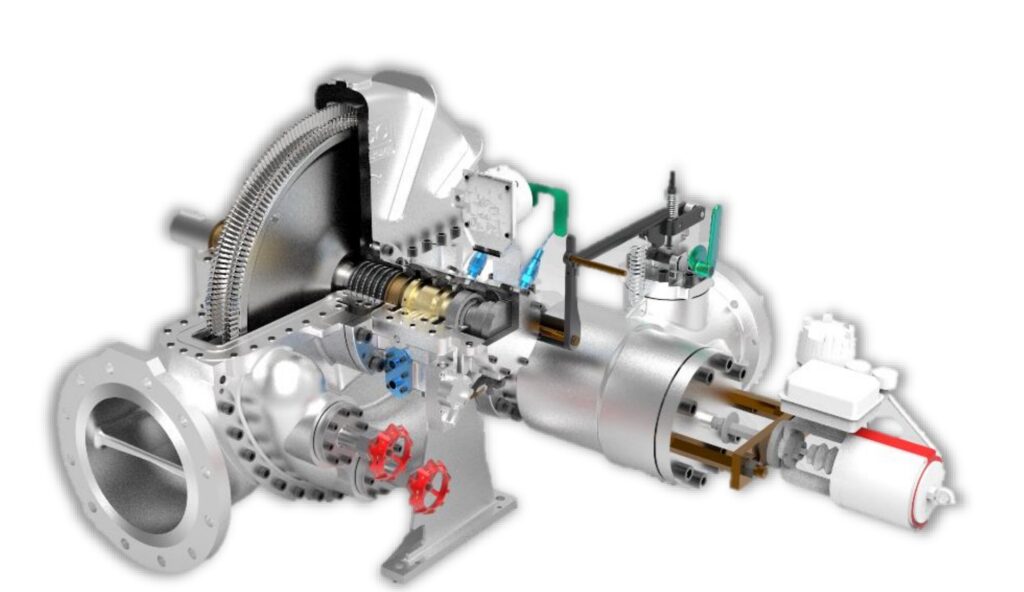
Extraction Back-pressure Turbines: Steam turbines are mechanical devices that convert the thermal energy of steam into mechanical work. They play a crucial role in power generation and industrial processes, operating based on the principles of thermodynamics and fluid dynamics. Steam turbines are widely used in power plants, ships, and industrial facilities due to their high efficiency, reliability, and ability to handle large power outputs. The working principle of a steam turbine involves steam generated in a boiler or heat source, which is then directed into the turbine. The high-pressure, high-temperature steam expands through a series of stationary and rotating blades arranged in stages. As the steam expands, it transfers its energy to the blades, causing the rotor to spin and generate mechanical power. This mechanical power can be used to drive a generator, producing electricity, or to power other machinery.
There are two main types of steam turbines: impulse and reaction turbines. Impulse turbines use high-velocity steam jets directed onto turbine blades to produce rotary motion. The kinetic energy of the steam is converted into mechanical energy primarily through the impulse force. In contrast, reaction turbines utilize both the pressure and velocity of the steam to generate motion. Here, the steam flows continuously through both stationary and moving blades, with energy conversion occurring due to the reaction force generated as the steam accelerates through the moving blades. Reaction turbines are generally used for applications requiring higher efficiency and larger power outputs, while impulse turbines are more suitable for smaller, simpler installations.
Steam turbines are classified further based on their operating conditions and applications. Condensing turbines are used in power generation to maximize efficiency by exhausting the steam into a condenser at sub-atmospheric pressure. This process allows for greater energy extraction from the steam. Back-pressure turbines, on the other hand, exhaust steam at a pressure suitable for downstream processes, such as heating or industrial applications. These turbines are often found in cogeneration systems where both power and process steam are required. Extraction turbines are a hybrid design that allows for controlled steam extraction at intermediate pressures for industrial processes while the remaining steam continues through the turbine for power generation. This flexibility makes them valuable in combined heat and power (CHP) systems.
Efficiency is a critical factor in steam turbine operation. Modern turbines are designed to operate at high efficiencies, often exceeding 40% in large power plants. Advanced materials, improved blade designs, and better thermal insulation contribute to these high efficiencies. However, steam turbines are still subject to losses due to heat transfer, friction, and leakage. Maintaining optimal operating conditions, such as proper steam temperature and pressure, is essential for achieving maximum efficiency. Additionally, regular maintenance and monitoring are necessary to ensure reliable and efficient operation over the turbine’s lifespan.
Steam turbines have significant advantages over other types of prime movers. They are capable of handling large power outputs and operate smoothly with minimal vibrations. They are also highly reliable and can achieve high thermal efficiencies, especially when combined with modern boiler technologies. Despite these advantages, steam turbines also have limitations. They require a significant initial investment and are best suited for applications with continuous and stable power demands. Furthermore, the operation of steam turbines relies on a consistent supply of high-quality steam, necessitating well-designed and maintained boilers or heat sources.
In recent years, advancements in materials science and manufacturing techniques have led to the development of more efficient and durable steam turbines. The use of advanced alloys and coatings has improved resistance to high temperatures and corrosion, while computational fluid dynamics (CFD) has enhanced blade design for better performance. Additionally, the integration of steam turbines with renewable energy sources, such as biomass and solar thermal systems, has expanded their role in sustainable energy solutions. These innovations continue to enhance the efficiency, reliability, and versatility of steam turbines, ensuring their continued relevance in modern energy systems.
The role of steam turbines in power generation and industrial processes cannot be overstated. They form the backbone of large-scale electricity production, particularly in fossil fuel, nuclear, and some renewable energy plants. In a conventional thermal power plant, steam turbines are part of a broader Rankine cycle. Heat energy is generated by burning fuel or through nuclear reactions, and this heat is used to produce steam at high pressures and temperatures. The steam is then expanded through the turbine, converting its thermal energy into mechanical work. After expansion, the low-pressure steam is condensed back into water in a condenser, which is then recirculated to the boiler to complete the cycle. This closed-loop system ensures the efficient use of water and heat, while minimizing energy losses.
In addition to their application in electricity generation, steam turbines are extensively used in industrial settings for process steam and mechanical power. Industries such as petrochemical, pulp and paper, food processing, and textiles rely on steam turbines to meet their heating, cooling, and mechanical energy needs. In many of these applications, back-pressure turbines are employed, as they allow for the simultaneous generation of power and steam at suitable conditions for industrial processes. This combined heat and power (CHP) approach is highly efficient, as it utilizes the thermal energy of steam more effectively than generating electricity and process steam separately. For example, in a paper mill, steam turbines not only generate electricity but also provide steam for drying paper, ensuring efficient energy utilization.
The operation of a steam turbine is influenced by a variety of factors, including steam quality, load conditions, and environmental factors. High-quality steam, free of impurities and moisture, is essential to avoid damage to turbine blades and other components. Impurities in steam can cause erosion, scaling, and corrosion, which reduce efficiency and increase maintenance costs. Similarly, fluctuations in load demand can impact the turbine’s performance and efficiency. Steam turbines are typically designed to operate at specific conditions for maximum efficiency, and deviations from these conditions can lead to performance losses. As a result, sophisticated control systems are often used to optimize turbine operation, ensuring that steam flow, pressure, and temperature remain within the desired range.
Environmental considerations also play a significant role in steam turbine operation. The disposal of condenser cooling water, emissions from boilers, and overall plant efficiency are closely monitored to minimize environmental impact. Many modern power plants incorporate advanced technologies, such as air-cooled condensers and low-emission boilers, to address these concerns. Additionally, the integration of carbon capture and storage (CCS) technologies in some power plants aims to reduce greenhouse gas emissions, ensuring compliance with environmental regulations and sustainability goals.
Steam turbine technology has evolved significantly over the years, driven by the demand for greater efficiency, reliability, and sustainability. Advanced turbine designs now include features such as multi-stage configurations, reheat cycles, and supercritical and ultra-supercritical operating conditions. These innovations enable turbines to extract more energy from steam while operating at higher temperatures and pressures. Supercritical and ultra-supercritical turbines, for instance, operate above the critical point of water, where steam behaves as a single-phase fluid. This eliminates phase change losses and improves overall cycle efficiency. Such advancements are critical for reducing fuel consumption and emissions in power plants, making them more environmentally friendly.
Maintenance and monitoring are critical aspects of steam turbine operation. Regular inspections, lubrication, and component replacements are necessary to ensure reliable performance and prevent unexpected failures. Advanced monitoring systems, often employing sensors and data analytics, provide real-time insights into turbine performance, allowing operators to detect anomalies and take corrective actions before they escalate. Condition-based maintenance strategies, enabled by these technologies, help reduce downtime and maintenance costs, further improving the economic viability of steam turbines.
The future of steam turbines is likely to be shaped by emerging trends and innovations in energy and technology. As the world transitions toward cleaner energy sources, steam turbines are being adapted to work with renewable energy systems, such as geothermal, solar thermal, and biomass power plants. Hybrid systems that combine renewable and conventional energy sources also leverage steam turbines for efficient energy conversion. Furthermore, the development of small modular reactors (SMRs) in the nuclear sector presents new opportunities for steam turbines in compact, scalable power generation systems. These developments highlight the adaptability and enduring relevance of steam turbine technology in an ever-changing energy landscape.
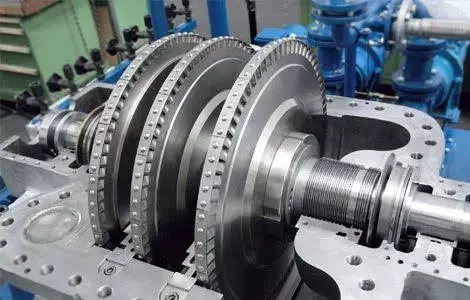
Steam turbines are also gaining importance in the context of energy efficiency and sustainability. The shift towards decarbonization has led to a greater focus on optimizing existing energy systems and integrating steam turbines into combined cycle and cogeneration systems. In a combined cycle configuration, steam turbines operate alongside gas turbines, using the waste heat from gas turbine exhaust to generate additional power. This configuration significantly enhances the overall efficiency of the power plant, often exceeding 60%. By utilizing what would otherwise be wasted energy, combined cycle systems not only reduce fuel consumption but also lower greenhouse gas emissions, making them a key component in modern power generation.
The use of steam turbines in renewable energy systems, such as geothermal and solar thermal power plants, demonstrates their versatility and adaptability. Geothermal power plants exploit heat from the Earth’s interior to produce steam, which drives the turbine. These systems are particularly advantageous because they provide reliable, baseload power with minimal emissions. Similarly, solar thermal power plants use concentrated solar energy to heat a working fluid, producing steam for turbines. In both cases, the ability of steam turbines to handle a wide range of steam conditions and their scalability make them well-suited for these applications.
Beyond power generation, steam turbines play a vital role in industrial energy recovery. Many industrial processes generate large amounts of waste heat, which can be captured and used to produce steam for turbines. This practice, known as waste heat recovery, improves the overall efficiency of industrial operations and reduces energy costs. For example, in a steel plant, the hot exhaust gases from furnaces can be used to generate steam, which is then fed into a turbine to produce electricity or drive equipment. Such applications not only enhance energy efficiency but also contribute to sustainability by reducing the environmental footprint of industrial activities.
The design and materials used in steam turbines are critical to their performance, especially in demanding environments. High-temperature and high-pressure conditions necessitate the use of advanced materials that can withstand extreme thermal and mechanical stresses. Alloys with high creep resistance and corrosion resistance are commonly used for turbine blades and rotors. Additionally, coatings are often applied to enhance durability and minimize wear and tear. These materials and coatings have been developed through extensive research and testing, ensuring that modern steam turbines can operate reliably for decades under challenging conditions.
Another significant area of development in steam turbine technology is the use of advanced digital tools and analytics. Digital twins, which are virtual replicas of physical turbines, are becoming increasingly popular for monitoring and optimizing turbine performance. These models use real-time data from sensors to simulate the turbine’s behavior under various operating conditions. By analyzing this data, operators can identify inefficiencies, predict maintenance needs, and optimize performance, all of which contribute to reducing downtime and operational costs. Artificial intelligence and machine learning algorithms further enhance these capabilities by identifying patterns and providing actionable insights that would be difficult to detect manually.
The growing interest in hydrogen as a clean energy source presents another potential avenue for steam turbines. Hydrogen can be burned in gas turbines, and the waste heat can be used to generate steam for steam turbines in a combined cycle setup. Alternatively, hydrogen can be used to produce steam directly for turbine applications. These approaches are particularly promising as hydrogen production becomes more cost-effective and widespread, supporting the global shift towards low-carbon energy systems.
Despite their many advantages, steam turbines face challenges that must be addressed to maintain their relevance in the evolving energy landscape. The high capital cost of turbine installations can be a barrier for some applications, particularly in smaller-scale or remote locations. Additionally, the efficiency of steam turbines is inherently limited by the Carnot cycle, and further improvements require innovations in thermodynamics, materials, and system integration. The need for skilled operators and maintenance personnel is another challenge, as the complexity of steam turbine systems demands specialized knowledge and training.
In summary, steam turbines are a cornerstone of modern energy systems, offering high efficiency, reliability, and versatility across a range of applications. From large-scale power generation to industrial energy recovery, their ability to convert thermal energy into mechanical work has made them indispensable. Advances in materials, design, and digital technologies continue to push the boundaries of what steam turbines can achieve, ensuring their role in both traditional and renewable energy systems. As the energy sector evolves to meet the demands of a sustainable future, steam turbines will remain a critical technology, adapting to new challenges and opportunities.
The adaptability of steam turbines in diverse energy applications underscores their enduring relevance in a rapidly changing world. One of the key advantages of steam turbines is their scalability, enabling them to cater to both large-scale power plants and smaller industrial facilities. For instance, in small-scale or decentralized energy systems, steam turbines can be integrated into microgrids to provide reliable electricity and heat for local communities or industrial complexes. These installations are particularly valuable in remote areas where access to centralized power grids is limited. By utilizing locally available fuels, such as biomass or waste products, these systems enhance energy security while reducing transportation costs and emissions associated with fuel supply chains.
In the marine industry, steam turbines have historically played a critical role in powering large vessels, particularly during the era of steamships. Today, while diesel engines dominate the marine sector, steam turbines are still used in specialized applications, such as nuclear-powered ships and submarines. In these contexts, the turbines provide unmatched reliability, efficiency, and the ability to operate for extended periods without refueling. Additionally, advancements in turbine technology have made them a viable option for liquefied natural gas (LNG) carriers, where steam turbines can utilize boil-off gas as a fuel source, further improving efficiency and reducing waste.
Steam turbines are also finding innovative applications in emerging technologies. For example, they are being integrated into energy storage systems, such as molten salt energy storage, where excess renewable energy is used to heat molten salt. The stored thermal energy can later be used to produce steam and drive a turbine, providing electricity during periods of high demand or low renewable energy production. This approach not only enhances grid stability but also maximizes the utilization of renewable energy resources. Similarly, advanced thermodynamic cycles, such as the Kalina cycle, are being explored to improve the efficiency of low-temperature heat sources. These cycles leverage the flexibility of steam turbines to operate with alternative working fluids, expanding their applicability to geothermal, solar thermal, and waste heat recovery systems.
Environmental sustainability is an increasingly important consideration in steam turbine operation and development. The focus on reducing carbon footprints and complying with stringent environmental regulations has driven the adoption of cleaner and more efficient technologies. Supercritical and ultra-supercritical steam turbines, for instance, operate at much higher temperatures and pressures than traditional systems, enabling greater energy extraction from the same amount of fuel. This reduces fuel consumption and emissions, making them an attractive option for coal-fired and natural gas power plants. Furthermore, carbon capture and storage (CCS) technologies are being integrated with steam turbine systems to capture CO₂ emissions before they are released into the atmosphere. This combination of high-efficiency turbines and CCS can significantly mitigate the environmental impact of fossil fuel-based power generation.
