating. Efficient cooling systems also help maintain consistent operating temperatures, ensuring the turbine runs smoothly.
- Modern impulse turbines often incorporate advanced lubrication and cooling systems that enhance performance and reliability, especially under high-load conditions.
5. Technological Innovations for Improved Efficiency
Technological advancements have led to the development of more efficient and reliable impulse turbines. Innovations in materials, design, and digital control systems have made it possible to achieve higher efficiencies and better performance across a range of applications.
5.1 Advanced Computational Design
- Computational Fluid Dynamics (CFD):
- CFD technology allows engineers to simulate and optimize the flow of steam through the nozzles and blades. This leads to more precise designs that minimize turbulence, friction, and energy losses.
- Advanced simulations help identify the best configurations for nozzles, blades, and casings, ensuring that the turbine operates at peak efficiency.
- 3D Printing and Precision Manufacturing:
- Modern manufacturing techniques, such as 3D printing, allow for the creation of complex blade geometries that would be difficult to achieve through traditional methods. This results in more aerodynamic and efficient designs.
- Precision manufacturing ensures that all components are built to exact specifications, reducing the risk of misalignment and mechanical stress.
5.2 Improved Control Systems
- Digital Monitoring and Automation:
- Digital monitoring systems allow operators to track the performance of impulse turbines in real time, identifying potential issues before they lead to inefficiencies or failures.
- Automation systems can adjust operating parameters based on real-time data, optimizing the turbine’s performance and maintaining consistent efficiency across different load conditions.
- Artificial Intelligence (AI) and Machine Learning:
- AI and machine learning algorithms can analyze data from turbine operations to predict maintenance needs, optimize performance settings, and improve efficiency. These technologies help reduce downtime and improve the longevity of the turbines.
- Predictive maintenance systems use AI to anticipate when components may require repair or replacement, preventing unexpected failures and maintaining optimal efficiency.
6. Enhancements Through Combined Cycles
One of the most effective ways to improve the efficiency of impulse turbines is by integrating them into combined cycle power plants. In a combined cycle setup, a gas turbine is used to generate electricity, and the waste heat from the gas turbine is used to produce steam for an impulse turbine.
- Benefits of Combined Cycles:
- Combined cycles can achieve efficiencies of over 60%, much higher than standalone impulse turbines. This is because they utilize the waste heat from the gas turbine, converting it into additional electrical power.
- The combination of gas and steam turbines allows for more flexible operation, handling peak and variable loads more effectively.
Conclusion
The efficiency and performance of impulse turbines depend on a range of factors, including the conditions of the steam, the design of the turbine components, and the operational practices in place. By optimizing these factors, it is possible to achieve higher energy conversion rates, reduced energy losses, and improved reliability. Modern technological advancements, from computational design tools to digital control systems, have further enhanced the efficiency of impulse turbines, making them indispensable components in power generation and industrial applications. As new materials, designs, and technologies continue to emerge, the future of impulse turbines promises even greater efficiency and sustainability in energy production.
Applications of Impulse Turbines: Versatile Uses Across Industries
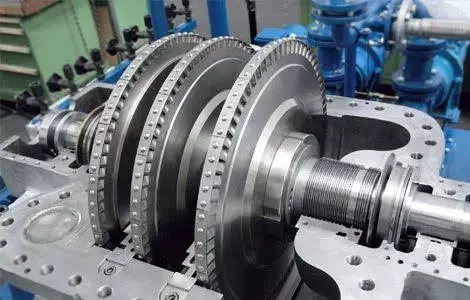
Impulse turbines are renowned for their simple yet efficient design, making them a popular choice for various applications where reliable mechanical power is needed. These turbines work by converting the kinetic energy of high-velocity steam jets into mechanical energy, which can then be used to generate electricity or drive machinery. Over the years, impulse turbines have proven to be versatile and efficient, finding applications in power generation, industrial processes, and even marine propulsion.
This section explores the wide range of applications for impulse turbines, focusing on their use in power plants, industrial setups, marine vessels, and other specialized areas. We will also discuss how advances in technology have expanded the utility of impulse turbines across different sectors.
1. Power Generation
The primary and most widespread application of impulse turbines is in the field of power generation. Their efficiency, reliability, and ability to handle varying loads make them ideal for converting steam energy into electricity in power plants. Impulse turbines are especially favored in smaller to medium-sized power generation facilities but can also be found in larger setups when integrated with other systems.
1.1 Thermal Power Plants
- Coal-Fired and Gas-Fired Power Plants:
- Impulse turbines are used in coal-fired and gas-fired power plants to generate electricity. In these plants, water is heated to produce high-pressure steam, which is directed through nozzles to create high-velocity jets. These jets then strike the turbine blades, causing the rotor to spin and drive an electrical generator.
- While these turbines may not be as common in very large-scale power plants that use multi-stage reaction turbines, they are ideal for smaller plants where high-speed, efficient power generation is required.
- Combined Cycle Power Plants:
- In combined cycle power plants, a gas turbine generates electricity, and the waste heat from the gas turbine is used to produce steam for an impulse turbine. This arrangement maximizes efficiency by utilizing the waste heat that would otherwise be lost.
- Impulse turbines in this configuration help achieve higher overall efficiency, making combined cycle plants one of the most efficient ways to generate electricity. These systems can achieve efficiencies of over 60%, much higher than traditional standalone plants.
1.2 Decentralized and Small-Scale Power Generation
- Small Industrial Power Plants:
- Many small industrial facilities use impulse turbines to generate power on-site, especially in regions where connecting to the central power grid may be challenging. Impulse turbines are ideal for these setups due to their compact size and straightforward design.
- These turbines can be used to generate power for industrial operations while also providing process steam for heating, making them a key component of cogeneration systems.
- Renewable Energy Integration:
- Although impulse turbines are traditionally associated with fossil-fuel power generation, they are also integrated into renewable energy systems, such as biomass plants. In these setups, biomass is burned to produce steam, which then drives the impulse turbine to generate electricity.
- The use of impulse turbines in renewable energy applications contributes to cleaner power generation and supports sustainable energy goals.
2. Industrial Applications
Impulse turbines play a crucial role in various industrial processes by providing mechanical power to drive machinery. Their ability to operate at high speeds and deliver consistent power output makes them suitable for a range of industrial uses.
2.1 Mechanical Drives for Industrial Equipment
- Pumps and Compressors:
- Impulse turbines are often used to drive pumps and compressors in industries such as oil and gas, chemical processing, and water treatment. These turbines provide reliable mechanical power, ensuring that pumps and compressors operate efficiently and consistently.
- High-speed impulse turbines can directly drive centrifugal compressors, which are essential for processes that involve gas compression, such as natural gas processing and air separation.
- Blowers and Fans:
- In industrial settings that require the movement of air or gases, impulse turbines are used to power blowers and fans. These are particularly common in industries where ventilation, cooling, or air circulation is necessary.
- The efficient, high-speed operation of impulse turbines ensures that blowers and fans can handle large volumes of air without interruptions.
2.2 Cogeneration and Combined Heat and Power (CHP) Systems
- Efficient Use of Energy:
- In cogeneration systems, also known as combined heat and power (CHP) systems, impulse turbines generate electricity and provide heat for industrial processes. The ability to use the same steam for both electricity generation and heating makes these systems highly efficient.
- For example, in a paper mill, an impulse turbine can generate power for the mill’s operations while also supplying steam for the drying process, leading to more efficient energy usage and cost savings.
- Versatile Energy Supply:
- Impulse turbines in cogeneration setups can adapt to fluctuating energy demands, ensuring that industrial facilities have a consistent power and heat supply. This versatility is essential for industries where power demands vary significantly throughout the day.
3. Marine Propulsion
Impulse turbines have a long history of use in marine propulsion, particularly in ships that require high-speed and reliable power. Although modern vessels often use diesel engines, impulse turbines remain relevant for certain applications, particularly in military and specialized commercial ships.
3.1 Steam Turbine-Driven Ships
- Naval Vessels:
- Historically, impulse turbines were widely used to power naval vessels, especially during the early and mid-20th century. These turbines provided the high-speed propulsion needed for warships, which required reliable and powerful engines.