One of the most promising avenues for the future of steam turbines lies in hybrid energy systems. These systems combine multiple energy sources to optimize efficiency and reliability. For example, a hybrid system might integrate solar thermal power with a steam turbine and a gas turbine, leveraging the strengths of each technology. During periods of high solar availability, the solar thermal system can generate steam to drive the turbine, while the gas turbine can provide backup power during cloudy or nighttime conditions. Such systems not only improve overall energy efficiency but also enhance the flexibility and resilience of the power grid, making them well-suited for the transition to a low-carbon energy future.
The economic benefits of steam turbines are also noteworthy. While the initial capital costs of turbine installations can be high, their long operational lifespans and low maintenance requirements make them cost-effective over time. Advances in predictive maintenance and real-time monitoring have further reduced operational costs, enabling operators to detect potential issues early and address them proactively. Moreover, the high efficiency of steam turbines translates into lower fuel consumption, resulting in significant cost savings, particularly in energy-intensive industries. These economic advantages, combined with their technical versatility, make steam turbines an attractive investment for both traditional and renewable energy projects.
In conclusion, steam turbines are not only a cornerstone of traditional power generation but also a vital component of the energy systems of the future. Their ability to adapt to diverse applications, from large-scale power plants to decentralized energy systems, highlights their versatility and importance. With ongoing advancements in materials, design, and integration with renewable energy technologies, steam turbines are poised to play a central role in the global transition to sustainable energy. As the energy landscape continues to evolve, steam turbines will remain a key technology, bridging the gap between legacy systems and innovative solutions, and ensuring a reliable, efficient, and sustainable energy supply for generations to come.
Double Extraction Steam Turbines
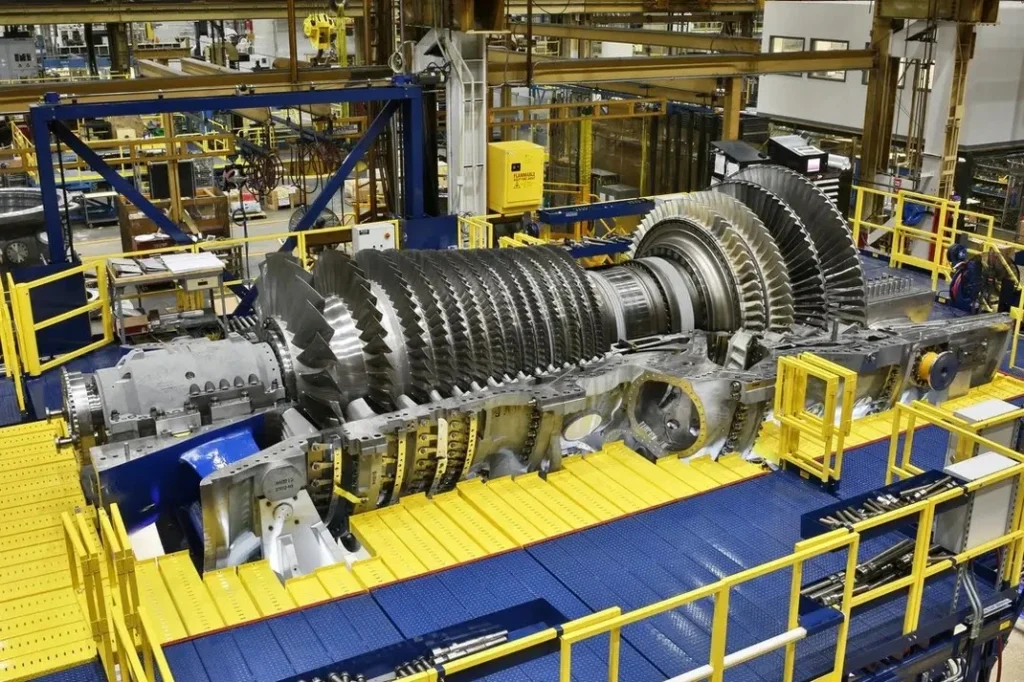
Double extraction steam turbines are specialized designs that allow for the controlled extraction of steam at two intermediate pressure levels during its expansion through the turbine. These turbines are particularly valuable in applications where both electricity generation and process steam are required, such as in combined heat and power (CHP) plants, industrial facilities, and chemical processing plants. By enabling steam to be extracted at multiple stages, double extraction turbines provide exceptional flexibility in meeting diverse energy and thermal demands.
The design of a double extraction steam turbine is more complex than a standard condensing or single extraction turbine. Steam enters the turbine at high pressure and temperature, where it begins its expansion process. As the steam passes through the turbine, a portion is extracted at the first intermediate pressure level to supply process requirements, such as heating or drying. The remaining steam continues to expand, and another portion is extracted at a second intermediate pressure level. The final stage of the turbine exhausts the residual steam, typically into a condenser for energy recovery or into a back-pressure system for further industrial use.
One of the primary advantages of double extraction turbines is their ability to optimize energy utilization in cogeneration systems. For example, in a large industrial plant, steam at different pressure levels may be required for various processes. Low-pressure steam might be used for space heating, medium-pressure steam for industrial chemical reactions, and high-pressure steam for mechanical work or electricity generation. A double extraction turbine can efficiently meet all these requirements by supplying steam at precisely the needed pressures and temperatures without the need for separate boilers or steam reducing stations.
Another key benefit is the ability to improve overall plant efficiency. By extracting steam at intermediate pressures for process use, double extraction turbines reduce the amount of steam that must be fully expanded to the condenser. This leads to better utilization of the steam’s thermal energy, minimizing waste and increasing the efficiency of the system as a whole. In cases where the turbine is part of a power plant, this configuration can enhance the plant’s overall thermal efficiency, particularly when process steam is used locally, reducing distribution losses.
Double extraction turbines are highly customizable and can be tailored to specific plant requirements. The pressure levels of the two extraction points, as well as the flow rates of extracted steam, are typically designed based on the process and power generation needs of the facility. Advanced control systems are often employed to regulate the extraction flow rates and ensure a stable supply of steam to the various processes. These control systems also help maintain the turbine’s efficiency and balance under varying load conditions, as process steam demands and power generation needs can fluctuate over time.
The applications of double extraction turbines extend across a wide range of industries. In chemical plants, for instance, different processes might require steam at varying pressure levels for heating, distillation, or reaction control. Similarly, in pulp and paper mills, steam at medium pressure might be used for paper drying, while low-pressure steam is used for space heating or other auxiliary needs. In power plants, double extraction turbines can be integrated with district heating systems, where they supply both electricity to the grid and steam for heating residential or commercial buildings.
However, the implementation of double extraction turbines comes with certain challenges. Their design and operation are more complex than single extraction or condensing turbines, requiring precise engineering and control. The initial capital cost is typically higher due to the additional components, such as extraction ports, piping, and control systems. Moreover, operators must ensure that the turbine operates within its designed parameters to avoid efficiency losses or mechanical issues. This requires robust monitoring systems and skilled personnel to manage the turbine’s operation effectively.
Despite these challenges, the long-term benefits of double extraction steam turbines often outweigh the initial costs. Their ability to enhance energy efficiency, reduce fuel consumption, and provide flexibility in meeting thermal and electrical demands makes them a valuable asset in both industrial and power generation contexts. Advances in materials and digital control systems have further improved their reliability and performance, ensuring that double extraction turbines remain a key technology in modern energy systems.
As industries and power plants continue to prioritize sustainability and energy efficiency, double extraction steam turbines are expected to play an increasingly important role. Their ability to integrate seamlessly into CHP systems and adapt to variable process demands makes them particularly well-suited for applications where maximizing energy utilization is critical. By effectively combining power generation and process steam supply, double extraction turbines contribute to a more sustainable and efficient energy future.
The design and operation of double extraction steam turbines involve several engineering considerations that ensure their performance, efficiency, and reliability. One critical aspect is the configuration of the turbine’s stages and extraction ports. The steam turbine must be designed to handle varying steam flow rates and pressures across its stages, which depend on the specific requirements of the extraction points. Each extraction port is strategically located at an intermediate stage of the turbine to achieve the desired steam pressure and temperature. The steam extracted from these ports is directed to industrial processes, heating systems, or other applications where it is needed. The remaining steam continues its expansion through the turbine, contributing to power generation or other uses.
Advanced control systems play a vital role in managing the operation of double extraction turbines. These systems regulate the flow of steam at the extraction points to ensure a stable and continuous supply of steam at the required pressure levels. This is particularly important in industrial plants, where steam demands can vary significantly depending on production schedules and operational conditions. Modern control systems utilize sensors, actuators, and real-time data analytics to monitor and adjust steam flows dynamically. This level of precision ensures that the turbine operates efficiently while meeting the varying thermal and electrical demands of the facility.
The integration of double extraction turbines into a larger energy system often requires detailed planning and coordination. For instance, in a cogeneration plant, the steam turbine is typically linked to a boiler or heat recovery steam generator (HRSG) that provides the high-pressure steam required for turbine operation. The extracted steam is then distributed to various process units or heating systems through a network of pipelines and heat exchangers. Ensuring that these components are properly sized, aligned, and optimized is essential for the efficient operation of the entire system. Additionally, provisions must be made to handle fluctuations in steam demand and supply, such as installing bypass systems or incorporating thermal storage solutions.
The thermodynamic efficiency of a double extraction steam turbine is influenced by the pressure and temperature conditions at the extraction points, as well as the quality of the steam entering the turbine. Higher inlet steam pressures and temperatures generally result in better overall efficiency, as they allow more energy to be extracted from the steam. However, the design must also account for the specific requirements of the extraction points, as extracting steam at too high or too low a pressure can lead to inefficiencies or process disruptions. Advanced modeling and simulation tools are often used during the design phase to optimize the turbine’s performance and ensure compatibility with the intended application.
Maintenance and reliability are also critical considerations for double extraction turbines. Regular inspections and preventive maintenance are necessary to ensure the long-term performance and durability of the turbine. Key components, such as the rotor, blades, and bearings, must be checked for signs of wear, erosion, or corrosion. Steam quality is another factor that directly impacts the turbine’s longevity; impurities, such as dissolved salts or particulates, can cause scaling or erosion of turbine components. Proper water treatment and steam conditioning are therefore essential to maintain the turbine’s reliability and efficiency.
One of the most significant advantages of double extraction turbines is their ability to adapt to a wide range of industrial and power generation scenarios. In a chemical plant, for example, the extracted steam may be used to drive chemical reactions in reactors, control temperatures in distillation columns, or provide heat for separation processes. In a sugar mill, the turbine can generate electricity while supplying steam for sugarcane juice evaporation and crystallization. In a district heating system, extracted steam can be distributed through an extensive network of insulated pipes to provide heating for residential and commercial buildings. These diverse applications demonstrate the versatility of double extraction turbines in addressing complex energy and thermal demands.
The economic and environmental benefits of double extraction turbines further enhance their appeal. By integrating power generation with process steam supply, these turbines maximize the utilization of fuel energy, reducing waste and lowering greenhouse gas emissions. This makes them an ideal choice for facilities seeking to improve energy efficiency and reduce their carbon footprint. In regions where energy costs are high or environmental regulations are stringent, the efficiency gains achieved with double extraction turbines can result in substantial cost savings and compliance benefits.
As industries continue to evolve, the role of double extraction steam turbines is expected to expand. Advances in materials science, turbine design, and digital technology are enabling the development of more efficient, durable, and adaptable turbines. For example, the use of advanced alloys and coatings can enhance the turbine’s resistance to high temperatures and corrosive environments, extending its operational life. Digital twins and predictive analytics are also being increasingly used to monitor turbine performance, identify potential issues, and optimize maintenance schedules. These innovations ensure that double extraction turbines remain at the forefront of energy-efficient and sustainable solutions in industrial and power generation applications.
Another critical factor in the adoption and continued innovation of double extraction steam turbines is their role in improving overall system reliability and energy security. By serving as a central component of cogeneration or combined heat and power (CHP) systems, these turbines allow facilities to operate independently of external electricity supplies in certain cases, enhancing resilience during grid outages or energy supply disruptions. This capability is particularly valuable for industries such as petrochemicals, pharmaceuticals, and food processing, where consistent power and steam supply are critical to maintaining production and product quality.
The flexibility of double extraction steam turbines also supports their use in facilities with highly variable energy and steam demands. For instance, in an industrial plant with seasonal production cycles or batch processing operations, steam demand may fluctuate widely over time. Double extraction turbines can handle these fluctuations efficiently by dynamically adjusting steam extraction rates and power output. This adaptability not only ensures that the facility’s energy and process steam needs are met but also prevents inefficiencies and potential losses associated with overproduction or underutilization of steam.
In terms of economic performance, the lifecycle cost benefits of double extraction turbines are particularly noteworthy. While these turbines may have higher initial capital costs due to their advanced design and additional extraction points, the long-term savings in fuel consumption and operating expenses often outweigh the upfront investment. This is because the turbines make better use of the thermal energy in the steam, reducing the amount of fuel required for both power generation and process heat. Additionally, integrating double extraction turbines into energy systems can lead to eligibility for government incentives, tax breaks, or carbon credits in jurisdictions that promote energy efficiency and low-carbon technologies.
The versatility of double extraction turbines also extends to their compatibility with a variety of fuels and energy sources. In traditional setups, high-pressure steam for the turbine is typically generated using fossil fuels such as natural gas, coal, or oil. However, double extraction turbines can also be integrated into systems using alternative and renewable fuels, such as biomass, municipal solid waste, or biogas. In a biomass power plant, for example, the combustion of wood chips, agricultural residues, or other organic materials generates steam for the turbine. The extracted steam can then be used for drying biomass feedstock or powering other industrial processes, creating a closed-loop system that minimizes waste and maximizes resource efficiency.
Moreover, double extraction turbines can play an important role in integrating renewable energy sources into industrial processes. In solar thermal power plants, for instance, steam generated from concentrated solar power (CSP) systems can be fed into a double extraction turbine. This setup enables simultaneous electricity generation and process steam supply, making it particularly valuable for industrial operations in regions with abundant sunlight. Similarly, in geothermal power plants, steam extracted from geothermal wells can be directed into a double extraction turbine to meet both power and heat requirements.
As global energy systems transition toward decarbonization, the potential for coupling double extraction turbines with emerging technologies, such as hydrogen and carbon capture, is becoming increasingly apparent. Hydrogen-fired boilers or gas turbines can produce high-pressure steam for double extraction turbines, enabling facilities to significantly reduce their carbon emissions. In carbon capture and storage (CCS) systems, steam extracted from intermediate stages of the turbine can be used to regenerate chemical solvents or drive compression equipment, further enhancing the system’s overall efficiency and environmental performance.
Advances in automation, digital monitoring, and data analytics are also transforming the operation of double extraction turbines. Modern turbines are equipped with extensive sensor networks that provide real-time data on parameters such as steam flow rates, temperatures, pressures, and vibration levels. This data is analyzed using predictive maintenance algorithms to identify early signs of wear, corrosion, or mechanical issues, allowing operators to address potential problems before they lead to costly downtime or damage. Digital twins—virtual models of the physical turbine—are increasingly being used to simulate turbine behavior under various scenarios, optimizing performance and maintenance strategies without interrupting operations.
From an engineering perspective, the materials and manufacturing techniques used in double extraction turbines continue to evolve to meet the demands of modern energy systems. Advanced materials, such as nickel-based superalloys and ceramic coatings, are being employed to improve the turbine’s resistance to high temperatures, corrosion, and mechanical stress. These innovations enable turbines to operate at higher temperatures and pressures, which directly translates to increased thermodynamic efficiency. Additive manufacturing techniques, such as 3D printing, are also being explored for producing complex turbine components, reducing lead times and enabling the customization of parts for specific applications.
In summary, double extraction steam turbines represent a highly efficient, flexible, and adaptable technology for meeting the diverse energy and steam demands of industrial and power generation facilities. Their ability to optimize energy use, support a wide range of fuels and applications, and integrate with emerging technologies positions them as a key component of sustainable energy systems. As innovations in materials, digital technologies, and renewable energy integration continue to advance, double extraction turbines are expected to play an even more significant role in shaping the future of energy efficiency and sustainability.
Looking toward the future, the role of double extraction steam turbines is set to expand as industries and power systems evolve to meet the demands of decarbonization, decentralization, and digitalization. One of the most significant drivers of this evolution is the global push to reduce greenhouse gas emissions and transition to a low-carbon energy economy. Double extraction turbines, with their ability to maximize energy efficiency and integrate seamlessly with renewable and low-carbon technologies, are uniquely positioned to support this transition.
For instance, as hydrogen emerges as a key component of clean energy systems, double extraction turbines could be utilized in hydrogen-based power and industrial systems. Hydrogen can be used as a fuel in boilers or combined cycle power plants to generate the high-pressure steam required for turbine operation. The extracted steam from these turbines could then be directed to hydrogen production processes, such as steam methane reforming (SMR) with carbon capture or advanced water electrolysis, creating a closed-loop system that minimizes energy losses and emissions. This synergy between hydrogen and steam turbines could be particularly valuable in hard-to-decarbonize sectors, such as steelmaking, chemicals, and heavy industry.
In addition to hydrogen, double extraction turbines are well-suited for integration into circular economy initiatives, where waste products are repurposed as valuable resources. For example, in waste-to-energy plants, municipal solid waste is incinerated to generate high-pressure steam for turbines. The extracted steam can then be used for district heating, industrial processes, or even desalination, while the remaining electricity can be supplied to the grid. Similarly, in biomass plants, agricultural residues, wood chips, or other organic materials are converted into steam, with the extraction points providing thermal energy for drying biomass or other pre-treatment steps.
The continued development of renewable energy technologies also presents opportunities for double extraction turbines to expand their applications. In hybrid renewable systems, these turbines can act as a bridge between intermittent energy sources like wind and solar and the stable energy requirements of industrial facilities. For example, during periods of high renewable energy production, excess electricity can be used to generate steam from renewable heat sources, such as solar thermal collectors or geothermal wells. The extracted steam can then supply thermal energy to industrial processes, reducing reliance on fossil fuels and enhancing overall energy efficiency.
The integration of advanced thermodynamic cycles offers another avenue for enhancing the performance of double extraction turbines. Concepts such as the Kalina cycle or Organic Rankine Cycle (ORC) are being explored to utilize low-grade heat sources, such as waste heat from industrial processes or geothermal fluids. These cycles often operate with working fluids that differ from water, but their principles can complement traditional double extraction turbines. For instance, a hybrid system could combine a double extraction steam turbine for high-temperature processes with an ORC system to recover low-temperature waste heat, maximizing the energy output from a given resource.
One of the most promising areas of innovation is the digital transformation of steam turbine technology. Advanced monitoring systems, powered by the Industrial Internet of Things (IIoT), allow operators to continuously monitor turbine performance and diagnose potential issues in real time. This data-driven approach enables predictive maintenance strategies, reducing unplanned downtime and extending the operational life of the turbine. Additionally, artificial intelligence and machine learning algorithms can be used to optimize turbine performance under varying load conditions, ensuring that the turbine operates at peak efficiency even as steam and power demands fluctuate.
Digital twins are increasingly being adopted in the design, operation, and maintenance of double extraction turbines. These virtual replicas of physical turbines allow engineers to simulate different operating scenarios, identify potential performance bottlenecks, and test design modifications before implementing them in the real world. In operation, digital twins can be used to optimize energy flows, predict wear and tear, and ensure that the turbine operates within its design limits, all of which contribute to improved reliability and efficiency.
From a policy and regulatory perspective, double extraction turbines are also benefiting from initiatives aimed at promoting energy efficiency and reducing carbon emissions. Many governments and organizations offer incentives, grants, or tax credits for facilities that implement high-efficiency cogeneration systems, including those utilizing double extraction turbines. These incentives help offset the higher initial capital costs of these turbines, making them a more attractive option for industrial and power generation applications. Additionally, the growing emphasis on carbon pricing and emissions trading systems further incentivizes the adoption of energy-efficient technologies like double extraction turbines.
In terms of infrastructure, the deployment of double extraction turbines often requires collaboration between multiple stakeholders, including equipment manufacturers, process engineers, and energy providers. This collaborative approach ensures that the turbine is integrated seamlessly into the broader energy and process systems of the facility. For instance, in a large industrial complex, the design and operation of the turbine must be coordinated with the steam distribution network, process equipment, and power generation systems to maximize efficiency and reliability.
The growing role of automation and robotics in manufacturing is also influencing the production and maintenance of double extraction turbines. Precision manufacturing techniques, such as additive manufacturing and advanced machining, are enabling the production of complex turbine components with greater accuracy and efficiency. These techniques reduce lead times and allow for the customization of turbine designs to meet specific application requirements. In maintenance, robotic systems are being developed to perform inspections, repairs, and component replacements in hard-to-access areas of the turbine, reducing downtime and improving worker safety.
In conclusion, double extraction steam turbines represent a versatile and forward-looking technology that is well-suited to meet the challenges and opportunities of the modern energy landscape. Their ability to optimize energy use, integrate with renewable and low-carbon systems, and adapt to a wide range of applications ensures their continued relevance in an era of rapid technological and environmental change. As innovations in materials, digital technology, and thermodynamic cycles continue to advance, double extraction turbines are poised to play an even greater role in driving energy efficiency, sustainability, and resilience in industrial and power generation systems around the world.
The future of double extraction steam turbines is increasingly tied to advancements in energy storage systems, hybrid energy systems, and decentralized energy generation. These trends reflect the growing need for energy systems that are both flexible and resilient in the face of shifting demands and the increasing penetration of renewable energy sources. By seamlessly integrating with these technologies, double extraction turbines are expected to expand their applications and redefine how energy is produced, distributed, and utilized.
One emerging area where double extraction turbines can play a pivotal role is in hybrid energy systems that combine traditional steam turbines with energy storage technologies, such as thermal energy storage (TES) or advanced battery systems. In such configurations, excess heat or power generated during periods of low demand can be stored for later use. For example, during off-peak hours, surplus steam could be stored in molten salt or phase-change materials within a thermal storage system. This stored energy can then be released during peak demand periods to provide additional process steam or boost power generation. By leveraging energy storage, double extraction turbines can enhance overall system efficiency, improve load balancing, and ensure a reliable supply of both electricity and thermal energy.
Additionally, the role of double extraction turbines in microgrid systems is gaining attention. Microgrids are localized energy systems that can operate independently or in conjunction with the main power grid. They are particularly useful in remote locations, industrial parks, and facilities where energy reliability is critical. Double extraction turbines can serve as a central component of a microgrid, providing both electricity and thermal energy to meet local demand. This is especially valuable in industrial microgrids, where the extracted steam can be used for manufacturing processes while the remaining energy is used to power local operations or exported to the grid. The integration of renewable energy sources, such as solar and wind, into these microgrids further enhances their sustainability and energy independence.
Decentralized energy systems are another growing trend where double extraction turbines can have significant applications. As energy production becomes increasingly distributed, facilities are seeking ways to generate power and heat locally rather than relying solely on centralized power plants. Double extraction turbines fit well into this model because of their ability to provide a reliable and efficient source of cogeneration. Industrial facilities, commercial buildings, and even large residential complexes can install these turbines to meet their specific energy and thermal needs while reducing transmission losses and enhancing energy security.
In addition to their compatibility with decentralized energy systems, double extraction turbines can support grid stabilization and demand response programs. As the share of variable renewable energy sources, such as wind and solar, increases in power grids, balancing supply and demand becomes more challenging. Double extraction turbines, especially when integrated with thermal energy storage or advanced control systems, can adjust their power and steam output dynamically to match grid requirements. For instance, during periods of high renewable energy generation, the turbine can focus on meeting process steam needs while reducing electricity output. Conversely, during low renewable energy generation, the turbine can ramp up electricity production to help stabilize the grid. This operational flexibility makes double extraction turbines a valuable asset in modern power systems that prioritize renewable integration.
The adoption of advanced materials and manufacturing techniques is expected to further enhance the performance and reliability of double extraction turbines. Research into high-temperature alloys, ceramics, and advanced coatings is enabling turbines to operate at higher temperatures and pressures, which translates directly into improved efficiency. These materials are particularly important for handling the harsh conditions associated with renewable fuels, such as biomass or waste-derived fuels, which can introduce additional corrosive or erosive challenges. Additive manufacturing, or 3D printing, is also being utilized to produce intricate turbine components with high precision and reduced lead times. This allows for greater customization and quicker responses to evolving energy demands.
Moreover, the focus on carbon neutrality and the circular economy has driven innovation in how steam is generated for double extraction turbines. Low-carbon and zero-carbon fuel options, such as renewable hydrogen, ammonia, or synthetic fuels, are increasingly being explored as alternatives to traditional fossil fuels. In these systems, double extraction turbines play a critical role in ensuring the efficient conversion of thermal energy into usable power and process steam. The use of such fuels, combined with the efficiency gains from double extraction, can significantly reduce the carbon footprint of industrial and power generation systems.
Another transformative development is the application of artificial intelligence (AI) and machine learning to optimize the operation and maintenance of double extraction turbines. AI algorithms can analyze real-time data from sensors embedded within the turbine to identify performance trends, predict maintenance needs, and optimize operation for maximum efficiency. For example, an AI-driven system might adjust extraction steam flow rates based on changing process demands or predict when turbine blades require inspection based on vibration analysis. This level of intelligence not only reduces downtime and maintenance costs but also ensures that the turbine operates at peak performance throughout its lifecycle.
Digital twins are particularly powerful in this context. By creating a virtual model of the turbine and its associated systems, operators can simulate various scenarios, such as changes in process steam demand, fluctuations in fuel supply, or grid outages. These simulations allow for proactive adjustments that enhance efficiency and reliability while minimizing risks. Digital twins also facilitate collaboration between engineers and operators, as they provide a shared platform for analyzing performance and planning improvements.
As global energy markets continue to prioritize sustainability, energy efficiency, and resilience, the versatility of double extraction turbines ensures they will remain an integral part of the energy landscape. Their ability to integrate with emerging technologies, adapt to decentralized and hybrid energy systems, and meet diverse industrial needs positions them as a cornerstone of modern energy solutions. Furthermore, ongoing research and development efforts are likely to unlock new capabilities and applications, ensuring that double extraction turbines continue to evolve in step with the changing demands of the energy industry.
In the coming decades, as industries embrace carbon-neutral practices and advanced energy systems, double extraction turbines are expected to play a central role in driving this transformation. Their unmatched flexibility, coupled with advancements in materials, digital technologies, and integration strategies, ensures their relevance and importance in achieving a more sustainable and efficient energy future.
Cogeneration
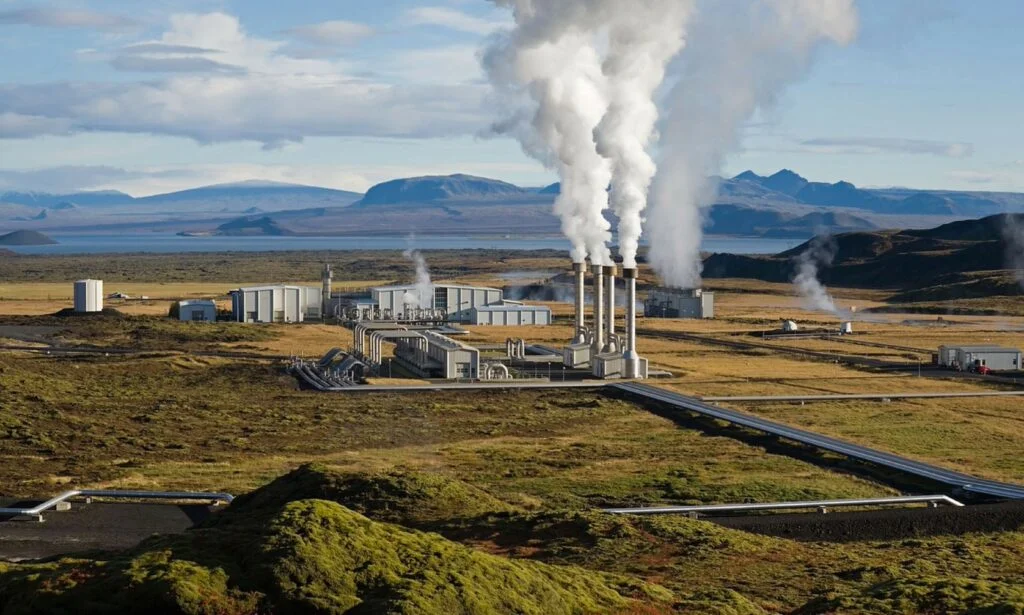
Cogeneration, also known as combined heat and power (CHP), is a highly efficient energy production process that simultaneously generates electricity and useful heat from a single energy source. Unlike conventional power generation methods, where energy is typically wasted in the form of excess heat, cogeneration captures and utilizes this heat for various industrial, residential, or commercial purposes. This process significantly improves the overall energy efficiency of a system, reduces fuel consumption, and lowers greenhouse gas emissions.
In a typical cogeneration system, a fuel source—such as natural gas, biomass, coal, or waste—is burned to produce heat, which drives a steam turbine or engine to generate electricity. The excess heat, which would otherwise be lost in traditional power plants, is captured and used for heating purposes, such as space heating, industrial processes, or water heating. This dual use of energy can result in overall efficiencies of 70% to 90%, compared to the 33% to 40% efficiency of conventional power plants. This makes cogeneration systems an attractive option for industries with both electricity and thermal energy demands, such as chemical manufacturing, food processing, paper mills, and refineries.
The key advantage of cogeneration is its ability to meet the combined demand for electricity and heat. This is particularly important for facilities that have a constant or variable need for both forms of energy. In industries such as food production, where precise temperature control is crucial, the ability to use waste heat for heating processes can lead to significant cost savings. In addition, cogeneration systems can be designed to scale with the needs of the facility, from small systems for individual buildings to large-scale industrial plants.
One of the most significant benefits of cogeneration is its contribution to energy efficiency and sustainability. By capturing and utilizing waste heat, cogeneration reduces the need for additional fuel consumption, leading to lower operating costs and a reduction in the environmental impact of energy production. This makes cogeneration a preferred option for industries aiming to improve energy efficiency, reduce their carbon footprint, and comply with increasingly stringent environmental regulations. The ability to use waste heat for applications such as district heating, steam supply, or absorption refrigeration also provides a significant advantage in terms of energy savings and emissions reductions.
In addition to its environmental and economic benefits, cogeneration offers energy security and reliability. In many cases, cogeneration systems can operate independently or in parallel with the main grid, providing backup power during grid outages or interruptions. This capability is particularly valuable for industries that require a consistent and reliable supply of energy for continuous operations. Cogeneration can also be integrated into microgrids or decentralized energy systems, where local energy generation and consumption are optimized to reduce dependence on external power sources.
The efficiency of cogeneration systems is further enhanced through the use of advanced technologies, such as combined cycle systems, which use both gas and steam turbines to generate electricity. In a combined cycle plant, exhaust gases from a gas turbine are directed to a steam boiler, where they generate additional steam to drive a steam turbine, thereby increasing the overall efficiency of the system. Additionally, advancements in digital control systems and monitoring technologies allow for the real-time optimization of energy production, further improving the performance and reliability of cogeneration plants.
Cogeneration is also gaining popularity as a key component of renewable energy integration. Biomass and waste-to-energy systems, which use organic materials to generate heat and power, are examples of renewable cogeneration systems that contribute to the decarbonization of the energy sector. These systems can be integrated with district heating networks to provide both electricity and heating to local communities, further reducing reliance on fossil fuels and enhancing sustainability.