- Although many naval vessels today have switched to diesel engines or gas turbines, some still use steam turbines, especially for aircraft carriers and submarines where stealth and endurance are crucial. Steam turbines, including impulse designs, offer quieter operation compared to diesel engines, reducing the acoustic signature of the vessel.
- Commercial Shipping:
- Certain commercial ships, such as icebreakers and cargo vessels, use steam turbines to power their propulsion systems. Impulse turbines can be part of these setups, providing the mechanical energy needed to turn the propellers and drive the ship forward.
- These turbines are particularly beneficial in vessels that require high-speed operation and steady power output over long distances. The durability and reliability of impulse turbines make them well-suited for maritime conditions, where maintenance opportunities may be limited.
4. Specialized Applications and Niche Markets
Beyond conventional power generation, industrial processes, and marine propulsion, impulse turbines also find use in various niche markets and specialized applications. Their adaptability and robust design make them suitable for a range of unique scenarios.
4.1 Power Recovery in Industrial Processes
- Turboexpanders:
- Impulse turbines can act as turboexpanders in industrial setups, recovering energy from high-pressure gas streams. Turboexpanders are used in industries such as natural gas processing, where high-pressure gas needs to be expanded and cooled. The impulse turbine converts the energy from this process into mechanical power, which can be used to drive generators or other machinery.
- This energy recovery process improves the overall efficiency of the industrial operation, reducing waste and lowering operational costs.
- Geothermal Energy Systems:
- Impulse turbines are sometimes used in geothermal power plants, where high-pressure steam from geothermal reservoirs is used to drive the turbine. While geothermal plants more commonly use reaction turbines due to their ability to handle varying steam pressures, impulse turbines are employed in specific setups where the steam pressure can be managed effectively.
- In these plants, the ability of impulse turbines to operate efficiently at high speeds makes them a viable option for generating clean, renewable energy.
4.2 Experimental and Research Applications
- Aerospace and Test Facilities:
- Impulse turbines are used in experimental setups and test facilities for aerospace research. Their high-speed operation allows them to simulate the conditions needed for testing jet engines, compressors, and other high-speed machinery.
- Research facilities use impulse turbines to study aerodynamic behaviors and improve the design of other rotating equipment, such as gas turbines and fans. The insights gained from these studies can lead to the development of more efficient and reliable machinery for various industries.
- Cryogenic and Refrigeration Systems:
- In cryogenic and refrigeration systems, impulse turbines can be used to drive compressors that are responsible for cooling gases to extremely low temperatures. The efficiency and reliability of impulse turbines make them suitable for these specialized applications, where consistent performance is essential.
5. Future Trends and Emerging Applications
The future of impulse turbines looks promising, with technological advancements opening up new possibilities for their use. Developments in material science, digital control systems, and energy efficiency are making impulse turbines more adaptable and capable than ever before.
5.1 Integration with Renewable Energy
- Biomass and Waste-to-Energy Plants:
- Impulse turbines are increasingly being integrated into biomass and waste-to-energy plants, where organic waste is burned to produce steam. These setups convert waste materials into useful energy, reducing environmental impact and supporting sustainability efforts.
- The use of impulse turbines in these plants ensures efficient energy conversion, helping to make renewable energy sources more competitive with traditional fossil fuels.
- Hybrid Power Systems:
- Hybrid power systems combine different energy sources, such as solar, wind, and biomass, to provide a more reliable and balanced energy supply. Impulse turbines play a role in these systems by efficiently converting steam generated from renewable sources into electricity.
- The flexibility of impulse turbines allows them to adapt to varying energy inputs, making them a valuable component in hybrid power setups that aim to minimize reliance on non-renewable energy.
5.2 Advances in Turbine Design and Digitalization
- Digital Monitoring and Automation:
- Modern impulse turbines are equipped with digital monitoring systems that track performance, temperature, pressure, and other parameters in real time. This allows operators to optimize the turbine’s efficiency and anticipate maintenance needs before issues arise.
- Automation systems can adjust the turbine’s operation based on real-time data, ensuring optimal performance even under fluctuating load conditions. This integration of digital technology enhances the reliability and lifespan of impulse turbines.
- New Materials and Manufacturing Techniques:
- The development of advanced materials, such as high-temperature alloys and ceramics, is leading to the production of more durable and efficient turbine components. These materials allow impulse turbines to operate at higher temperatures and pressures, improving their performance in demanding environments.
- Additive manufacturing (3D printing) is also being used to create complex turbine components with high precision, reducing the cost and time required for production. This makes it easier to design customized turbines for specific applications, expanding their utility across different industries.
Conclusion
Impulse turbines have proven to be versatile, efficient, and reliable machines, suitable for a wide range of applications. From traditional power generation in thermal plants to driving industrial machinery and marine propulsion, these turbines have demonstrated their value across multiple sectors. Advances in technology have further enhanced their performance, enabling their integration into renewable energy systems and specialized industrial processes.
As industries continue to evolve and prioritize efficiency and sustainability, the role of impulse turbines will likely expand, finding new applications and integrating with modern energy solutions. With ongoing innovations in design, materials, and digital control, impulse turbines remain a crucial component of the global energy landscape, supporting both traditional and emerging industries.
Power Generation with Steam Turbines: A Comprehensive Overview
Steam turbines are a cornerstone of modern power generation, converting the thermal energy of steam into mechanical energy, which can then be used to produce electricity. This technology has been a driving force in the energy sector for over a century, powering everything from small industrial facilities to large-scale power plants. Steam turbines play a crucial role in thermal power plants, where various fuel sources are used to produce steam, which drives the turbines to generate electricity.
This section provides an in-depth look at how steam turbines are used in power generation, including their working principles, types of power plants, efficiency factors, and technological advancements. It also explores the benefits and challenges associated with steam turbine-based power generation, highlighting its significance in the global energy mix.
1. The Working Principle of Steam Turbines in Power Generation
Steam turbines operate on a straightforward principle: they convert the thermal energy of steam into mechanical energy through the expansion of steam across turbine blades. This mechanical energy is then converted into electrical energy via a generator. The entire process can be broken down into several key stages:
1.1 Steam Generation
- Boiler Operation:
- Water is heated in a boiler to produce high-pressure, high-temperature steam. The energy required for this process can come from burning fossil fuels (coal, natural gas, oil), nuclear reactions, or renewable sources (biomass, concentrated solar power).
- The steam produced is superheated, meaning it is heated beyond its boiling point to prevent condensation within the turbine, which can lead to inefficiencies and damage.
1.2 Expansion in the Steam Turbine
- Nozzle Action and Blade Movement:
- The high-pressure steam is directed into the turbine through nozzles, where it expands and accelerates. The nozzles convert the thermal energy into kinetic energy, forming high-velocity jets of steam.
- These jets then hit the turbine blades, causing them to rotate. The blades are designed to change the direction of the steam, creating an impulse or reaction force that drives the rotor. This is where the mechanical energy is generated.
- In multi-stage turbines, the steam passes through several stages of nozzles and blades, gradually losing pressure and transferring energy at each stage.
1.3 Energy Conversion and Electricity Generation
- Driving the Generator:
- The rotating turbine shaft is connected to an electric generator. As the turbine spins, it drives the generator, which converts the mechanical energy into electrical energy.
- The generated electricity is then transmitted to the power grid, where it can be distributed to homes, businesses, and industries.
1.4 Exhaust and Condensation
- Condensing Steam for Efficiency:
- After passing through the turbine, the steam exits at a much lower pressure and temperature. It is then directed to a condenser, where it cools and condenses back into water.
- The condensed water is pumped back to the boiler, completing the cycle. This closed-loop system helps improve efficiency and conserves water.
2. Types of Power Plants Using Steam Turbines
Steam turbines are used in various types of power plants, each utilizing different fuel sources and technologies to produce steam. The main types include thermal power plants, nuclear power plants, and renewable energy plants.
2.1 Thermal Power Plants
- Coal-Fired Power Plants:
- Coal remains one of the most common fuels for power generation worldwide. In coal-fired power plants, coal is burned in a furnace to produce heat, which is used to convert water into steam.
- Despite concerns about emissions, coal plants are still prevalent, especially in regions with abundant coal resources. Modern coal plants have integrated technologies to reduce emissions and improve efficiency, such as supercritical and ultra-supercritical steam conditions.
- Natural Gas Power Plants:
- Natural gas power plants operate similarly to coal plants but use natural gas as the fuel. These plants are often more efficient and produce fewer emissions compared to coal plants.
- Combined cycle plants utilize both gas turbines and steam turbines, increasing efficiency by using the waste heat from the gas turbines to generate additional steam for the steam turbine. This combined approach can achieve efficiencies of over 60%.
- Oil-Fired Power Plants:
- Less common today, oil-fired plants work on the same principles as coal and gas plants. They are typically used in regions where oil is abundant or in places where fuel supply diversity is necessary.
2.2 Nuclear Power Plants
- Using Nuclear Reactions to Produce Steam:
- In nuclear power plants, the heat generated by nuclear fission reactions is used to produce steam. Uranium or plutonium fuel rods undergo fission, releasing a tremendous amount of heat, which is then transferred to water to generate steam.
- Nuclear plants are known for their ability to provide a consistent and reliable power supply without direct carbon emissions. However, the process of managing nuclear waste and ensuring safety is a significant challenge.
- Steam Turbine Role in Nuclear Plants:
- The steam turbines in nuclear plants are typically large and operate at lower temperatures compared to fossil-fuel plants, but they are designed for continuous, stable operation. Multi-stage reaction turbines are common due to their ability to handle high steam volumes efficiently.
2.3 Renewable Energy Plants
- Biomass Power Plants:
- Biomass plants use organic materials, such as wood chips, agricultural waste, or dedicated energy crops, to produce steam. This process involves burning the biomass to heat water, similar to fossil-fuel plants.
- Impulse and reaction turbines can both be used in biomass plants, depending on the design and scale of the facility. Biomass energy is considered renewable because the carbon dioxide released during combustion is roughly equivalent to the amount absorbed by the plants during their growth.
- Geothermal and Solar Thermal Plants:
- Geothermal plants use steam extracted from underground geothermal reservoirs to drive turbines. The naturally occurring steam or hot water is brought to the surface, where it is used to generate electricity. These plants are mostly located in geologically active regions.
- Solar thermal plants use mirrors to concentrate sunlight onto a receiver, where the heat is used to produce steam. This steam drives turbines to generate electricity, offering a clean and renewable way to harness solar energy.
3. Efficiency Factors in Steam Turbine Power Plants
The efficiency of power generation using steam turbines depends on several factors, including the design of the turbine, the quality of steam, and the type of plant setup. Improving efficiency is crucial for reducing fuel consumption, emissions, and operational costs.
3.1 Steam Conditions (Pressure and Temperature)
- High-Pressure, High-Temperature Steam:
- The higher the pressure and temperature of the steam, the more energy it contains. Superheating the steam (raising the temperature above its boiling point) allows for more efficient energy conversion in the turbine.
- Modern power plants often use supercritical and ultra-supercritical steam conditions, where the water is heated to a point where it no longer behaves as a typical liquid or gas, allowing for higher efficiencies.
- Dryness of Steam:
- Ensuring that the steam remains dry (free of water droplets) is important for preventing erosion of the turbine blades and maintaining efficiency. Water droplets can cause pitting and wear on the blades, reducing performance.
3.2 Multi-Stage Turbines
- Increased Efficiency with Multiple Stages:
- Multi-stage turbines are designed to extract more energy from the steam by expanding it through several stages. Each stage involves a set of nozzles and blades, with the steam losing pressure and transferring energy at each stage.
- Impulse Turbines and Reaction Turbines: Multi-stage impulse turbines are often used for smaller, high-speed applications, while reaction turbines are favored in larger power plants for their ability to handle high steam volumes more efficiently.
3.3 Combined Cycle and Cogeneration Systems
- Combined Cycle Power Plants:
- Combined cycle plants improve efficiency by using both gas and steam turbines. The gas turbine generates electricity, and the waste heat from the exhaust is used to produce steam, which drives a steam turbine.
- This configuration can achieve efficiencies of up to 60%, significantly higher than single-cycle power plants.
- Cogeneration (CHP) Systems:
- Cogeneration, or Combined Heat and Power (CHP) systems, use steam turbines to generate electricity and provide heat for industrial processes. By utilizing the waste heat from power generation, these systems can achieve efficiencies of 80% or higher.
- CHP systems are particularly popular in industries where both electricity and process heat are needed, such as in chemical manufacturing, food processing, and paper production.
4. Technological Advancements and Future Trends
The field of steam turbine power generation continues to evolve, with new technologies aimed at improving efficiency, reducing emissions, and integrating with renewable energy systems.
4.1 Advanced Materials and Blade Designs
- High-Temperature Alloys and Coatings:
- The development of high-temperature alloys and coatings has enabled turbines to operate at higher temperatures and pressures, improving efficiency. These materials help protect blades from wear, corrosion, and thermal stress.
- New blade designs, developed using computational fluid dynamics (CFD), optimize steam flow and reduce losses due to turbulence and drag, leading to greater energy extraction.
4.2 Digital Monitoring and Automation
- Smart Turbine Systems:
- Modern steam turbines are equipped with digital monitoring systems that track performance, temperature, pressure, and other parameters in real time. This allows for proactive maintenance, minimizing downtime and optimizing efficiency.
- Automation systems can adjust operating parameters based on real-time data, ensuring that the turbine operates at peak performance under varying load conditions.
4.3 Integration with Renewable Energy
- Hybrid Power Systems:
- Steam turbines are increasingly being integrated into hybrid power systems that combine renewable energy sources with traditional fuel-based systems. For example, solar thermal plants can be combined with natural gas plants to ensure a consistent power supply, even when sunlight is not available.
- Steam turbines also play a role in energy storage systems, where excess electricity from renewables is used to generate steam, which can be stored and later used to generate electricity when needed.
5. Benefits and Challenges of Steam Turbine Power Generation
5.1 Benefits
- High Efficiency and Reliability:
- Steam turbines are known for their ability to convert energy efficiently, especially in combined cycle and cogeneration systems. Their design allows for consistent, reliable operation, making them a dependable source of electricity.
- Versatility:
- Steam turbines can be used with a wide range of fuel sources, from fossil fuels to nuclear and renewable energy, offering flexibility in power generation.
- Scalability:
- Steam turbine systems can be scaled to meet different power needs, from small industrial plants to large power stations, making them suitable for a variety of applications.
5.2 Challenges
- Environmental Concerns:
- The use of fossil fuels to generate steam can lead to greenhouse gas emissions, contributing to climate change. Efforts are being made to reduce emissions through cleaner technologies and the integration of renewables.
- High Initial Costs:
- Setting up steam turbine power plants, especially those using advanced technologies or nuclear energy, requires significant capital investment. However, the long-term operational savings often justify these costs.
- Maintenance and Safety:
- Steam turbines, especially in nuclear power plants, require rigorous maintenance and safety protocols to prevent accidents and ensure continuous operation. This includes managing high-pressure systems and ensuring the integrity of turbine components.
Conclusion
Steam turbines have been a fundamental part of power generation for over a century, offering an efficient and reliable way to convert thermal energy into electricity. Whether used in traditional fossil-fuel plants, nuclear facilities, or integrated with renewable energy systems, steam turbines remain a vital component of the global energy infrastructure. Technological advancements in materials, design, and digital controls continue to improve their performance, making them more adaptable and efficient than ever before. As the world moves towards cleaner and more sustainable energy solutions, steam turbines will likely play a crucial role in bridging the gap between traditional and renewable power sources.