Despite its many advantages, the implementation of cogeneration systems requires careful planning and investment. The initial capital cost of installing a cogeneration plant can be significant, particularly for larger-scale systems. However, this cost is often offset by long-term energy savings, reduced fuel consumption, and lower operating costs. Additionally, government incentives, tax credits, and environmental policies that support energy efficiency and emissions reductions can make cogeneration more financially attractive.
The success of a cogeneration system depends on various factors, including the type of fuel used, the scale of the operation, the efficiency of the turbines or engines, and the integration with existing infrastructure. Facilities considering cogeneration must conduct thorough feasibility studies to determine the best design and configuration for their specific energy needs. This may involve analyzing the demand for both electricity and heat, as well as evaluating the potential for utilizing waste heat in other applications. In some cases, cogeneration systems can be designed with multiple energy extraction points, such as in double extraction steam turbines, which allow for even more efficient use of available thermal energy.
Looking to the future, cogeneration is expected to continue playing a critical role in the global energy transition. As industries seek to reduce their carbon emissions and energy consumption, the demand for efficient and sustainable energy solutions like cogeneration will increase. The development of new technologies, such as hybrid systems that integrate renewable energy sources with cogeneration, will further expand the potential applications of cogeneration. For example, the combination of solar thermal energy and a cogeneration system can provide both electricity and heat with minimal environmental impact, making it an attractive solution for facilities seeking to meet sustainability goals.
The ability of cogeneration systems to integrate with various energy sources, including renewables, is one of the key reasons for their continued growth and importance in modern energy systems. As global energy markets shift toward decarbonization and greater sustainability, cogeneration will remain a cornerstone of efficient and reliable energy production, helping to meet both the current and future energy needs of industries, communities, and the world at large.
As cogeneration continues to evolve, the growing emphasis on smart energy management and digital technologies is further enhancing its efficiency and performance. The integration of advanced sensors, data analytics, and machine learning algorithms enables real-time monitoring of cogeneration systems, providing valuable insights into operational performance, energy consumption patterns, and equipment health. This information allows operators to optimize energy flows, adjust output based on demand, and predict maintenance needs, reducing downtime and extending the lifespan of the equipment.
The implementation of smart grids and automated energy management systems (EMS) is also improving the integration of cogeneration into larger energy networks. These systems can dynamically balance energy supply and demand across multiple sources, ensuring that electricity and heat are distributed efficiently to end-users. For example, in a microgrid setting, a cogeneration system can be controlled to meet local energy demand while interacting with renewable energy sources, such as solar and wind, to ensure grid stability and reliability. By integrating energy storage systems, such as batteries or thermal storage, cogeneration plants can further enhance their ability to store excess energy produced during periods of low demand and release it during peak times, minimizing energy waste and improving overall grid resilience.
The use of artificial intelligence (AI) and predictive analytics in cogeneration systems also enables the optimization of fuel use, combustion processes, and steam extraction. AI-powered algorithms can analyze historical data and make real-time adjustments to the system, ensuring that the cogeneration process operates at its peak efficiency. For instance, AI can optimize the combustion process to reduce emissions and improve fuel-to-energy conversion ratios, while simultaneously adjusting steam extraction points to meet varying demand for electricity and thermal energy. By continuously learning from the system’s performance, AI can predict future operational conditions and recommend adjustments that maximize energy savings and minimize operational costs.
As environmental concerns and sustainability goals continue to drive change in the energy sector, cogeneration is gaining increasing attention as a method to reduce emissions and improve resource efficiency. Many governments and organizations are recognizing the role of cogeneration in achieving energy transition targets and are providing financial incentives, grants, and tax breaks to encourage its adoption. Policies promoting energy efficiency and emissions reductions, such as carbon pricing, renewable energy mandates, and energy performance standards, are making cogeneration a more attractive option for industries looking to reduce their carbon footprint and energy costs.
Cogeneration’s potential for integrating with renewable energy sources is particularly significant in the context of the global push for decarbonization. As renewable energy technologies, such as solar, wind, and geothermal, become more widespread, cogeneration systems can be adapted to take advantage of these intermittent and decentralized energy sources. For example, in a hybrid cogeneration system, solar thermal collectors could provide the heat needed for a steam turbine while the turbine simultaneously generates electricity. This integration allows for the reliable supply of both power and thermal energy, reducing reliance on fossil fuels and enhancing the sustainability of industrial processes.
Furthermore, the ability of cogeneration systems to capture and utilize waste heat opens up opportunities for industries to reduce their environmental impact and improve the circularity of their operations. For example, in a food processing plant, the heat generated by a cogeneration system could be used for drying, pasteurization, or other thermal processes, further reducing the plant’s need for external energy sources. Similarly, in manufacturing industries, waste heat could be harnessed for preheating materials or driving mechanical processes, improving overall process efficiency and minimizing waste. This approach aligns with the growing trend of circular economy practices, where waste is minimized and resources are continuously reused.
As the technology behind cogeneration continues to advance, there is also the potential for more widespread adoption in smaller-scale applications, such as residential buildings, commercial complexes, and district heating networks. In these settings, smaller cogeneration units can be used to generate electricity and heat on-site, reducing energy costs for consumers and improving energy independence. These systems could also be paired with renewable energy sources, such as rooftop solar panels or small-scale wind turbines, to create highly efficient, decentralized energy networks that reduce reliance on traditional power grids and provide local energy resilience.
In the context of global energy transition efforts, cogeneration is expected to play an increasingly important role in industries such as heavy manufacturing, chemicals, cement, and metals, where process heat is a significant part of the energy demand. By improving energy efficiency and reducing fuel consumption, cogeneration can help these industries transition toward low-carbon energy systems, lowering both operational costs and emissions. The ability to integrate cogeneration with carbon capture and storage (CCS) technologies further enhances its potential for decarbonization, as it allows industries to capture and store CO2 emissions from the cogeneration process, further contributing to the reduction of their carbon footprint.
In conclusion, cogeneration represents a key strategy for improving energy efficiency, reducing emissions, and meeting the growing demand for both electricity and heat in a sustainable way. Its ability to integrate with renewable energy sources, adapt to changing energy demands, and contribute to circular economy principles positions it as an essential technology for the future of energy systems. As innovations in digital technologies, advanced materials, and hybrid systems continue to develop, cogeneration will become an increasingly integral part of the global energy landscape, helping to drive a more sustainable, resilient, and efficient energy future.
The expansion of cogeneration systems into new markets and applications is also a crucial development in the global energy landscape. As industries and municipalities look to become more energy efficient, there is a growing interest in deploying cogeneration for district heating and cooling systems. In urban areas, particularly those with dense populations or cold climates, cogeneration offers a way to meet the heating demands of multiple buildings while simultaneously generating electricity. By capturing waste heat from the cogeneration process, these systems can significantly reduce the need for separate heating plants, leading to energy savings and reduced emissions. District heating systems, often coupled with cogeneration plants, provide a reliable and efficient way to deliver thermal energy to residential, commercial, and industrial users, reducing reliance on fossil fuels and promoting the use of renewable energy sources.
The increasing focus on energy efficiency and sustainability also means that cogeneration is becoming more attractive in the context of global efforts to improve the environmental performance of cities and industries. In regions where energy security is a significant concern, particularly in developing countries, cogeneration can be used to provide reliable and cost-effective energy solutions. By decentralizing energy production and utilizing local resources, cogeneration reduces the dependence on centralized power grids, which may be vulnerable to disruptions caused by natural disasters or other crises. This decentralized energy model enhances resilience and can also lead to lower transmission and distribution losses, making the system more efficient overall.
In the industrial sector, where high-temperature processes are common, the role of cogeneration systems is becoming more prominent. Many heavy industries, such as petrochemicals, refineries, and cement manufacturing, require large amounts of both electricity and process heat to sustain their operations. Cogeneration offers these industries a way to simultaneously meet both of these needs in a highly efficient manner. The extracted steam from the cogeneration process can be used to drive industrial processes like drying, distillation, or chemical reactions, while the electricity generated can power the machinery or be fed into the grid. This dual energy provision not only reduces operational costs but also makes industries more competitive in an increasingly energy-conscious world.
In terms of future innovation, there is growing potential for cogeneration systems to integrate with carbon capture, utilization, and storage (CCUS) technologies. These technologies can capture CO2 emissions from the combustion of fossil fuels in cogeneration plants and either store the carbon underground or repurpose it for use in other applications, such as enhanced oil recovery or the production of chemicals and fuels. Integrating cogeneration with CCUS can significantly reduce the carbon footprint of industries that are traditionally difficult to decarbonize, such as cement, steel, and chemicals, making these sectors more sustainable in the long run. The combination of cogeneration and CCUS could become a key enabler of net-zero emissions targets, particularly in industries where eliminating CO2 emissions entirely through renewable energy is challenging.
Moreover, the development of new fuels and alternative energy sources provides even more opportunities for cogeneration. Biomass and biogas, derived from organic waste materials, are renewable fuels that can be used in cogeneration systems to produce both heat and power. Biomass cogeneration plants are particularly beneficial in regions with abundant agricultural or forestry waste, as they allow for the conversion of these materials into energy while reducing landfill waste and mitigating methane emissions. Similarly, biogas produced from organic waste materials such as food scraps, sewage, or agricultural byproducts can be used in cogeneration systems, providing a sustainable and environmentally friendly alternative to natural gas.
The rise of green hydrogen as a viable energy source is also poised to impact cogeneration systems. Hydrogen, when produced through electrolysis powered by renewable electricity, can serve as a clean fuel in cogeneration systems, emitting only water vapor when burned. As the cost of renewable hydrogen production continues to fall, hydrogen-based cogeneration systems could become a game-changer for industries looking to decarbonize their operations. For example, steel manufacturers could use hydrogen as a fuel to drive their cogeneration plants, helping them transition away from coal and reducing their overall carbon emissions.
The energy transition also brings an increased focus on the resilience of energy systems, particularly in the face of climate change and growing weather-related disruptions. As the frequency of extreme weather events rises, energy systems that rely on centralized, fossil-fuel-based power plants are becoming more vulnerable to outages and disruptions. Cogeneration systems, particularly those integrated with decentralized energy grids or microgrids, provide a more resilient solution, ensuring that communities and industries have access to reliable energy even during natural disasters or grid failures. By distributing energy generation and utilizing locally sourced fuels, cogeneration plants offer greater flexibility and reduce the impact of grid failures.
Furthermore, the ongoing digital transformation of energy systems will continue to enhance the performance and capabilities of cogeneration plants. The use of advanced digital technologies, such as sensors, data analytics, and AI, enables real-time monitoring and optimization of energy production. These tools can help operators track performance metrics, identify inefficiencies, and predict when maintenance or repairs are needed. By proactively addressing potential issues before they cause downtime, digital tools can extend the life of cogeneration systems and improve their overall efficiency.
As energy markets become increasingly complex, cogeneration systems are also being adapted to work in tandem with other distributed energy resources (DERs), such as rooftop solar, wind turbines, and energy storage systems. By integrating cogeneration with DERs, energy users can maximize their self-sufficiency, reduce reliance on the grid, and create more sustainable and resilient energy systems. For example, during periods of high renewable energy generation, a cogeneration system could be used to store excess energy in thermal storage or batteries for later use, ensuring that energy demand is met even when renewable energy sources are not producing at their peak.
In conclusion, the role of cogeneration in the future of energy systems is expected to grow significantly. With its ability to improve energy efficiency, reduce emissions, and integrate with renewable energy sources and advanced technologies, cogeneration offers a flexible and sustainable solution for meeting both electricity and thermal energy demands. The continued development of new fuels, digital technologies, and decentralized energy systems will further enhance the potential of cogeneration to contribute to a more sustainable, resilient, and efficient energy future. As industries, municipalities, and governments pursue their energy transition goals, cogeneration will remain a vital tool for achieving greater energy efficiency and environmental sustainability.
As the world continues to prioritize energy efficiency and sustainability, the role of cogeneration in both industrial and residential applications is becoming increasingly important. One of the major drivers of this trend is the growing need to reduce energy costs while simultaneously lowering carbon emissions. Cogeneration allows industries and facilities to optimize their energy consumption by producing both electricity and useful heat simultaneously, which reduces waste and increases the overall efficiency of energy production. This efficiency can be especially beneficial in energy-intensive industries such as chemicals, pulp and paper, cement, and metals, where both electricity and steam are vital for the production process.
One area where cogeneration is particularly effective is in the use of waste heat recovery. Many industries produce significant amounts of excess heat as a byproduct of their operations, which often goes unutilized or is vented into the environment. By capturing and repurposing this waste heat through a cogeneration system, industries can convert this otherwise lost energy into valuable electricity or process heat. This not only reduces their dependency on external energy sources but also helps in lowering their overall fuel consumption. For example, in a cement plant, waste heat from the kiln can be recovered and used to generate steam, which in turn drives a turbine to produce electricity. This energy recovery system significantly improves the plant’s energy efficiency, lowers operational costs, and reduces its environmental impact.
Cogeneration systems are also gaining attention in the context of sustainable building design. As urbanization increases and the demand for energy-efficient buildings rises, the integration of cogeneration into commercial and residential developments is becoming more common. In these settings, cogeneration plants can provide heating, cooling, and electricity, reducing a building’s reliance on the grid and promoting energy independence. For instance, in a large mixed-use development, a cogeneration unit can produce both hot water and electricity for the building’s residents while simultaneously reducing energy costs. Similarly, cogeneration is being used in district energy systems, where multiple buildings are connected to a central cogeneration plant that supplies both heat and electricity. This approach is particularly beneficial in urban areas, where space constraints and high energy demands make it difficult to rely solely on traditional energy sources.
One of the most significant advantages of cogeneration in urban areas is its potential to reduce grid congestion and improve energy resilience. By decentralizing energy production, cogeneration plants can help to alleviate stress on centralized power grids, particularly during periods of peak demand. In the event of grid failures or natural disasters, cogeneration plants can continue to operate independently or in conjunction with microgrids, providing a reliable source of electricity and heating. This capability is particularly valuable in regions that experience frequent power outages or where the energy infrastructure is vulnerable to disruptions. For example, during a power outage caused by a storm, a cogeneration plant serving a hospital or critical infrastructure facility can continue to supply electricity and heating, ensuring that vital operations remain unaffected.
As the renewable energy sector continues to grow, cogeneration is increasingly being integrated with renewable energy sources to create hybrid systems that maximize energy efficiency and minimize emissions. One example of this integration is the combination of cogeneration with solar thermal systems. In such a hybrid system, solar collectors capture solar energy and convert it into heat, which is then used in the cogeneration process to generate electricity. This combination reduces reliance on fossil fuels and helps to decarbonize the energy supply. Additionally, cogeneration systems can be paired with energy storage technologies, such as thermal storage or batteries, to store excess energy generated during periods of low demand and release it when needed. This ensures that the system can operate efficiently even when renewable energy sources, like solar or wind, are not producing electricity.
Furthermore, the rise of green hydrogen as a clean fuel source is opening up new possibilities for cogeneration systems. Green hydrogen, produced through electrolysis powered by renewable energy, can be used as a fuel in cogeneration plants to generate electricity and heat without emitting carbon dioxide. Hydrogen has the potential to replace natural gas in cogeneration systems, offering a low-carbon alternative to traditional fossil fuels. In regions with abundant renewable energy resources, such as wind and solar, hydrogen can be produced in large quantities and used to power cogeneration systems, further reducing the carbon footprint of industrial and residential energy consumption. The use of hydrogen in cogeneration systems is still in the early stages of development, but as the cost of hydrogen production continues to fall and infrastructure for its use improves, hydrogen-powered cogeneration systems are expected to play an increasingly important role in decarbonizing the energy sector.