Thermal Power Plants: An In-Depth Overview
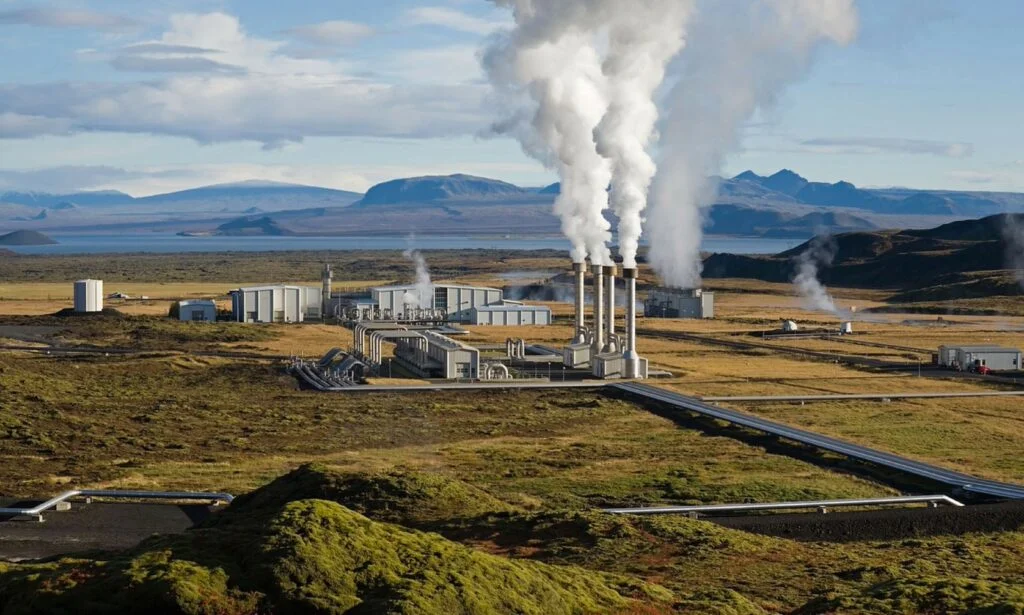
Thermal power plants are a cornerstone of global electricity production, generating power by converting heat energy into electrical energy. They operate on the fundamental principle of the Rankine cycle, which involves the generation of steam from water, the expansion of this steam to produce mechanical energy, and the subsequent conversion of this mechanical energy into electrical energy. Thermal power plants can use various fuel sources, including fossil fuels (coal, natural gas, oil), nuclear energy, and even renewable biomass, to generate the heat needed for steam production.
This section provides a comprehensive overview of thermal power plants, exploring their working principles, types, key components, efficiency factors, and the benefits and challenges they present. Understanding how these plants operate is essential to appreciating their role in the global energy mix and the ongoing efforts to make power generation more efficient and sustainable.
1. How Thermal Power Plants Work
Thermal power plants generate electricity by harnessing heat energy to produce steam, which drives a turbine connected to a generator. The process involves several key steps, as outlined below:
1.1 Fuel Combustion and Heat Generation
- Boiler Operation:
- In a thermal power plant, the first step is the combustion of fuel to produce heat. This fuel can be coal, natural gas, oil, biomass, or nuclear fuel.
- The heat generated from the combustion process is used to convert water into high-pressure steam. In a nuclear power plant, the heat comes from the nuclear fission of uranium or plutonium atoms.
- The efficiency of the combustion process and the quality of the fuel play a significant role in determining the overall efficiency of the power plant.
1.2 Steam Production and Superheating
- Steam Generation:
- The water is heated in a boiler to produce steam. The steam is then superheated to increase its temperature and energy content, which helps improve the efficiency of the energy conversion process.
- Superheated steam is preferred because it reduces the risk of condensation in the turbine, which can cause erosion of the turbine blades and loss of efficiency.
1.3 Expansion in the Steam Turbine
- Energy Conversion:
- The high-pressure, high-temperature steam is directed to a steam turbine, where it expands and drives the turbine blades. This expansion process converts the thermal energy of the steam into mechanical energy by causing the turbine rotor to spin.
- The turbine is connected to an electric generator, which converts the mechanical energy into electrical energy that can be transmitted to the power grid.
1.4 Exhaust and Condensation
- Steam Condensation:
- After passing through the turbine, the steam exits at a much lower pressure and temperature. This exhaust steam is directed to a condenser, where it is cooled and condensed back into water.
- The condensed water is then pumped back to the boiler, completing the cycle. This closed-loop system helps conserve water and improve overall plant efficiency.
2. Types of Thermal Power Plants
Thermal power plants can be classified based on the fuel used to generate heat. Each type has its own operational characteristics, benefits, and challenges. The main types include:
2.1 Coal-Fired Power Plants
- Operation:
- Coal is the most common fuel used in thermal power plants globally. In these plants, pulverized coal is burned in a furnace to generate heat, which converts water into steam.
- The steam drives a turbine connected to a generator, producing electricity. Despite concerns over emissions, coal plants are still widely used, particularly in countries with abundant coal reserves.
- Advantages and Challenges:
- Coal plants are reliable and capable of generating large amounts of electricity. However, they are also associated with high carbon emissions and air pollutants, including sulfur dioxide (SO₂) and nitrogen oxides (NOx).
- Modern coal plants have integrated technologies, such as flue gas desulfurization and selective catalytic reduction, to reduce emissions. Advanced designs like supercritical and ultra-supercritical plants further improve efficiency and reduce emissions.
2.2 Natural Gas Power Plants
- Operation:
- Natural gas plants burn natural gas to produce heat, which is used to generate steam. Natural gas plants are generally more efficient and cleaner than coal-fired plants.
- A common design is the combined cycle power plant, which uses both gas and steam turbines. The gas turbine generates electricity, and the waste heat from its exhaust is used to produce steam for a steam turbine, maximizing energy extraction and efficiency.
- Advantages and Challenges:
- Natural gas plants emit less carbon dioxide (CO₂) and other pollutants compared to coal plants, making them a cleaner option for power generation.
- However, natural gas is still a fossil fuel, and concerns about methane emissions during extraction and transport present environmental challenges. Combined cycle plants are highly efficient, with efficiencies reaching 60% or higher.
2.3 Nuclear Power Plants
- Operation:
- Nuclear plants use the heat produced from nuclear fission reactions to generate steam. Uranium or plutonium fuel rods undergo fission, releasing energy that is used to heat water.
- The steam drives a turbine, similar to coal and gas plants, but without the direct combustion of fossil fuels. Nuclear plants provide a stable and reliable source of electricity.
- Advantages and Challenges:
- Nuclear power is a low-carbon energy source, as it does not emit CO₂ during operation. This makes it a key player in reducing greenhouse gas emissions.
- However, the management of radioactive waste, the high cost of plant construction, and safety concerns related to accidents and nuclear proliferation are significant challenges. Advanced reactor designs aim to address some of these issues.
2.4 Biomass Power Plants
- Operation:
- Biomass plants burn organic materials, such as wood chips, agricultural waste, and dedicated energy crops, to generate heat for steam production. This process is similar to coal combustion but uses renewable sources.
- The carbon released during biomass combustion is roughly equivalent to the amount absorbed by the plants during their growth, making biomass a carbon-neutral energy source when managed sustainably.
- Advantages and Challenges:
- Biomass plants support sustainable energy goals by using waste materials and reducing reliance on fossil fuels. They can be integrated into existing coal plants, reducing the need for new infrastructure.
- Challenges include the availability of biomass resources, the logistics of collection and transportation, and ensuring that biomass is sourced sustainably to avoid deforestation and other environmental impacts.
3. Key Components of Thermal Power Plants
Thermal power plants consist of several key components that work together to convert fuel energy into electricity efficiently. Each component plays a specific role in the process:
3.1 Boiler
- Function:
- The boiler is where the water is heated to produce steam. It contains a furnace where the fuel is burned, transferring heat to water-filled tubes.
- In coal-fired plants, pulverized coal is burned in a boiler furnace. In nuclear plants, the heat from fission reactions is transferred to a heat exchanger that acts as a boiler.
- Design Variations:
- Modern boilers are designed for high efficiency and can operate at supercritical or ultra-supercritical conditions, which means they can produce steam at temperatures and pressures beyond the critical point of water, improving efficiency.
3.2 Steam Turbine
- Function:
- The steam turbine is the heart of the power plant, where steam energy is converted into mechanical energy. The high-pressure steam drives the turbine blades, causing the rotor to spin.
- Turbines are designed to handle high temperatures and pressures, and multi-stage designs allow for efficient energy extraction across different pressure levels.
- Types of Turbines:
- Impulse turbines use nozzles to direct high-speed steam jets onto the blades. Reaction turbines rely on pressure drops across both stationary and moving blades, making them suitable for larger plants with high steam volumes.
3.3 Generator
- Function:
- The generator is connected to the turbine and converts the mechanical energy from the rotating turbine into electrical energy. It does this through electromagnetic induction, where the movement of the turbine shaft induces an electrical current in the generator’s coils.
- The generated electricity is then transmitted to the power grid for distribution.
3.4 Condenser
- Function:
- After passing through the turbine, the steam is directed to a condenser, where it is cooled and condensed back into water. This is an essential part of the power cycle, as it allows the water to be recycled and reused in the boiler.
- Condensers use cooling water from nearby water bodies or cooling towers to cool the steam efficiently. In coastal power plants, seawater is often used as the cooling medium.
3.5 Cooling System
- Cooling Towers:
- Cooling towers are used to dissipate the heat from the condenser into the atmosphere. They release the excess heat in the form of water vapor, helping to maintain the temperature of the power plant’s components.
- Plants located near large water bodies may use water cooling systems instead of cooling towers, drawing in water to cool the steam and then discharging it back.
4. Efficiency Factors in Thermal Power Plants
The efficiency of thermal power plants is determined by how effectively they convert fuel energy into electrical energy. Improving efficiency is crucial for reducing fuel consumption, emissions, and operational costs.
4.1 Supercritical and Ultra-Supercritical Technology
- Higher Efficiency:
- Supercritical and ultra-supercritical plants operate at higher temperatures and pressures, which allows for more efficient steam generation and expansion. These plants can achieve efficiencies of 40% to 50%, compared to 30% to 35% for traditional subcritical plants.
- By improving the thermal efficiency, these advanced plants reduce the amount of fuel needed and, consequently, the emissions produced.
4.2 Combined Cycle Power Plants
- Combining Gas and Steam Cycles:
- Combined cycle plants use both gas and steam turbines to maximize energy extraction. The gas turbine generates electricity, and the waste heat from the exhaust is used to produce steam for a steam turbine.
- This setup can achieve efficiencies of up to 60%, making it one of the most efficient configurations for power generation. It is particularly popular in natural gas plants.
4.3 Cogeneration (CHP)
- Heat and Power Production:
- Cogeneration, or combined heat and power (CHP), systems use the waste heat from electricity generation for industrial processes, district heating, or other applications. This dual use of energy makes CHP systems highly efficient, with efficiencies of 80% or more.
- Cogeneration is commonly used in industrial plants, hospitals, and universities, where there is a need for both electricity and heat.
5. Advantages and Challenges of Thermal Power Plants
5.1 Advantages
- Reliable and Scalable:
- Thermal power plants provide a consistent and reliable source of electricity, making them ideal for meeting baseload demand. They can be scaled to generate power from a few megawatts to several gigawatts.
- Versatile Fuel Options:
- These plants can operate on a wide range of fuels, including coal, natural gas, oil, biomass, and nuclear energy, offering flexibility in fuel sourcing and energy security.
- Integration with Renewable Energy:
- Thermal power plants can be integrated with renewable sources like biomass and solar thermal, reducing carbon emissions and supporting sustainable energy goals.
5.2 Challenges
- Environmental Impact:
- The combustion of fossil fuels in thermal power plants results in CO₂ emissions, contributing to climate change. Plants also emit pollutants like sulfur dioxide, nitrogen oxides, and particulate matter, which can harm air quality and public health.
- Nuclear plants pose challenges related to radioactive waste disposal and safety concerns. Biomass plants require sustainable sourcing to avoid deforestation and environmental degradation.
- High Capital Costs:
- Setting up thermal power plants, especially those using advanced technologies or nuclear reactors, requires significant investment. However, once operational, these plants can provide a steady and reliable power supply for decades.
Conclusion
Thermal power plants have been the backbone of global electricity production for over a century, offering reliable and scalable power generation. With advancements in technology, these plants have become more efficient and cleaner, integrating renewable energy sources and employing advanced designs like combined cycle and cogeneration systems. Despite the environmental challenges associated with fossil fuels, thermal power plants remain crucial in the global energy mix, providing a steady supply of electricity to meet growing energy demands. As the world transitions to cleaner and more sustainable energy solutions, ongoing innovations in thermal power plant technology will play a key role in bridging the gap between traditional energy sources and future renewable systems.
Decentralized and Small-Scale Power Generation: A Comprehensive Overview
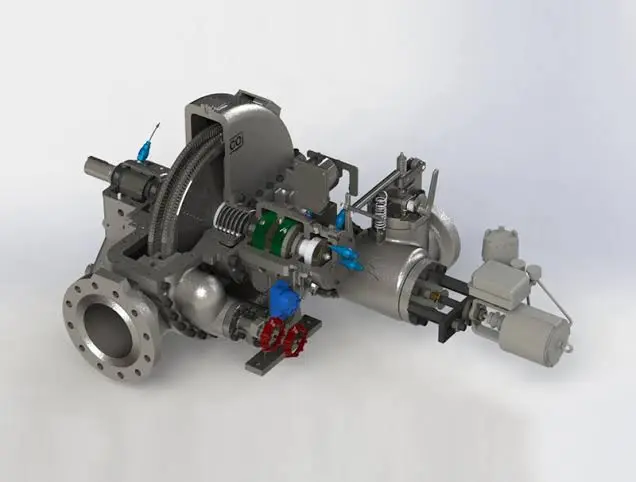
Decentralized and small-scale power generation refers to the production of electricity close to the point of use, rather than relying on large, centralized power plants that transmit electricity over long distances. This approach to power generation is becoming increasingly popular due to its potential to increase energy efficiency, enhance grid reliability, and support the integration of renewable energy sources. Unlike traditional power generation systems, which rely on massive infrastructure and centralized control, decentralized systems are more flexible, scalable, and capable of adapting to local energy needs.
This section explores the concept of decentralized and small-scale power generation, its benefits and challenges, various technologies employed, and its role in shaping a more sustainable and resilient energy future.
1. Understanding Decentralized Power Generation
Decentralized power generation involves producing electricity near where it will be used, such as in homes, businesses, or communities. This contrasts with centralized power plants, which generate electricity in bulk and distribute it across vast regions via transmission lines. Decentralized systems can operate independently or be integrated into the national grid to supplement power supply during peak demand or emergencies.
1.1 Key Characteristics of Decentralized Power Systems
- Local Energy Production:
- Electricity is generated close to where it is consumed, reducing transmission losses and improving overall system efficiency. This is particularly beneficial in rural or remote areas where extending the central grid would be expensive and impractical.
- Smaller-Scale Installations:
- Decentralized power generation systems are typically smaller than centralized plants, ranging from a few kilowatts (kW) for residential use to a few megawatts (MW) for industrial facilities or communities.
- Distributed Energy Resources (DERs):
- These systems often include distributed energy resources (DERs) such as solar panels, wind turbines, small gas turbines, and diesel generators. They can be stand-alone systems or interconnected to form a local energy network (microgrid).
1.2 Microgrids and Off-Grid Systems
- Microgrids:
- A microgrid is a small, self-sufficient energy system that can operate independently or connect to the main power grid. It combines various energy sources, including renewables, batteries, and backup generators, to provide reliable and resilient power.
- Microgrids can serve specific buildings, communities, or industrial sites. During a power outage, they can disconnect from the main grid and continue to provide electricity, ensuring continuous operation for critical services.
- Off-Grid Systems:
- Off-grid systems operate entirely independently of the main power grid. They are crucial for remote areas without access to traditional electricity infrastructure. These systems often rely on renewable energy sources, such as solar panels, wind turbines, and battery storage, to provide a sustainable power supply.
2. Technologies for Small-Scale Power Generation
Various technologies can be used for decentralized and small-scale power generation. The choice of technology depends on factors such as location, energy demand, and the availability of resources. Here are some of the most common technologies:
2.1 Solar Photovoltaic (PV) Systems
- How They Work:
- Solar PV systems convert sunlight directly into electricity using solar panels made of semiconductor materials (typically silicon). These systems can be installed on rooftops, in open fields, or as part of building-integrated systems.
- They are modular, scalable, and can range from small systems for residential use to large solar farms that supply power to entire communities.