In addition to energy efficiency and sustainability, the economic benefits of cogeneration are driving its adoption across a wide range of industries. Cogeneration systems often provide significant cost savings by reducing the amount of purchased energy needed to meet both electricity and thermal energy demands. This is particularly valuable for energy-intensive industries that rely heavily on both forms of energy for their operations. For example, in the chemical industry, where high-temperature steam is required for various processes, cogeneration can provide the necessary heat while simultaneously generating electricity for other parts of the plant. By using a single energy source to meet multiple needs, companies can significantly reduce their fuel consumption and operating costs. Furthermore, cogeneration plants can often be financed through performance-based contracts or power purchase agreements, which help to reduce the upfront capital investment required.
In some cases, cogeneration can even generate additional revenue for businesses by allowing them to sell excess electricity back to the grid. In regions with favorable feed-in tariffs or renewable energy incentives, businesses with cogeneration systems may be able to sell surplus energy to utilities, offsetting their initial investment and improving the financial viability of the system. The ability to generate both heat and electricity simultaneously makes cogeneration particularly attractive for businesses that have a constant need for both forms of energy. Whether it is a manufacturing facility, a large commercial complex, or a district heating network, cogeneration provides a flexible, cost-effective solution that maximizes energy efficiency and reduces overall operational expenses.
Looking ahead, the development of new technologies and innovations will continue to enhance the performance and capabilities of cogeneration systems. Advances in materials science, for example, may lead to more efficient turbines and heat exchangers, reducing energy losses and improving overall system efficiency. Additionally, the growing use of digital tools, such as predictive maintenance, remote monitoring, and optimization software, will allow operators to monitor and adjust cogeneration systems in real time, further improving their performance and extending their lifespan.
The future of cogeneration will also be shaped by the increasing demand for clean energy solutions. As global governments set ambitious carbon reduction targets and companies strive to meet sustainability goals, the adoption of cogeneration is expected to grow. The technology’s ability to reduce energy consumption, lower emissions, and provide a reliable energy supply will make it an essential component of the future energy mix, particularly in industries and regions where both electricity and heat are needed. By continuing to innovate and adapt to changing energy needs, cogeneration systems will remain a cornerstone of efficient and sustainable energy production in the years to come.
Adaptability of Double Extraction Steam Turbines
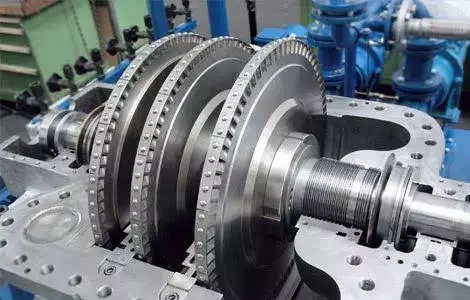
Double extraction steam turbines are known for their remarkable adaptability in various industrial and energy production settings. These turbines are designed to efficiently extract steam at two separate stages, which allows them to be tailored to meet a range of operational needs. The adaptability of double extraction steam turbines lies not only in their ability to operate across diverse industries but also in their flexibility to respond to changing demands for both electricity and thermal energy.
One of the key features that make double extraction steam turbines so adaptable is their ability to operate in systems where both electricity and heat are required. In many industrial applications, especially those in the chemical, petrochemical, and paper industries, there is a significant need for process heat alongside electricity generation. The double extraction steam turbine can extract steam at two points, allowing it to provide heat at different temperatures depending on the needs of the process. This dual-purpose capability makes it highly versatile and ideal for combined heat and power (CHP) plants. The first steam extraction typically occurs at a higher pressure, providing heat for processes that require high-temperature steam, while the second extraction, at a lower pressure, can supply heat to processes that require lower temperature steam or even feed into district heating networks. This flexibility allows the turbine to adapt to varying industrial energy requirements, making it a valuable asset for plants that must efficiently balance electricity production with thermal energy needs.
In addition to meeting the varying needs for steam at different pressures, the adaptability of double extraction turbines extends to their capacity to respond to fluctuating energy demands. In industries where both electricity and heat are essential, there can be times when one energy source is needed more than the other. For example, during periods of high electrical demand, the turbine can operate to generate more power, with the extractions adjusted accordingly. Conversely, when the thermal load increases due to heating requirements, the turbine can adapt to extract more steam for heating while reducing its power output. This dynamic adaptability is particularly important in industries with variable energy needs, as it allows for continuous, efficient operation without unnecessary energy waste. Additionally, double extraction steam turbines can be integrated with energy storage systems, such as thermal energy storage, allowing for even greater flexibility in meeting energy demands. In this case, excess heat or power can be stored and used when demand increases, enhancing the overall system’s efficiency and reducing operational costs.
The turbine’s adaptability is also evident in its ability to operate in a variety of operational configurations. Whether it’s part of a stand-alone CHP system or integrated into a larger combined-cycle or multi-stage turbine setup, double extraction steam turbines can be optimized for specific applications. For instance, in a combined-cycle plant, a gas turbine may generate electricity while the waste heat is used to produce steam for the double extraction turbine, enhancing overall plant efficiency. This type of integration is increasingly common in modern power generation facilities, where maximizing efficiency and minimizing environmental impact are key priorities. The ability of the double extraction turbine to operate seamlessly within these complex configurations allows it to contribute effectively to both power generation and heat recovery, making it a versatile solution for a wide range of energy systems.
Moreover, double extraction steam turbines can be modified or upgraded over time to accommodate changes in operational conditions or energy demand. This is particularly beneficial in industries where energy needs evolve due to changes in production processes, fuel availability, or regulatory requirements. Turbines can be retrofitted with advanced control systems, new blade materials, or optimized steam path configurations to improve efficiency or expand their range of operation. This adaptability to technological advancements and changing operational parameters ensures that the double extraction steam turbine remains relevant and capable of meeting evolving energy requirements over its lifespan.
Another aspect of the adaptability of double extraction steam turbines is their ability to integrate with renewable energy sources. As the energy sector transitions towards decarbonization, the integration of renewable energy, such as biomass, solar, or wind, into traditional energy systems becomes more common. Double extraction steam turbines can complement these renewable sources by providing a reliable backup power supply or supporting hybrid energy systems. For example, in a biomass-to-power plant, the double extraction steam turbine can be used to efficiently convert biomass into both heat and electricity, providing an adaptable and sustainable solution for regions that rely on renewable fuels. In such systems, the turbine can adjust its operation to balance the intermittent nature of renewable energy generation, ensuring that the supply of electricity and thermal energy remains stable.
In industries that rely on highly variable energy demands, such as district heating, the ability of double extraction turbines to modulate their output to match the changing needs of consumers is an invaluable characteristic. District heating systems often require significant amounts of thermal energy during colder months, and the demand for both heat and electricity can fluctuate daily or seasonally. The double extraction steam turbine’s ability to adjust its output—either generating more power or providing additional steam for heating—ensures that the district heating system can meet consumer demand while maintaining operational efficiency.
The environmental adaptability of double extraction steam turbines also contributes to their appeal in the transition to more sustainable energy systems. These turbines are designed to operate with a variety of fuels, including both traditional fossil fuels and renewable sources such as biomass or biogas. In the context of a transition to lower-carbon energy systems, the turbine’s ability to accommodate different fuels makes it a flexible solution for industries seeking to reduce their carbon footprint while still maintaining reliable energy production. Furthermore, the high efficiency of double extraction turbines, especially when integrated into CHP plants, minimizes fuel consumption and emissions, which supports global efforts to reduce greenhouse gas emissions.
Lastly, the adaptability of double extraction steam turbines is reflected in their widespread use across different geographical regions and industrial sectors. From large-scale power plants to smaller, decentralized cogeneration systems, these turbines can be scaled up or down to meet local energy needs. In remote or off-grid areas, where access to reliable power may be limited, the double extraction turbine can provide a self-sustaining energy solution that produces both electricity and heat for local communities or industrial operations. Similarly, in urban areas with dense populations and complex energy networks, the turbine’s ability to work within district energy systems or large-scale industrial processes makes it a valuable component of modern energy infrastructure.
In conclusion, the adaptability of double extraction steam turbines lies in their ability to operate in a variety of energy production contexts, meet fluctuating demand for electricity and heat, integrate with renewable energy sources, and evolve with technological advancements. Their versatility in both industrial and power generation settings, coupled with their ability to optimize efficiency and reduce environmental impact, makes them a critical component of modern energy systems. As industries continue to embrace energy efficiency, sustainability, and flexibility, the double extraction steam turbine will remain a reliable and adaptable solution for meeting the complex and evolving demands of energy production.
The adaptability of double extraction steam turbines extends beyond the industrial and power generation sectors and into applications where highly dynamic and tailored energy outputs are essential. In such diverse environments, these turbines help to address specific operational requirements while maintaining efficiency and minimizing waste. Their ability to respond flexibly to varying heat and power demands has led to their widespread application in areas such as district heating, commercial cogeneration, and integrated industrial systems.
In district heating systems, for instance, the adaptability of double extraction turbines plays a pivotal role in ensuring that communities and industrial zones receive a steady supply of both electricity and thermal energy. These systems often experience fluctuations in heating demand, driven by seasonal changes or daily shifts in weather patterns. The ability of double extraction turbines to adjust their steam extraction pressures allows operators to efficiently manage this variability. During colder months or periods of peak heating demand, the turbine can increase steam extraction to provide additional heat, while scaling back its power generation. Similarly, during milder conditions, the turbine can focus more on power generation, optimizing the balance between electricity supply and thermal demand.
The integration of double extraction turbines into commercial cogeneration systems further highlights their adaptability. In commercial applications such as large hotels, hospitals, and manufacturing plants, the simultaneous need for electricity and heat can vary dramatically depending on usage patterns, weather conditions, or production schedules. A double extraction steam turbine can modulate its output to meet the energy needs of the building or facility in real time. This is especially important in industries that require consistent thermal energy for heating, drying, or chemical processes, while also needing electricity for lighting, cooling, or machinery. The turbine’s ability to provide a stable, on-demand supply of both types of energy allows businesses to lower energy costs, reduce reliance on external grid power, and achieve more efficient and sustainable operations.
Moreover, as industries move towards more decentralized energy models, the adaptability of double extraction steam turbines is even more critical. In a decentralized energy system, such as a microgrid or localized energy network, the turbine can operate in tandem with other distributed energy resources, such as renewable power sources, batteries, or fuel cells, to ensure a consistent energy supply. These systems are particularly valuable in remote or off-grid areas, where centralized power grids may not be available or reliable. By extracting steam at two stages, the double extraction turbine can optimize its output based on the energy demands of the microgrid, ensuring that both electricity and heat are available when needed, and reducing the need for backup power generation.
The flexibility of double extraction turbines also allows for their use in systems that involve combined heat and power (CHP) generation using alternative fuels. As the energy industry shifts toward more sustainable practices, the integration of renewable fuels, such as biomass, biogas, or hydrogen, is becoming increasingly important. Double extraction steam turbines can operate effectively with these renewable fuels, providing a more sustainable way of generating both power and heat. For example, in a biomass plant, the turbine can extract steam from the combustion of organic materials at different pressures, supplying both the heat needed for industrial processes and the electricity required by the grid or local users. The ability to switch between fuels or integrate multiple fuel types without sacrificing performance makes double extraction turbines well-suited for adapting to changing energy sources and regulatory requirements.
In addition to fuel flexibility, double extraction turbines can be designed to operate across a wide range of load conditions. This feature is especially useful in plants or facilities that experience fluctuating or unpredictable demand. For example, in an industrial process that requires steam for heating but can tolerate fluctuations in electricity production, the turbine can adjust its performance to prioritize steam extraction, reducing electricity output during low-demand periods. Conversely, when electricity demand rises, the turbine can shift focus to generate more power, ensuring that both electricity and heat are efficiently distributed as needed. This on-demand flexibility provides operators with the ability to fine-tune the turbine’s operation to optimize energy usage while reducing waste.
The turbine’s ability to adapt to these various scenarios is made possible by advancements in turbine design and digital control systems. Modern double extraction turbines can be equipped with advanced monitoring systems that use real-time data to optimize performance. Sensors can track steam flow, pressure, temperature, and efficiency, enabling operators to adjust the turbine’s operation on the fly to respond to changes in load or fuel quality. These data-driven insights allow for predictive maintenance, minimizing downtime and ensuring that the turbine operates at peak efficiency throughout its lifecycle. Additionally, the integration of automation and AI into turbine control systems enables operators to respond to fluctuations in demand more quickly and with greater precision, further enhancing the turbine’s adaptability.
One of the emerging areas where double extraction turbines are proving their adaptability is in the integration of carbon capture, utilization, and storage (CCUS) technologies. As industries aim to reduce their carbon emissions, integrating CCUS with cogeneration systems powered by double extraction turbines presents a potential solution for mitigating the environmental impact of industrial operations. The turbine can be used to generate power and heat, while carbon capture systems can capture CO2 emissions before they are released into the atmosphere. The captured CO2 can then be utilized in other processes, such as enhanced oil recovery, or stored underground to reduce the plant’s overall carbon footprint. This combination of energy generation and emissions reduction makes double extraction turbines a valuable component of low-carbon energy systems and helps industries transition toward more sustainable practices.
In addition to their role in carbon management, double extraction turbines can also contribute to the overall resilience of the energy system. Their adaptability allows them to function effectively in a wide range of environmental and operational conditions. For instance, in regions prone to extreme weather events or natural disasters, the ability to quickly adjust the output of double extraction turbines ensures that critical infrastructure, such as hospitals, data centers, and emergency response centers, can maintain a steady supply of electricity and heat. This resiliency is especially important as climate change leads to more frequent and intense weather events that threaten the reliability of traditional power systems.
Finally, the long-term economic adaptability of double extraction steam turbines should not be overlooked. The ability of these turbines to operate efficiently with both conventional and renewable fuels, their flexibility in adjusting steam extraction pressures, and their integration with advanced control systems allow them to remain valuable investments over time. As energy prices fluctuate or regulatory requirements change, the turbine’s ability to adapt to these external factors ensures that it continues to deliver reliable and cost-effective energy solutions. This long-term adaptability, coupled with lower operational costs, can provide industries with significant financial savings and reduce their overall exposure to energy market volatility.
In conclusion, the adaptability of double extraction steam turbines is a key factor in their widespread adoption across various sectors. Their ability to provide both electricity and heat, adjust to fluctuating demand, integrate with renewable energy sources, and operate with multiple fuels makes them a highly versatile solution for modern energy systems. Whether used in large-scale industrial applications, decentralized energy networks, or renewable-based cogeneration systems, the adaptability of double extraction turbines helps industries optimize energy efficiency, reduce environmental impact, and improve system resilience. As the global energy landscape continues to evolve, the adaptability of double extraction steam turbines will remain a vital asset in achieving a sustainable, flexible, and efficient energy future.
The adaptability of double extraction steam turbines is not just limited to operational flexibility, but also extends to their role in enhancing the overall efficiency and sustainability of energy systems. This flexibility allows these turbines to play a key role in the shift towards more decentralized and integrated energy networks, helping to address both environmental concerns and the growing demand for reliable and affordable energy across the globe. As industries and power plants work toward increasing their energy efficiency and minimizing carbon emissions, double extraction steam turbines provide a versatile and dynamic solution.
In addition to traditional industrial applications, the integration of double extraction steam turbines into hybrid systems—where they work alongside various energy technologies—further illustrates their adaptability. For example, in an industrial facility, a double extraction turbine might be combined with a waste heat recovery system, which can capture excess heat from a production process that would otherwise be wasted. This recovered heat can be fed directly into the turbine to increase steam production, thereby improving energy efficiency without additional fuel consumption. Similarly, double extraction turbines can be integrated with renewable technologies like solar thermal, geothermal, or even wind power, to create a more resilient and sustainable hybrid energy system. By utilizing various energy sources in tandem, these systems ensure that energy production remains reliable, even when renewable energy output fluctuates due to weather conditions or seasonal changes.