- Applications:
- Ideal for homes, businesses, and off-grid locations. Solar PV is particularly effective in areas with abundant sunlight and can be combined with battery storage to provide power during cloudy days or nighttime.
- Advantages:
- Renewable and clean source of energy, low maintenance, and reduced electricity bills. Solar PV systems can also be installed relatively quickly and scaled to meet varying power demands.
2.2 Small Wind Turbines
- How They Work:
- Small wind turbines generate electricity by harnessing the kinetic energy of the wind. They are similar to larger wind turbines used in wind farms but are designed for lower power outputs, making them suitable for individual homes, farms, and small businesses.
- These systems can be stand-alone or connected to the grid, providing supplemental power during peak periods or when the wind is strong.
- Applications:
- Effective in rural areas, coastal regions, and other locations with consistent wind patterns. Small wind turbines can provide off-grid power or be integrated into microgrids.
- Advantages:
- Clean and renewable energy, can be installed in remote areas, and reduces dependency on fossil fuels. When combined with other energy sources, wind turbines can help balance power generation across different conditions.
2.3 Combined Heat and Power (CHP) Systems
- How They Work:
- Combined heat and power (CHP) systems, also known as cogeneration, simultaneously generate electricity and useful heat from the same fuel source (e.g., natural gas, biomass, or waste heat). This dual output improves overall energy efficiency, as it reduces the need for separate heating and electricity systems.
- CHP systems are particularly effective in industrial facilities, hospitals, and universities, where there is a constant demand for both power and heat.
- Applications:
- Suitable for industrial sites, commercial buildings, and residential complexes that require reliable energy and heating. CHP systems can also be part of microgrids, enhancing efficiency and resilience.
- Advantages:
- Higher efficiency (up to 80% or more), reduced energy costs, and lower carbon emissions compared to separate heat and power systems. CHP systems can operate on various fuels, including natural gas, biogas, and even hydrogen.
2.4 Small-Scale Gas Turbines and Diesel Generators
- How They Work:
- Small gas turbines and diesel generators are compact power generation units that use the combustion of fuel (natural gas, diesel, or biofuel) to produce electricity. These systems are often used as backup power sources or in locations where renewable options are insufficient.
- Diesel generators are widely used for emergency power supply and are known for their reliability, although they have higher emissions compared to gas turbines.
- Applications:
- Industrial facilities, hospitals, data centers, and remote areas where reliable power is critical. Gas turbines and diesel generators can also serve as backup power sources for microgrids.
- Advantages:
- Reliable and flexible, can be deployed quickly, and provide immediate power on demand. However, they tend to have higher operating costs and emissions compared to renewable options.
2.5 Biomass and Biogas Systems
- How They Work:
- Biomass systems burn organic materials (such as wood chips, agricultural waste, and dedicated energy crops) to produce heat, which can be used to generate electricity. Biogas systems use the anaerobic digestion of organic matter (e.g., food waste, manure) to produce methane, which can be burned for power generation.
- These systems are particularly useful in rural areas where biomass resources are abundant and can be integrated into local waste management strategies.
- Applications:
- Ideal for farms, rural communities, and industries that produce organic waste. Biomass and biogas systems can also provide combined heat and power, increasing overall efficiency.
- Advantages:
- Renewable and can help manage waste, reducing landfill use and emissions. Biomass and biogas systems can operate continuously, providing stable and reliable power.
3. Benefits of Decentralized and Small-Scale Power Generation
Decentralized power generation offers several benefits, making it an attractive option for many communities and industries.
3.1 Improved Efficiency and Reduced Transmission Losses
- Decentralized systems generate electricity close to where it is consumed, minimizing the energy lost during transmission over long distances. This improves the overall efficiency of the energy system.
- By reducing reliance on the central grid, decentralized systems can help reduce the need for extensive transmission infrastructure, lowering costs and environmental impact.
3.2 Increased Energy Security and Reliability
- Decentralized systems can enhance energy security by diversifying the energy supply. In case of disruptions to the main power grid, local power generation can continue to provide electricity, reducing the impact of outages.
- Microgrids and off-grid systems can also operate independently, ensuring that critical facilities (e.g., hospitals, data centers, emergency shelters) have a continuous power supply even during emergencies.
3.3 Support for Renewable Energy Integration
- Decentralized power systems make it easier to integrate renewable energy sources, such as solar, wind, and biomass, into the energy mix. These systems can be customized to take advantage of local resources, promoting sustainability and reducing carbon emissions.
- By using technologies like battery storage, decentralized systems can store excess renewable energy for use during periods of low generation, further stabilizing the grid.
3.4 Economic Benefits and Local Development
- Decentralized power generation can stimulate local economies by creating jobs in the installation, maintenance, and operation of small-scale energy systems. Local businesses can also benefit from reduced energy costs.
- In rural and remote areas, decentralized systems provide access to electricity, supporting development initiatives and improving the quality of life.
4. Challenges of Decentralized Power Generation
While decentralized and small-scale power generation offers many benefits, there are also challenges to consider:
4.1 Initial Capital Costs
- Setting up decentralized systems can require significant upfront investment, particularly for technologies like solar PV systems, wind turbines, and CHP units. However, falling costs of renewable technologies and government incentives can help offset these expenses.
- Long-term savings from reduced energy bills and lower transmission costs can make decentralized systems economically viable over time.
4.2 Intermittency and Energy Storage
- Renewable energy sources like solar and wind are intermittent, meaning they do not produce electricity consistently throughout the day. This can lead to issues with reliability if not managed properly.
- Energy storage solutions, such as batteries, are essential for balancing supply and demand in decentralized systems, but they can add to the overall cost and complexity.
4.3 Regulatory and Policy Barriers
- In some regions, regulatory frameworks and policies are not yet adapted to support decentralized energy systems. Issues such as grid interconnection, tariffs, and subsidies can hinder the development of local power generation projects.
- Effective policies are needed to encourage the adoption of decentralized power systems, promote renewable energy, and ensure fair compensation for distributed energy producers.
5. The Future of Decentralized and Small-Scale Power Generation
The future of energy is moving towards a more decentralized and resilient model. Innovations in technology, combined with growing concerns about climate change and energy security, are driving the adoption of small-scale power generation systems. Some key trends to watch include:
5.1 Integration of Smart Grids and IoT
- Smart grids use digital technology to monitor, control, and optimize the flow of electricity. When integrated with decentralized systems, smart grids can efficiently manage energy production and consumption, ensuring stability and reliability.
- The Internet of Things (IoT) enables devices to communicate and share data, which can be used to automate and optimize decentralized power systems. This can lead to better demand response, improved energy efficiency, and reduced costs.
5.2 Advances in Energy Storage Technology
- Energy storage is critical for overcoming the intermittency of renewable energy sources. Advances in battery technology, such as lithium-ion and solid-state batteries, are making it more feasible to store large amounts of energy for decentralized systems.
- Other storage solutions, like pumped hydro, flywheels, and hydrogen storage, are also being developed to enhance the flexibility and resilience of small-scale power generation.
5.3 Policy Support and Financial Incentives
- Governments worldwide are recognizing the benefits of decentralized energy and are introducing policies to encourage its growth. This includes tax incentives, grants, and subsidies for renewable energy installations and decentralized power projects.
- Financial innovations, such as community energy projects, crowdfunding, and green bonds, are also making it easier for individuals and businesses to invest in decentralized power systems.
Conclusion
Decentralized and small-scale power generation represents a shift towards a more sustainable, resilient, and efficient energy future. By generating electricity close to where it is consumed, these systems reduce transmission losses, enhance grid stability, and support the integration of renewable energy. Despite challenges related to cost, intermittency, and regulatory hurdles, the benefits of decentralized systems are driving their adoption across the globe. As technology continues to advance and policies evolve, decentralized power generation will play an increasingly important role in the global energy landscape, supporting a cleaner and more equitable energy system for all.
Industrial Applications of Decentralized and Small-Scale Power Generation
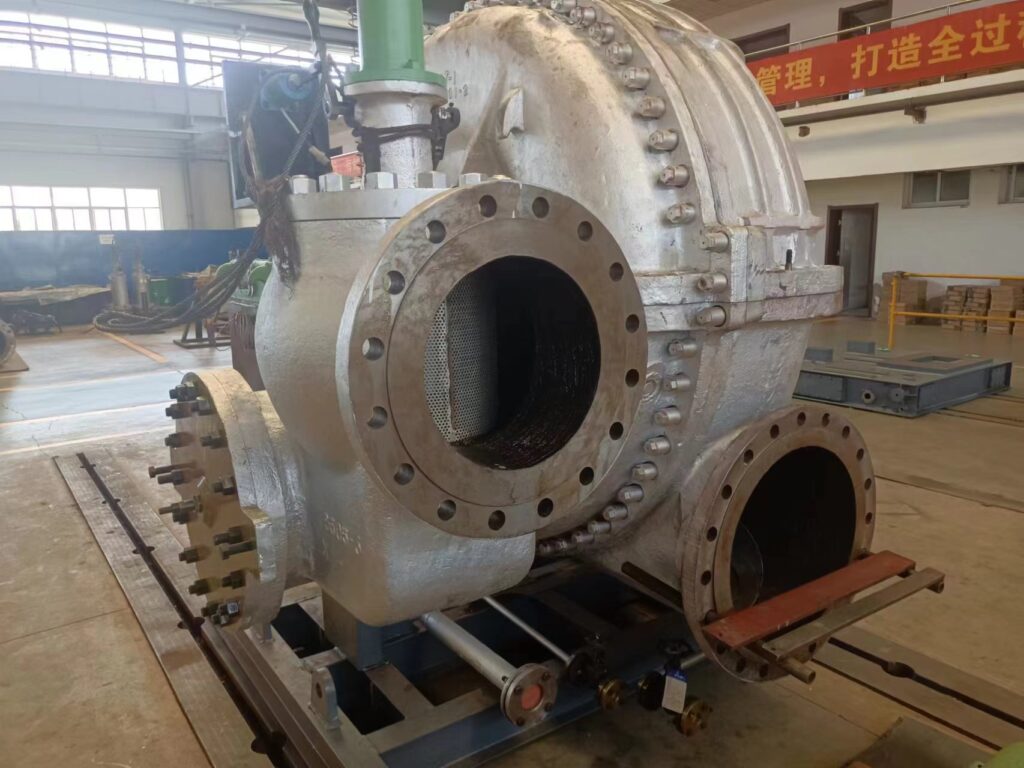
Decentralized and small-scale power generation systems have found numerous applications in industrial settings, offering benefits such as improved efficiency, cost savings, energy security, and reduced environmental impact. These systems generate electricity close to where it is needed, minimizing transmission losses and providing consistent, reliable power, even in areas where access to a stable central grid may be limited. Industrial applications of decentralized power generation range from powering manufacturing facilities to supporting specialized processes in refineries, chemical plants, and other heavy industries.
This section explores the various industrial applications of decentralized power generation, highlighting the technologies involved, benefits, challenges, and future trends.
1. Combined Heat and Power (CHP) Systems
1.1 Overview of CHP Systems
- How CHP Works:
- Combined Heat and Power (CHP) systems, also known as cogeneration, simultaneously produce electricity and useful heat from a single energy source, such as natural gas, biomass, or waste heat. In traditional power generation, a significant portion of energy is lost as heat, but CHP systems capture this heat and use it for industrial processes, heating, or cooling, leading to overall energy efficiencies of up to 80% or more.
- CHP systems are often installed on-site in industrial facilities, providing a reliable and efficient power source that reduces dependence on the central grid.
1.2 Industrial Applications
- Manufacturing Facilities:
- Many manufacturing plants require both electricity and heat for various processes, such as metal forging, food processing, and chemical manufacturing. CHP systems provide an efficient solution by generating electricity and using the waste heat for processes like drying, heating, and sterilization.
- For example, food processing facilities can use the waste heat from CHP systems for cooking and pasteurization, while textile manufacturers can use it for dyeing and drying.
- Chemical and Petrochemical Plants:
- Chemical production facilities, including refineries, often need significant amounts of heat for distillation, chemical reactions, and other processes. By integrating CHP systems, these plants can generate the electricity they need while utilizing the waste heat for process heating, improving overall energy efficiency.
- For instance, refineries can use CHP systems to power equipment while using the waste heat for distilling crude oil into various petroleum products.
- Paper and Pulp Industry:
- The paper and pulp industry is energy-intensive and requires large quantities of heat for drying and processing pulp into paper. CHP systems enable these plants to use one energy source to generate both electricity and steam, reducing fuel consumption and energy costs.
1.3 Benefits and Challenges
- Benefits:
- Higher energy efficiency, reduced energy costs, and lower carbon emissions compared to separate heat and power systems.
- Increased energy reliability and security, as industries are less dependent on the central power grid, reducing the risk of production downtime.
- Challenges:
- High initial capital costs for installing CHP systems, especially for smaller facilities.
- Requires continuous demand for both electricity and heat to maximize efficiency, making it less suitable for industries with highly variable heat needs.
2. On-Site Renewable Energy Systems
2.1 Solar Photovoltaic (PV) Systems
- How Solar PV Works:
- Solar photovoltaic (PV) systems convert sunlight directly into electricity using solar panels. These systems can be installed on rooftops, on the ground, or as part of a solar canopy in parking areas, providing a clean and renewable energy source for industrial facilities.
- Solar PV systems can be integrated into microgrids or operate as stand-alone systems, providing power during daylight hours and reducing the facility’s reliance on the central grid.
2.2 Industrial Applications
- Warehouses and Distribution Centers:
- Warehouses and distribution centers with large, flat rooftops are ideal for installing solar PV panels. These systems can generate significant amounts of electricity during the day, offsetting energy consumption and reducing costs.
- Large e-commerce and logistics companies are increasingly adopting solar power to improve sustainability and lower operational expenses.
- Manufacturing Plants:
- Solar PV systems can be used to supplement the electricity needs of manufacturing plants. For example, factories can use solar power to run machinery during the day or charge battery storage systems that provide backup power.
- In some cases, solar PV systems are combined with battery storage to ensure a stable energy supply, even when the sun is not shining.
2.3 Wind Power Systems
- Small-Scale Wind Turbines:
- Industrial facilities located in regions with consistent wind patterns can install small wind turbines to generate on-site electricity. These systems are especially effective in coastal or rural areas with open spaces and minimal obstructions.
- Wind turbines can be used in combination with other renewable systems, such as solar PV, to create hybrid power solutions that enhance energy reliability.
2.4 Benefits and Challenges
- Benefits:
- Renewable energy systems like solar PV and wind power reduce carbon emissions and contribute to sustainability goals. They also provide energy independence, allowing industries to control their energy production and costs.
- Excess electricity generated by renewable systems can be fed back into the grid, providing additional revenue streams through net metering.
- Challenges:
- Intermittency and variability of renewable energy sources can make it challenging to ensure a consistent power supply without adequate energy storage solutions.
- Initial installation costs for renewable systems can be high, although declining prices and government incentives are making these technologies more accessible.
3. Industrial Energy Storage Solutions
3.1 The Role of Energy Storage in Decentralized Systems
- Battery Energy Storage Systems (BESS):
- Battery storage is a crucial component of decentralized power systems, allowing industries to store excess energy generated from on-site renewables and use it when needed. This improves energy reliability and helps balance supply and demand.
- Lithium-ion batteries are the most common type of industrial energy storage, offering high energy density and rapid response times.
- Thermal Energy Storage:
- Thermal energy storage involves capturing and storing heat or cold energy for later use. This is useful for industrial processes that require heating or cooling, allowing facilities to use stored energy during peak demand periods.
- For example, surplus heat from a CHP system can be stored in thermal storage tanks and used during periods of high demand or when the primary heat source is not available.
3.2 Applications in Industrial Settings
- Peak Shaving and Load Management:
- Energy storage can help industries manage peak demand charges by storing energy during off-peak hours and using it during peak times. This practice, known as peak shaving, reduces electricity costs and eases the load on the main grid.
- Factories and data centers can use battery storage to maintain operations during peak hours without drawing additional power from the grid, thus avoiding expensive demand charges.
- Backup Power Supply:
- Energy storage systems provide backup power in case of grid outages, ensuring continuous operation for critical industrial processes. This is particularly important for industries that require uninterrupted power, such as chemical manufacturing, pharmaceuticals, and data centers.