One specific example of this hybrid adaptability is in combined-cycle power plants, where double extraction steam turbines are paired with gas turbines. In such a configuration, the gas turbine generates electricity using natural gas, and the waste heat from the gas turbine is used to generate steam, which then drives the double extraction turbine. This arrangement allows for highly efficient energy production, with the double extraction turbine generating both power and useful heat for industrial processes or district heating. By combining the electricity generation from the gas turbine and the thermal energy recovery through the steam turbine, the combined-cycle system maximizes the energy extracted from the same fuel, leading to improved fuel efficiency and reduced emissions.
Double extraction steam turbines can also contribute to the decarbonization of the energy sector by enabling the integration of low-carbon fuels such as hydrogen, biogas, or synthetic fuels into existing infrastructure. For example, in a hydrogen-fueled system, hydrogen can be used as a clean alternative to natural gas in the combustion process, reducing carbon emissions and providing a sustainable solution for industrial energy needs. The double extraction turbine can operate with these alternative fuels, delivering both power and thermal energy in a manner that complements the broader goal of reducing greenhouse gas emissions. The ability to shift between various fuel types while maintaining consistent performance adds to the turbine’s adaptability in responding to changing energy demands and fuel availability.
The design of double extraction steam turbines also allows for scalability, which is particularly useful in large, diverse energy networks or in areas where energy demands are expected to grow over time. These turbines can be implemented in modular configurations, allowing for easy expansion or modification as demand increases. For example, in a large industrial facility, multiple double extraction turbines can be installed to meet the growing electricity and heat demands as the plant scales. Furthermore, new technologies in turbine materials, such as advanced alloys and coatings, are helping to improve the durability and efficiency of these turbines, allowing them to operate for longer periods while withstanding higher temperatures and pressures. This makes them more suitable for a wide range of environments and conditions, enhancing their overall adaptability.
Another aspect of their adaptability is the potential for integration with digital technologies to further optimize turbine operation. The rise of the Industrial Internet of Things (IIoT) and smart grids has enabled greater connectivity and automation in energy systems, allowing turbines to be integrated into real-time monitoring and control networks. Through advanced sensors and data analytics, operators can gain insights into the turbine’s performance and make adjustments as needed to optimize efficiency and reduce energy consumption. Predictive maintenance algorithms can also be implemented to detect potential issues before they become serious problems, minimizing downtime and reducing operational costs. The ability to constantly monitor and optimize turbine performance through these digital tools adds another layer of adaptability, ensuring that the turbine operates at peak efficiency throughout its operational life.
In terms of environmental impact, double extraction steam turbines can be pivotal in supporting industries and power plants in their efforts to meet sustainability goals. With increasing regulations aimed at reducing carbon emissions and improving energy efficiency, the adoption of efficient technologies such as double extraction steam turbines is becoming a strategic priority. These turbines can help organizations reduce their dependence on fossil fuels, improve fuel efficiency, and lower emissions by optimizing the use of energy resources. Furthermore, their ability to efficiently manage both power and thermal energy simultaneously allows for a reduction in overall energy consumption and waste, contributing to more sustainable production practices.
The versatility and efficiency of double extraction steam turbines make them ideal candidates for industries that need to balance multiple energy demands while maintaining environmental responsibility. In the manufacturing sector, for instance, a double extraction turbine can provide a reliable source of electricity for machinery and equipment, while also generating steam for heating, drying, or processing operations. This ability to deliver two forms of energy from a single source streamlines operations, reduces fuel consumption, and minimizes emissions by ensuring that waste heat is not released into the environment without being repurposed.
As the global energy transition continues, double extraction steam turbines are positioned to play an essential role in shaping the future of energy production. Their ability to adapt to different fuels, configurations, and operational conditions enables them to meet the ever-evolving energy demands of industries, commercial buildings, and communities. By offering an efficient, sustainable solution that addresses both power and heat requirements, these turbines will continue to contribute to the decarbonization of energy systems, the reduction of energy costs, and the improvement of overall energy efficiency. Whether in the context of large-scale power generation, industrial cogeneration, or integrated renewable energy systems, the adaptability of double extraction steam turbines ensures that they will remain a key component of the energy landscape for years to come.
Operational Flexibility of Double Extraction Steam Turbines
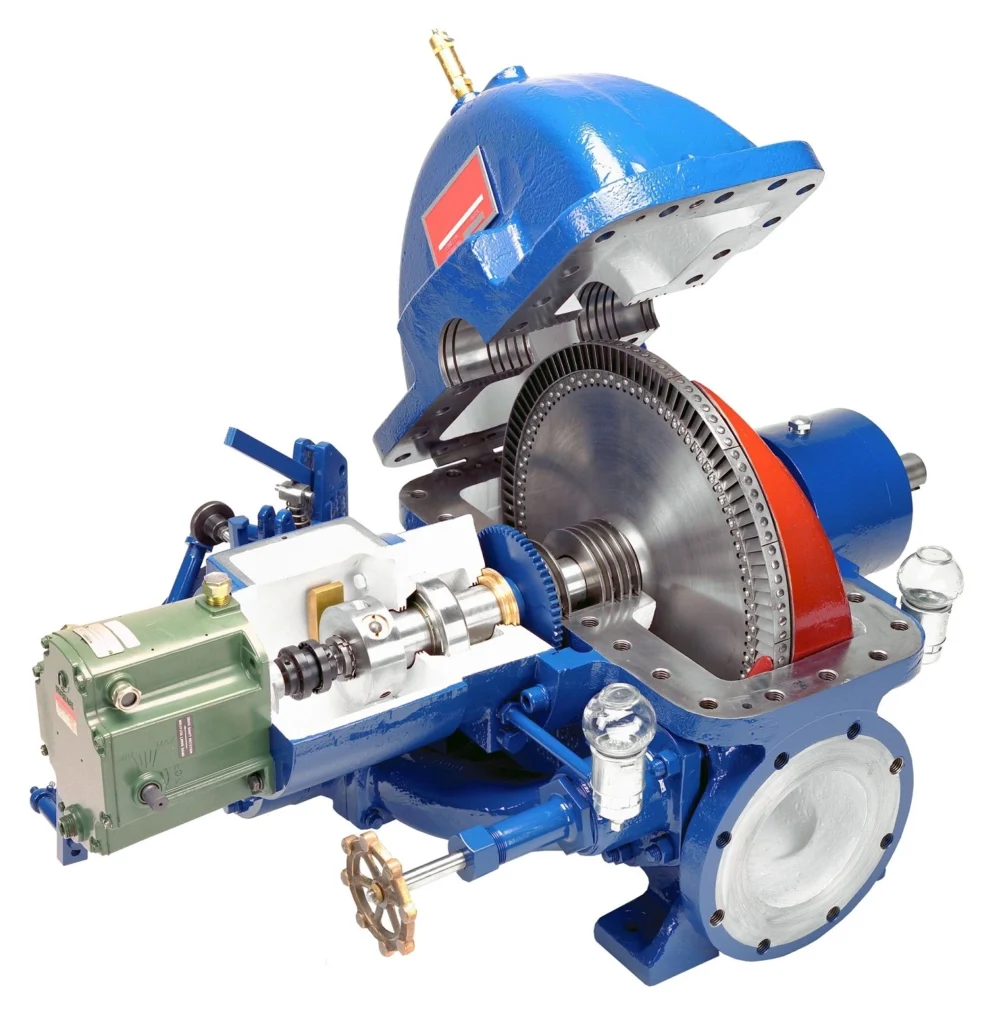
Double extraction steam turbines offer significant operational flexibility, making them highly adaptable to a wide range of energy generation systems. Their ability to produce both electricity and useful thermal energy simultaneously, by extracting steam at two different pressures, provides a level of versatility that is crucial for optimizing energy use across various applications. This operational flexibility allows these turbines to respond dynamically to the changing energy needs of industrial facilities, power plants, and combined heat and power (CHP) systems, where both electrical power and thermal energy are essential.
One of the key aspects of operational flexibility in double extraction steam turbines is their ability to adjust to varying demand for both heat and power. In industrial settings, where energy requirements fluctuate based on factors such as production schedules, seasonal changes, and weather conditions, the turbine can quickly adapt its output. For instance, in a facility that uses both heat and electricity for different processes, the turbine can modulate its steam extraction levels to prioritize one form of energy over the other. During periods of high thermal demand, such as in colder months or during intensive production processes, the turbine can increase the amount of steam extracted for heating purposes, while reducing power generation. Conversely, during times of increased electricity demand or lower heating requirements, the turbine can shift to producing more electricity, ensuring that both energy forms are available as needed without wasting resources.
This flexibility extends to the ability to operate at varying loads and adjust steam extraction pressures based on the specific needs of the system. Double extraction turbines are often used in systems where there are multiple levels of heat requirement, and their capacity to extract steam at two different pressures allows them to meet these needs efficiently. For example, the turbine can provide high-pressure steam for processes that require elevated temperatures, such as chemical or food processing industries, while also supplying lower-pressure steam for district heating or other applications that require moderate temperatures. This fine-tuned control over steam extraction not only improves overall system efficiency but also ensures that the energy produced is closely aligned with the operational needs of the plant or facility.
Furthermore, double extraction steam turbines are often part of larger, integrated energy systems, where they can be combined with other technologies to optimize overall performance. In a combined-cycle power plant, for example, the turbine can work in conjunction with a gas turbine. The gas turbine produces electricity by burning natural gas, and the waste heat from this process is used to generate steam for the double extraction turbine. This process allows for the recovery and utilization of waste heat, which would otherwise be lost, making the entire system more efficient. The flexibility of double extraction steam turbines to operate seamlessly in such integrated configurations makes them ideal for maximizing energy recovery and minimizing fuel consumption.
In combined heat and power (CHP) plants, where both electricity and thermal energy are generated for industrial processes or district heating, double extraction turbines excel due to their ability to switch between different operational modes depending on the demand. When the thermal load increases, for instance, the turbine can increase its steam extraction to provide additional heat for industrial heating processes or for supplying district heating networks. During off-peak times when heating demand is lower, the turbine can scale back its thermal energy output and focus more on electricity production. This capability enables the CHP system to operate at optimal efficiency, ensuring that energy is used as effectively as possible without generating excess waste heat or unused electricity.
Additionally, the operational flexibility of double extraction turbines allows them to adapt to changes in fuel sources or fuel mixtures. As the global energy landscape shifts towards lower-carbon and renewable energy sources, the ability of these turbines to operate with various fuels—such as biomass, biogas, hydrogen, or synthetic fuels—adds another layer of flexibility. These turbines can be retrofitted or designed to handle different types of fuels, helping industries transition to cleaner energy options without needing to overhaul the entire system. For example, a double extraction turbine that has been operating on natural gas can be adjusted to work with a mix of renewable biomass or hydrogen, making it more adaptable to changing fuel availability or environmental regulations.
Moreover, the operational flexibility of double extraction steam turbines is enhanced by modern digital technologies and control systems. With advancements in automation and real-time monitoring, operators can continuously adjust turbine settings to meet fluctuating energy demands or changes in operational conditions. Smart sensors and predictive analytics can optimize steam extraction pressures, track performance, and identify inefficiencies or potential issues before they cause significant problems. These control systems allow for more precise management of the turbine’s operation, ensuring that it runs efficiently even under variable conditions, such as changes in fuel quality, pressure, or temperature.
This operational flexibility also translates into enhanced reliability and resilience for the overall energy system. In plants where energy demand is subject to rapid changes, such as in the case of unexpected fluctuations in production schedules or load shifts in district heating systems, the double extraction turbine can quickly respond to ensure a stable supply of energy. Its ability to operate across a range of output levels and adjust steam extraction pressures ensures that it can continue to function efficiently, even when external conditions are not constant. Additionally, the ability to scale up or down in response to load changes means that the turbine can maintain optimal performance over extended periods without the need for frequent maintenance or shutdowns.
Double extraction steam turbines also contribute to the operational flexibility of industrial processes by reducing the need for multiple standalone energy systems. In many industries, separate systems are required to provide electricity and heat, which can lead to inefficiencies, higher maintenance costs, and operational complexity. The dual-purpose capability of double extraction turbines, however, consolidates these functions into one integrated system, reducing the need for additional equipment and simplifying plant operations. This streamlining of energy generation helps companies cut costs, minimize downtime, and achieve more efficient overall energy use.
The operational flexibility of double extraction steam turbines also supports their role in supporting grid stability and balancing energy supply and demand. In areas where the power grid is subject to fluctuations, such as when integrating renewable energy sources like wind or solar power, double extraction turbines can help maintain grid stability by providing backup power. When the availability of renewable energy sources drops due to weather conditions or other factors, the turbine can ramp up electricity production, ensuring that demand is met without interruptions. Similarly, during periods of surplus renewable energy, the turbine can reduce its power output and focus on providing thermal energy, helping to balance the grid and prevent excess energy from being wasted.
Furthermore, the flexibility of double extraction steam turbines to respond to real-time operational conditions enhances their role in reducing operational costs. In many industrial applications, especially those that require both electricity and heat, fluctuating energy prices can have a significant impact on overall costs. By allowing operators to adjust energy output based on market prices, the turbine can help optimize energy use, reduce reliance on external power sources, and minimize energy costs during peak price periods. This ability to adapt to price signals from the energy market is especially important for industries that are energy-intensive and need to balance operational costs with energy efficiency.
In conclusion, the operational flexibility of double extraction steam turbines is a key factor in their widespread use across various industries. Their ability to produce both power and thermal energy, adjust to fluctuating energy demands, integrate with other energy technologies, and operate on a variety of fuels makes them a highly adaptable and efficient solution for modern energy systems. Whether in large-scale power generation, industrial cogeneration, or district heating systems, the turbine’s flexibility ensures that energy production remains reliable and efficient under a wide range of conditions, enhancing both economic and environmental performance. As energy demands continue to evolve, the operational flexibility of double extraction steam turbines will remain a critical asset in meeting the complex energy needs of the future.
The operational flexibility of double extraction steam turbines plays an important role not just in responding to immediate changes in energy demand, but also in adapting to long-term shifts in operational strategy and the energy landscape. In industries where energy consumption patterns evolve over time due to factors like expansion, technological advancements, or changes in regulatory frameworks, the ability of these turbines to adjust to new requirements is a crucial advantage.
One of the key features of this adaptability is the turbine’s capability to integrate seamlessly into systems that require a balance between multiple energy forms. As industries adopt new technologies or shift their focus toward greater sustainability, the operational flexibility of double extraction turbines ensures that they remain effective, even as the nature of energy production or consumption changes. For example, in a manufacturing plant that begins to adopt more energy-efficient equipment or processes, the overall energy demand might decrease, but there will still be a need for consistent heat and power. The double extraction turbine can easily scale back or adjust its output to match the new, lower demand without sacrificing performance. Conversely, if the facility later increases its energy consumption due to expanding operations, the turbine can adapt again, increasing its steam extraction for heating while ensuring a consistent supply of electricity.
Additionally, the integration of these turbines into more complex energy systems, such as microgrids or hybrid energy solutions, further enhances their operational flexibility. Microgrids, which are localized energy networks that can operate independently or in conjunction with the larger grid, are becoming increasingly important as a means of improving energy resilience and promoting sustainability. Double extraction turbines are ideally suited for such systems due to their dual-purpose nature, providing both heat and power in a way that supports grid independence while optimizing energy efficiency. In these scenarios, the turbine can adjust to changing input from renewable sources like wind or solar, which often have variable generation profiles. When renewable power is abundant, the turbine can reduce its power output and focus on thermal energy production, storing excess energy from the renewables for later use. During periods of low renewable generation, the turbine can ramp up its electricity generation to ensure a steady supply of power, effectively complementing the intermittent nature of renewable sources.
The increased integration of digital control systems also enhances the operational flexibility of double extraction steam turbines. With advancements in automation and real-time monitoring, these turbines can be equipped with sensors that track and respond to fluctuations in pressure, temperature, and steam flow. This enables more precise adjustments to steam extraction rates and turbine output, further improving the turbine’s ability to adapt to dynamic operational conditions. Operators can use sophisticated software tools to monitor the turbine’s performance remotely and make adjustments on the fly, ensuring that the turbine operates at optimal efficiency regardless of changing loads, fuel qualities, or energy prices.