- Batteries can quickly switch from charging to discharging mode, providing an immediate power supply during an emergency.
4. Industrial Microgrids
4.1 Overview of Microgrids in Industrial Applications
- How Microgrids Work:
- A microgrid is a localized energy system that can operate independently or in conjunction with the main power grid. It can include a mix of energy sources, such as solar panels, wind turbines, CHP units, and battery storage, to ensure a reliable power supply.
- Industrial microgrids offer enhanced energy security and resilience, particularly for facilities in regions prone to grid outages or with unreliable power infrastructure.
4.2 Applications and Benefits
- Resilience for Critical Operations:
- Industrial microgrids provide a reliable power supply for critical operations that cannot afford downtime, such as hospitals, data centers, and food processing plants. During a grid outage, the microgrid can disconnect from the main grid and continue to operate independently.
- This resilience ensures that essential services remain operational, even during extreme weather events or other emergencies that disrupt the central grid.
- Optimized Energy Management:
- Microgrids allow industries to optimize energy use by integrating various generation sources and storage solutions. They can automatically switch between grid power, on-site generation, and stored energy based on real-time demand and cost considerations.
- This flexibility helps industries reduce energy costs, manage peak demand, and achieve sustainability goals.
5. Benefits of Decentralized Power Generation for Industries
5.1 Cost Savings and Energy Efficiency
- Decentralized power generation can lead to significant cost savings by reducing reliance on the central grid and lowering peak demand charges. On-site generation, especially when using CHP or renewable systems, often results in lower operational costs.
- The efficiency of decentralized systems, such as CHP, can be as high as 80% compared to 30-40% for traditional power generation, reducing fuel consumption and emissions.
5.2 Enhanced Energy Security and Reliability
- On-site generation ensures that industries have a consistent and reliable power supply, even during grid outages. This is critical for facilities that require uninterrupted power to maintain safety and productivity.
- Microgrids enhance resilience by enabling facilities to operate independently, providing backup power, and integrating renewables for a stable energy supply.
5.3 Sustainability and Reduced Environmental Impact
- Decentralized systems support sustainability by enabling the use of renewable energy sources, such as solar, wind, and biomass. This reduces greenhouse gas emissions and contributes to climate goals.
- Efficient systems like CHP also reduce the overall carbon footprint by making better use of fuel resources, minimizing waste, and cutting down on emissions associated with power generation.
6. Challenges and Future Trends
6.1 Challenges
- High Initial Investment:
- Setting up decentralized power systems, particularly those involving advanced technologies like microgrids and CHP, can require significant capital investment. However, long-term savings and government incentives can offset these costs.
- Regulatory Barriers:
- In some regions, regulatory frameworks and policies may not be fully supportive of decentralized energy solutions. Issues such as grid interconnection, tariffs, and net metering policies can impact the deployment of these systems.
- Integration and Complexity:
- Managing multiple energy sources and storage systems in a microgrid requires sophisticated control systems. Integrating these components into a seamless and efficient network can be technically challenging.
6.2 Future Trends
- Digitalization and Smart Systems:
- The integration of digital technologies, such as smart sensors, IoT, and artificial intelligence, is making decentralized power systems more efficient and easier to manage. These technologies enable real-time monitoring, predictive maintenance, and automated energy management.
- Hybrid Energy Solutions:
- The future of industrial power generation is likely to involve hybrid systems that combine multiple energy sources, such as solar, wind, and CHP, to optimize energy production and consumption. This will enhance reliability, sustainability, and cost-effectiveness.
- Advances in Energy Storage:
- As energy storage technology continues to improve, industries will be better able to store excess renewable energy and use it when needed, making decentralized systems more reliable and resilient.
Conclusion
Decentralized and small-scale power generation offers significant advantages for industrial applications, including cost savings, enhanced energy security, and sustainability. By generating electricity close to the point of use, industries can optimize energy efficiency, reduce dependence on the central grid, and integrate renewable energy sources into their operations. Although challenges remain, advances in technology, supportive policies, and the growing demand for sustainable energy solutions are driving the adoption of decentralized power systems across various industries. As the world moves towards a more resilient and sustainable energy future, decentralized power generation will play an essential role in transforming how industries produce and consume energy.
EMS Power Machines
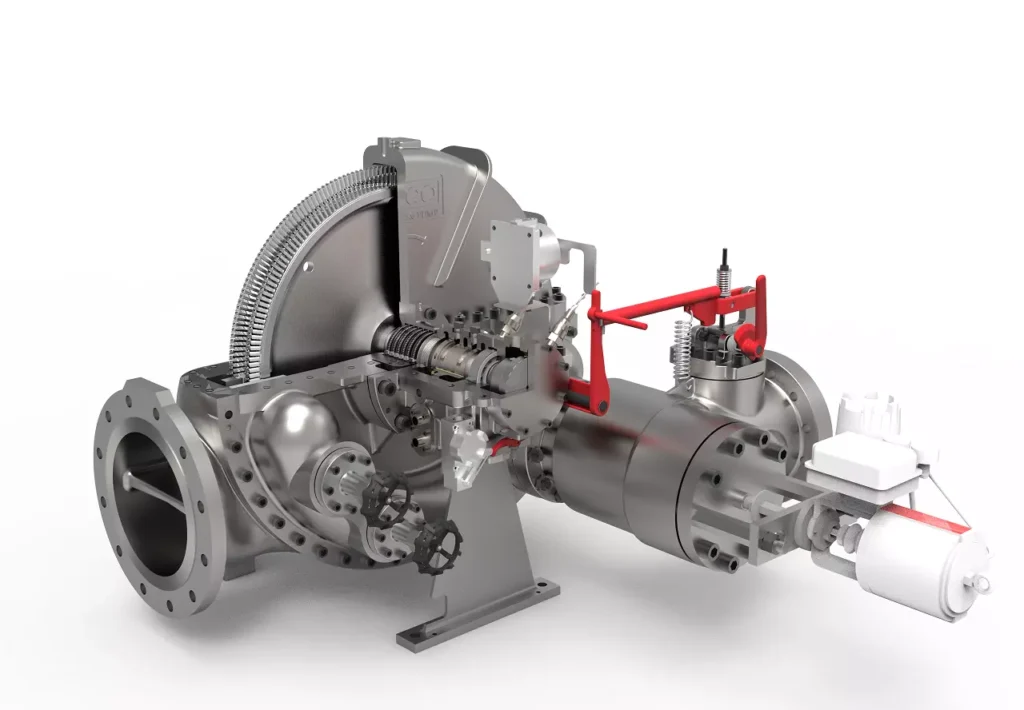
We design, manufacture and assembly Power Machines such as – diesel generators, electric motors, vibration motors, pumps, steam engines and steam turbines
EMS Power Machines is a global power engineering company, one of the five world leaders in the industry in terms of installed equipment. The companies included in the company have been operating in the energy market for more than 60 years.
EMS Power Machines manufactures steam turbines, gas turbines, hydroelectric turbines, generators, and other power equipment for thermal, nuclear, and hydroelectric power plants, as well as for various industries, transport, and marine energy.
EMS Power Machines is a major player in the global power industry, and its equipment is used in power plants all over the world. The company has a strong track record of innovation, and it is constantly developing new and improved technologies.
Here are some examples of Power Machines’ products and services:
- Steam turbines for thermal and nuclear power plants
- Gas turbines for combined cycle power plants and industrial applications
- Hydroelectric turbines for hydroelectric power plants
- Generators for all types of power plants
- Boilers for thermal power plants
- Condensers for thermal power plants
- Reheaters for thermal power plants
- Air preheaters for thermal power plants
- Feedwater pumps for thermal power plants
- Control systems for power plants
- Maintenance and repair services for power plants
EMS Power Machines is committed to providing its customers with high-quality products and services. The company has a strong reputation for reliability and innovation. Power Machines is a leading provider of power equipment and services, and it plays a vital role in the global power industry.
EMS Power Machines, which began in 1961 as a small factory of electric motors, has become a leading global supplier of electronic products for different segments. The search for excellence has resulted in the diversification of the business, adding to the electric motors products which provide from power generation to more efficient means of use.