Another factor that enhances the operational flexibility of double extraction turbines is their ability to integrate with energy storage systems. As energy storage technologies, such as batteries and thermal storage, continue to improve, they provide an opportunity for double extraction turbines to further optimize their performance. For instance, in a cogeneration system, the turbine can generate electricity when demand is high, while excess thermal energy can be stored for later use. When energy demand peaks or when renewable generation falls short, the stored thermal energy can be used to maintain a steady supply of heat without relying on additional fuel consumption. This ability to combine energy generation with storage makes the turbine even more adaptable, ensuring that the system can operate efficiently regardless of external factors.
Double extraction turbines also benefit from advances in turbine design and materials that further enhance their operational flexibility. Modern turbines are built with materials that allow them to withstand a wider range of temperatures and pressures, enabling them to operate more efficiently under a variety of conditions. This means that a turbine designed to handle high-pressure steam can also adjust to lower-pressure operation without significant losses in efficiency. The ability to operate across different pressure regimes without sacrificing performance makes the double extraction turbine an even more flexible solution for industries and power plants with complex or varying energy needs.
As energy efficiency becomes an increasingly important consideration for industries looking to reduce costs and improve sustainability, the operational flexibility of double extraction steam turbines allows them to play a key role in achieving these goals. By optimizing both power and heat generation, these turbines minimize fuel consumption and reduce emissions, contributing to cleaner, more sustainable energy systems. In addition, the ability to rapidly adjust to changing operational conditions, such as fluctuating demand or fuel availability, ensures that the turbine can continue to operate at peak efficiency throughout its lifecycle, helping to reduce overall operational costs and improve financial performance.
Moreover, the turbine’s flexibility extends to its capacity for integration with carbon capture technologies, further expanding its role in reducing environmental impact. In industries where carbon emissions are a concern, such as power generation and heavy industry, double extraction turbines can be used in conjunction with carbon capture, utilization, and storage (CCUS) technologies to mitigate CO2 emissions. By producing both power and heat with minimal waste, these turbines help reduce the overall carbon footprint of the system. The integration of CCUS with double extraction turbines allows for more efficient capture of CO2 emissions, ensuring that the turbine’s operational flexibility aligns with long-term sustainability goals.
In conclusion, the operational flexibility of double extraction steam turbines is one of their defining features, enabling them to adapt to a wide variety of applications and operational conditions. Their ability to simultaneously produce electricity and heat, adjust to fluctuating demands, integrate with renewable energy sources and storage systems, and operate on a range of fuels ensures that they remain a versatile solution for modern energy systems. Whether used in industrial cogeneration, district heating, combined-cycle plants, or renewable energy integration, the operational flexibility of double extraction turbines helps optimize energy production, reduce emissions, and improve overall system efficiency. As energy demands continue to evolve, the ability of these turbines to adapt to changing conditions will remain a critical asset in meeting both operational and environmental goals.
The operational flexibility of double extraction steam turbines is also critical in addressing the challenges posed by the global transition towards more decentralized and renewable-based energy systems. As more energy producers incorporate renewable sources like solar, wind, and hydroelectric power into their portfolios, the traditional central power plants that rely on constant, predictable energy sources face increasing difficulties in balancing supply and demand. The variability of renewable sources creates an added complexity, but double extraction steam turbines provide a versatile solution to stabilize energy systems.
One significant advantage is their ability to complement the intermittent nature of renewable energy production. Solar and wind power, for example, depend heavily on weather patterns and geographic conditions, often leading to periods of excess generation followed by lulls where power production falls short. In such cases, double extraction turbines can quickly adjust to these fluctuations in renewable output. During times of excess renewable energy, the turbine can shift its focus towards generating heat, utilizing the surplus energy to meet industrial heating needs or district heating requirements. On the other hand, when renewable generation drops, the turbine can increase its electricity generation, ensuring a continuous, stable power supply without requiring immediate external inputs.
In this context, double extraction turbines provide critical grid balancing capabilities. In decentralized energy networks or microgrids, where energy is generated locally but needs to be shared or transmitted across the network, the turbine can offer both generation and stabilization functions. By adjusting its output in response to real-time demand, the turbine helps to smooth out the fluctuations inherent in renewable energy sources, ensuring grid stability. This becomes especially relevant when integrating distributed generation sources, as double extraction turbines provide a dynamic solution that supports the grid’s overall flexibility.
Furthermore, the operational flexibility of double extraction turbines also enables them to meet different regulatory and market conditions. Energy markets are increasingly shifting towards systems that prioritize flexibility and demand response, with dynamic pricing schemes that incentivize producers to adjust their output based on real-time market conditions. Double extraction steam turbines, with their ability to modulate both heat and electricity production, are well-positioned to respond to these market signals. During peak demand periods, when energy prices are higher, the turbine can increase its power generation, taking advantage of the higher prices to maximize revenue. Conversely, during periods of low demand or when energy prices drop, the turbine can reduce its electricity output, focusing on thermal energy production or even shutting down temporarily to minimize operating costs. This level of responsiveness allows operators to optimize turbine performance and profitability, providing a competitive advantage in the increasingly price-sensitive energy markets.
Additionally, the ability of double extraction turbines to operate across a range of fuel types further enhances their operational flexibility. As the world moves towards decarbonization, industries and power plants must be able to switch between traditional fossil fuels and cleaner, renewable alternatives. Double extraction turbines can be adapted to run on a variety of fuels, including natural gas, biomass, biogas, hydrogen, and synthetic fuels, allowing them to maintain reliable operation even as fuel sources change. This adaptability ensures that operators can continue to utilize their existing infrastructure as they transition towards lower-carbon energy sources, reducing the need for costly replacements and upgrades. Furthermore, this fuel flexibility enables operators to take advantage of lower-cost fuels when they become available, optimizing fuel use and further improving efficiency.
The operational flexibility of double extraction turbines is also critical in emergency situations. In regions prone to natural disasters, such as hurricanes, earthquakes, or severe weather, the ability of a turbine to respond quickly to fluctuating energy needs is essential for maintaining grid stability and ensuring energy security. These turbines can be integrated with backup power systems, providing a reliable and rapid response when external power sources are disrupted. Whether it’s through increased power generation, adjusting thermal output for district heating, or integrating with energy storage systems to maintain consistent supply, double extraction steam turbines contribute to resilience in disaster recovery and emergency response scenarios.
Beyond large-scale power generation, the operational flexibility of double extraction steam turbines makes them highly effective in smaller, localized energy systems. In remote or off-grid locations, where energy demand can fluctuate unpredictably, the turbine can offer a cost-effective and efficient solution to meet both electricity and heating needs. These turbines can be integrated into local combined heat and power (CHP) plants, providing a steady energy supply while minimizing waste heat. In such installations, the turbine’s ability to adjust output based on local needs ensures that the community has a reliable source of energy, even when external supply lines are unreliable or unavailable.
In many cases, double extraction steam turbines are also used in industries where energy use is tied directly to production schedules, such as in the chemical, paper, or food processing sectors. In these industries, production cycles can be highly variable, with energy demand fluctuating depending on factors like production volume or specific process requirements. The turbine’s ability to modulate its output in real-time allows it to meet these varying energy demands without creating excess waste or incurring unnecessary costs. Additionally, as industries become more automated and data-driven, the integration of advanced monitoring and control systems further enhances the turbine’s operational flexibility. By utilizing data from sensors and predictive analytics, operators can optimize turbine performance, improving both efficiency and reliability while reducing the potential for downtime.
The future of energy systems is increasingly characterized by the need for greater flexibility and resilience, and the operational flexibility of double extraction steam turbines positions them as key assets in this transition. As the energy sector moves towards more distributed, low-carbon, and renewable-based systems, the ability of these turbines to adapt to changing conditions—whether in response to market demands, fuel availability, or renewable energy generation—ensures that they will continue to play an essential role. Their ability to provide both power and heat, combined with the ability to respond dynamically to changing energy needs, makes them a versatile and reliable technology for the evolving energy landscape. In this way, double extraction steam turbines will remain integral to ensuring energy systems are both efficient and resilient, supporting both industrial and grid-level operations for years to come.
As the demand for sustainable energy solutions continues to rise, the operational flexibility of double extraction steam turbines is increasingly seen as a strategic asset in achieving both economic and environmental goals. Their ability to adapt to evolving energy systems, fluctuations in energy demand, and the integration of renewable sources makes them an indispensable component of modern power generation and industrial energy systems.
The evolving landscape of energy policy and regulations is another factor that further highlights the importance of the operational flexibility of double extraction steam turbines. Many countries and regions have set ambitious targets for reducing greenhouse gas emissions and increasing the share of renewable energy in their power mix. In response to these regulatory changes, industries and power plants are under pressure to adopt cleaner technologies and optimize energy efficiency. Double extraction turbines, with their ability to shift between power and heat generation, offer a pathway to achieving these sustainability goals without sacrificing operational performance or reliability. By reducing waste heat and making full use of the energy generated, these turbines help minimize fuel consumption and decrease overall emissions. Furthermore, their flexibility allows plants to operate within the constraints of evolving environmental regulations, such as emissions caps or carbon pricing systems, without needing to make extensive infrastructure changes.
For example, as the demand for hydrogen as a clean fuel source increases, double extraction turbines can play a pivotal role in utilizing hydrogen in both electricity and heat generation. Hydrogen, being a zero-emission fuel, is expected to become more widespread in various industries, from power generation to heavy transportation. Double extraction turbines can be adapted to run on hydrogen, either exclusively or in combination with other fuels, ensuring that the turbine continues to operate efficiently in a carbon-neutral system. This adaptability allows energy producers to align with global decarbonization trends while still leveraging existing turbine infrastructure.
In addition to their compatibility with various fuels, the ability of double extraction steam turbines to integrate with energy storage systems opens up new possibilities for balancing intermittent renewable energy. Energy storage technologies, such as batteries, thermal storage, or pumped hydro storage, enable energy producers to store excess energy generated during periods of low demand or high renewable output for use during peak periods. By connecting double extraction turbines to these storage systems, plants can ensure that energy is used optimally. For example, during periods of excess solar or wind power generation, the turbine can produce thermal energy, which is stored in thermal storage systems. When renewable generation falls, the stored energy can be utilized to continue providing heat or power. This integration not only enhances system reliability but also maximizes the value of renewable energy, reducing reliance on fossil fuel-based backup generation.
One of the critical elements of operational flexibility for double extraction turbines is their contribution to reducing energy costs. In industries where energy is a significant portion of operational expenses, the ability to adjust energy production based on market conditions or demand fluctuations can result in substantial cost savings. For example, during periods of high electricity prices, the turbine can prioritize power generation to maximize profitability. On the other hand, during times of low electricity prices or when excess renewable energy is available, the turbine can focus on heat production, ensuring that energy is used in the most cost-effective manner. This flexibility allows industries to better manage energy costs, ensuring that their operations remain competitive in markets where energy prices can be volatile.
Additionally, the growing emphasis on energy efficiency and sustainability drives the need for energy systems that can provide a higher level of performance with fewer resources. Double extraction turbines contribute to this goal by optimizing energy generation through efficient steam extraction, minimizing waste heat, and reducing overall fuel consumption. This efficiency is further enhanced by the ability to modulate output based on real-time energy demands, ensuring that the turbine runs at peak efficiency and delivers maximum output with minimal waste. As energy efficiency becomes an ever-more critical focus in global industries, the turbine’s ability to adapt to varying operational conditions ensures that energy systems remain at the forefront of sustainable practices.
The operational flexibility of double extraction turbines also enables them to be a reliable part of backup power systems, particularly in regions that experience unreliable grid conditions or where energy security is a concern. In areas prone to power outages, natural disasters, or other emergencies, double extraction turbines can provide a resilient energy solution. These turbines can be integrated with backup power systems or microgrids, ensuring that energy supply remains uninterrupted even during times of crisis. Their ability to rapidly adjust output to meet fluctuating demand means that they can provide immediate power to critical infrastructure, such as hospitals, emergency services, and manufacturing facilities that rely on a steady supply of energy. Additionally, as part of a larger energy network, the turbine can help balance grid load and ensure that local energy needs are met, even when external power sources are disrupted.
Beyond providing backup power in emergencies, double extraction turbines play a role in maintaining energy security in remote or off-grid areas. In these locations, where access to centralized power generation is limited or unavailable, double extraction turbines can provide a reliable and cost-effective energy source. Whether deployed in industrial or residential settings, these turbines can generate both heat and electricity locally, reducing dependence on external energy providers and increasing self-sufficiency. This is particularly beneficial for remote communities, isolated industries, or areas where energy infrastructure is underdeveloped or vulnerable to disruption.
The continued advancement of digital technologies also enhances the operational flexibility of double extraction steam turbines. With the implementation of advanced analytics, predictive maintenance, and real-time monitoring, operators can gain deeper insights into the performance of turbines and optimize their operation more effectively. Digital tools allow for precise adjustments to turbine settings based on operational data, ensuring that the turbine is always running at optimal conditions. Predictive maintenance systems can also identify potential issues before they result in downtime or costly repairs, allowing operators to address concerns proactively and maintain smooth operation. This advanced level of control further improves the turbine’s flexibility, ensuring it can adapt seamlessly to changing conditions, whether related to fuel quality, pressure fluctuations, or shifts in demand.
In summary, the operational flexibility of double extraction steam turbines is a critical factor in their ability to adapt to the diverse and evolving demands of modern energy systems. Their ability to simultaneously generate power and heat, integrate with renewable energy sources, shift between multiple fuels, and adjust to market conditions makes them a versatile and valuable component of the global energy landscape. As industries and power plants work to achieve greater sustainability, energy efficiency, and cost-effectiveness, the flexibility of these turbines ensures that they remain a key part of the solution. Their role in balancing energy supply, optimizing resource use, and supporting grid stability underscores their importance in meeting the challenges of a low-carbon and decentralized energy future. Whether used for large-scale power generation, cogeneration systems, or emergency backup, the operational flexibility of double extraction steam turbines positions them as a cornerstone of modern energy infrastructure.
Advanced Control Systems of Double Extraction Steam Turbines
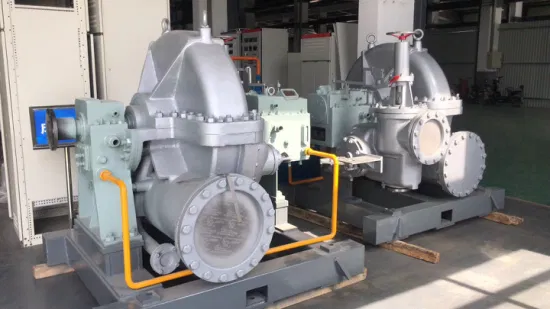
Advanced control systems in double extraction steam turbines have significantly enhanced their efficiency, operational flexibility, and adaptability. As these turbines are increasingly integrated into complex energy systems, the role of advanced control technology has become crucial in ensuring optimal performance across various operational conditions. The advancement of digital control systems has enabled operators to manage turbine performance with unprecedented precision, addressing challenges related to varying energy demands, fuel types, and fluctuating power generation from renewable sources.
At the core of advanced control systems for double extraction steam turbines is real-time monitoring and feedback. These systems rely on a network of sensors distributed throughout the turbine and its associated equipment to collect data on parameters such as pressure, temperature, steam flow, and rotational speed. This data is continuously fed into a central control unit, where algorithms process the information and make real-time adjustments to optimize turbine operation. For example, the system can adjust steam extraction levels, turbine speed, and load distribution to ensure that both the electricity and heat production remain balanced, even as operating conditions change. By utilizing this real-time data, advanced control systems can anticipate fluctuations in demand and adjust turbine performance to avoid inefficiencies or damage.
One of the primary benefits of these advanced control systems is the optimization of steam extraction. In a double extraction steam turbine, steam is extracted at different stages of the turbine to provide thermal energy for industrial heating or district heating systems. The ability to precisely control the amount of steam extracted at each stage is crucial to maximizing the overall efficiency of the turbine while meeting the specific heating demands of the facility. Advanced control systems use sophisticated algorithms to dynamically adjust extraction rates, ensuring that steam is delivered at the correct pressure and temperature for optimal heating while maintaining the turbine’s electrical output. This level of control allows for better integration of the turbine into cogeneration systems, where both power and heat are produced simultaneously, and ensures that neither energy form is produced excessively or insufficiently.
The integration of predictive analytics into advanced control systems further enhances the operation of double extraction turbines. Predictive analytics uses historical performance data, along with real-time input, to forecast turbine behavior and potential issues before they occur. For example, by analyzing patterns in vibration, temperature, and pressure, predictive models can identify early signs of component wear, steam leakage, or other performance degradation. This allows maintenance teams to take proactive action, scheduling repairs before they lead to system failure or unplanned downtime. In this way, predictive analytics extends the operational lifespan of the turbine and reduces the risk of costly repairs or lost production time.
Another important aspect of advanced control systems is their ability to optimize turbine efficiency based on external conditions. Double extraction turbines often operate in environments where factors such as fuel quality, ambient temperature, and pressure fluctuations can impact performance. Advanced control systems are capable of adjusting turbine settings to compensate for these variations, ensuring that the turbine operates at peak efficiency regardless of external changes. For example, if the steam pressure supplied to the turbine drops or if the fuel quality fluctuates, the control system can make real-time adjustments to steam flow, extraction rates, and turbine speed to maintain optimal performance. This adaptability is particularly valuable in systems that rely on multiple fuel types, as the turbine can adjust its operation based on the specific characteristics of each fuel.
In addition to improving efficiency, advanced control systems play a vital role in enhancing the turbine’s overall flexibility. As energy systems become more dynamic and interconnected with renewable energy sources, the ability to adjust turbine performance in response to real-time demand is essential. Advanced control systems allow double extraction turbines to respond to grid conditions, such as fluctuations in renewable energy generation, in a seamless manner. For instance, if there is a sudden drop in wind or solar power generation, the control system can increase the turbine’s power output to compensate for the lost renewable generation, ensuring a stable supply of electricity. Conversely, if excess renewable power is available, the control system can reduce the turbine’s power generation and increase steam extraction for heating purposes, effectively utilizing surplus energy in the most efficient way.
The incorporation of artificial intelligence (AI) and machine learning (ML) algorithms further boosts the capabilities of advanced control systems in double extraction steam turbines. AI and ML can be used to refine the turbine’s performance over time by learning from past operations and continuously adapting to new conditions. These technologies allow control systems to optimize turbine performance in ways that were previously not possible, identifying complex patterns in operational data that human operators might overlook. For example, AI-powered systems can learn to predict load fluctuations and adjust turbine settings in anticipation of changes, reducing the time it takes to respond to shifting energy needs. Furthermore, by continually refining operational parameters, AI-based systems can ensure that turbines operate at maximum efficiency, reducing fuel consumption and emissions while maintaining reliable energy production.
The integration of advanced control systems with wider energy management systems (EMS) is another key development in optimizing the operation of double extraction steam turbines. EMS platforms provide a centralized view of energy generation, storage, and consumption across an entire facility or network, allowing for coordinated management of energy flows. By integrating double extraction turbines into these systems, operators can ensure that the turbine works in harmony with other energy-producing and energy-consuming components, such as combined heat and power (CHP) units, boilers, and energy storage systems. This integration enables advanced load forecasting and dynamic load balancing, allowing the turbine to adjust its operation in real time based on broader system demands. For example, when demand for electricity is low but heating requirements are high, the EMS can signal the turbine to prioritize heat production, ensuring that energy is used efficiently across the entire system.
Furthermore, these control systems are increasingly becoming part of a larger trend toward digitization and remote monitoring in industrial environments. Through secure internet connections and cloud-based platforms, operators can monitor and control double extraction steam turbines remotely, allowing for greater flexibility in managing turbine performance across multiple sites or geographic locations. Remote monitoring also enables operators to perform diagnostics, adjust settings, and receive alerts about potential issues without needing to be physically present. This capability is especially useful in large industrial complexes or energy plants that may have turbines spread across different regions or countries, as it reduces the need for on-site personnel and enhances the overall efficiency of plant operations.
In addition to operational benefits, the use of advanced control systems can also lead to improved compliance with environmental regulations. By precisely controlling emissions and ensuring optimal fuel usage, these systems help operators meet stringent environmental standards while minimizing waste and reducing the carbon footprint of turbine operations. In regions with carbon pricing mechanisms or emissions reduction targets, control systems can help manage turbine operations in a way that minimizes the costs associated with emissions. For example, by adjusting turbine output during periods of high emissions or using cleaner fuels when available, the control system can help ensure that the turbine operates in an environmentally responsible manner while also reducing the financial impact of carbon-based penalties.
Finally, advanced control systems in double extraction steam turbines are key to enhancing safety and reliability. By continuously monitoring critical parameters and implementing automatic shutdown protocols in the event of unsafe conditions, these systems help protect both the turbine and surrounding equipment from damage. For example, if a fault is detected in a turbine component, the control system can immediately shut down the turbine to prevent further damage, avoiding costly repairs or safety hazards. The real-time data collected from sensors also enables operators to perform more accurate risk assessments, making it easier to identify and address potential issues before they escalate into safety concerns.
In conclusion, advanced control systems have transformed the operation of double extraction steam turbines, making them more efficient, flexible, and responsive to a wide range of operational and environmental conditions. By enabling real-time monitoring, predictive maintenance, and integration with wider energy management systems, these systems have enhanced turbine performance and operational reliability. As industries and energy systems become more complex and decentralized, the role of advanced control technology will continue to grow, allowing double extraction turbines to adapt seamlessly to changing demands, optimize energy production, and contribute to sustainable energy solutions. The integration of AI, machine learning, and cloud-based monitoring further extends the capabilities of these control systems, ensuring that double extraction turbines remain a central part of modern energy infrastructure for years to come.
Advanced control systems also play a critical role in ensuring the operational resilience and reliability of double extraction steam turbines, particularly in the face of increasing grid complexities and evolving energy demands. As power grids become more interconnected and decentralized, turbines must be able to adjust their performance in real-time to respond to varying load demands, system disturbances, and fluctuations in power generation from renewable sources. The flexibility to seamlessly adapt to these changing conditions is enabled by advanced control systems that use dynamic control algorithms and feedback loops to continually adjust turbine settings. This capability allows turbines to deliver consistent energy output, whether it’s generating electricity or thermal energy, even when faced with unexpected variations in demand or supply.
Furthermore, advanced control systems contribute significantly to improving overall system efficiency by reducing energy wastage and optimizing fuel consumption. For example, when the demand for heat increases, the system can adjust the turbine’s extraction points to increase heat output while maintaining an efficient level of power generation. This real-time balancing between power and heat ensures that both forms of energy are generated in optimal quantities, helping to avoid waste and reduce fuel consumption. The algorithms used in advanced control systems also make adjustments based on historical data, operational trends, and predictive analytics, helping the turbine to adjust its operational parameters before inefficiencies arise.
This optimization process becomes increasingly important in cogeneration systems where double extraction turbines are utilized to produce both power and thermal energy. In such systems, the challenge lies in simultaneously maximizing electricity output while providing enough steam for industrial heating or district heating needs. The ability of advanced control systems to fine-tune extraction rates and turbine load ensures that energy is efficiently distributed across both outputs without compromising overall performance. By doing so, the system not only meets the needs of industrial and residential consumers but also ensures that energy resources are used to their maximum potential.
Another major aspect of the advanced control system’s contribution to operational efficiency is in optimizing startup and shutdown procedures. Steam turbines are typically designed to operate continuously for long periods, but there are situations in which they must be ramped up or down based on demand, maintenance schedules, or changes in fuel availability. These startup and shutdown processes must be carefully managed to avoid equipment stress, thermal shock, and operational inefficiencies. Advanced control systems facilitate smoother transitions during these periods by precisely controlling steam flow, temperature, and pressure during turbine startup or shutdown. This level of control minimizes wear and tear on turbine components, reduces fuel consumption during ramp-up or ramp-down phases, and helps extend the life of the turbine by ensuring gradual and controlled changes in operating conditions.
Moreover, with the increasing trend of integrating energy storage systems into modern energy networks, advanced control systems for double extraction steam turbines play an essential role in optimizing the interaction between turbines and storage units. Energy storage, whether in the form of batteries, pumped hydro storage, or thermal energy storage, allows excess energy generated during periods of low demand to be stored and used later when demand spikes. Control systems can direct turbines to adjust their output, storing energy during off-peak hours and discharging it when grid demand rises or renewable energy generation falls. This integration helps smooth out the variability of renewable energy sources like wind and solar power, ensuring a more reliable and consistent energy supply.
For example, during periods of high solar generation, when electricity demand is met or exceeded by renewable sources, a turbine can be directed to increase its heat output for district heating or industrial processes, while diverting less steam for power generation. Conversely, during periods when renewable output is low, the turbine can shift focus back to electricity generation, ensuring that energy production continues without interruption. By optimizing this interaction between power generation and energy storage, advanced control systems allow turbines to be more effectively integrated into modern grids that rely on both renewable sources and energy storage to meet their operational demands.
The safety and reliability of double extraction steam turbines are significantly enhanced by the inclusion of advanced control systems. These systems can continuously monitor critical operating parameters, detect potential issues in real-time, and automatically implement corrective actions to mitigate risks. For instance, if the system detects an anomaly in steam pressure or a drop in flow rates, the control system can adjust operating parameters, including opening or closing valves, adjusting extraction levels, or even initiating a controlled shutdown if necessary. By enabling real-time fault detection and automated responses, advanced control systems help prevent mechanical failures, reduce the likelihood of costly repairs, and ensure that the turbine operates within safe parameters.
In addition, with the growing importance of cybersecurity in modern energy infrastructure, advanced control systems for double extraction turbines are increasingly incorporating robust security measures to protect against cyber threats. Since these turbines are often part of larger, interconnected energy networks, any compromise in the control system could lead to disruptions in power generation, equipment damage, or even grid instability. Advanced control systems are equipped with encryption protocols, authentication mechanisms, and other cybersecurity measures to ensure that they remain secure against potential threats. Furthermore, continuous monitoring and anomaly detection capabilities can help identify suspicious activities or attempted intrusions, enabling prompt action to protect the integrity of the turbine and the larger energy system.
The growing demand for sustainability also places pressure on power generation technologies to minimize emissions and operate with lower environmental impacts. Advanced control systems can assist in achieving these sustainability targets by optimizing fuel use, reducing emissions, and increasing overall efficiency. For example, if a turbine is burning fossil fuels, the control system can optimize the combustion process to minimize emissions, such as NOx and CO2, by adjusting steam extraction levels and optimizing burner configurations. Additionally, as the energy industry transitions to cleaner fuels like hydrogen, advanced control systems will play a vital role in ensuring that double extraction turbines can run efficiently on these new fuels, reducing carbon footprints while maintaining performance and reliability.
The flexibility of advanced control systems also supports the integration of renewable energy sources into double extraction turbine systems. As renewable energy generation, such as wind and solar, becomes more widespread, the role of flexible and responsive power systems becomes increasingly important. Advanced control systems allow turbines to respond quickly to fluctuations in renewable output, adjusting steam extraction or power generation to compensate for changes in grid frequency or load. In this way, turbines are able to balance the intermittency of renewable power, smoothing out supply fluctuations and helping to stabilize the grid. This enhances the ability of energy systems to integrate large quantities of renewable energy, contributing to the overall goal of decarbonizing the energy sector.
In conclusion, the role of advanced control systems in double extraction steam turbines has become integral to ensuring operational efficiency, flexibility, safety, and reliability in today’s complex and dynamic energy landscape. These systems enhance turbine performance by allowing for real-time adjustments, optimizing energy generation, and responding to fluctuating demands. They also contribute to sustainability by minimizing emissions, reducing energy wastage, and supporting the transition to cleaner fuels. As energy systems continue to evolve and integrate more renewable sources and energy storage, advanced control systems will remain at the forefront, enabling double extraction turbines to operate seamlessly in this changing environment. By continuously improving control algorithms, integrating predictive maintenance, and ensuring system security, these advanced systems will ensure that double extraction turbines can meet the energy demands of the future with greater efficiency and resilience.
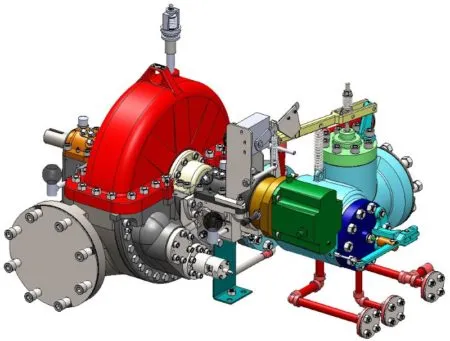
As the energy sector continues to evolve, the capabilities of advanced control systems for double extraction steam turbines will become even more vital in enhancing their operational performance and integration within diverse energy networks. One of the key areas where these systems will make a significant impact is in optimizing the coupling of turbines with emerging technologies, such as smart grids and distributed energy resources (DERs). These advancements allow for a more flexible, responsive energy network that can dynamically manage electricity and heat demands across decentralized systems. With the integration of double extraction turbines into such systems, advanced control systems play a crucial role in harmonizing energy flows between multiple generation sources, including renewables, storage, and conventional power plants.
Smart grids, for instance, rely on real-time data to automatically adjust generation and distribution based on consumer demand and grid conditions. By leveraging the data from advanced control systems, double extraction turbines can quickly respond to fluctuations in grid frequency, power surges, or drops in demand. This dynamic response is especially important in regions with high penetration of variable renewable energy sources like solar and wind power, which can fluctuate unpredictably. The control system can help mitigate the instability caused by these fluctuations, ensuring the turbine can either ramp up or ramp down to provide reliable power while maintaining heat output.
The ability of double extraction turbines to integrate with smart grid systems also enhances grid stability. As renewable energy sources, such as wind and solar, become more prevalent, their intermittent nature often creates challenges in maintaining grid frequency and voltage. Advanced control systems in double extraction turbines can help stabilize the grid by providing ancillary services like frequency regulation or voltage support. The turbine’s response to these grid signals is automated through real-time adjustments, ensuring that the system remains stable even during periods of high variability in renewable power generation. This level of flexibility helps prevent grid congestion and minimizes the risk of power outages or disruptions.
One of the key advantages of advanced control systems in this context is their ability to seamlessly interface with various forms of energy storage systems. Energy storage plays an increasingly important role in balancing the supply and demand of electricity, especially when renewable generation is intermittent. By working in tandem with energy storage systems, double extraction turbines can use stored energy during periods of low renewable generation to continue providing electricity or heat. During times when renewable energy generation is high, the control system can direct the turbine to extract more steam for heating or reduce power output to store the excess energy. This complementary relationship between turbines and storage systems helps to smooth out supply-demand imbalances and supports the broader goal of achieving grid decarbonization.
Another area where advanced control systems will be critical in the future is in ensuring the turbine’s ability to work with a broader range of fuels, including low-carbon and renewable alternatives. As the energy transition accelerates, the demand for cleaner fuels, such as hydrogen, ammonia, and biofuels, will increase. Double extraction turbines must be adaptable to work with these new fuel types while maintaining operational efficiency. Advanced control systems are instrumental in enabling this flexibility by allowing the turbine to adjust its parameters depending on the fuel characteristics. For example, hydrogen, which has a different combustion profile than traditional fossil fuels, may require adjustments in steam flow, combustion air, and exhaust systems. The control system can detect these changes in real time and adjust the turbine’s operation accordingly, ensuring that the turbine operates optimally with minimal emissions.
As industries and utilities continue to prioritize sustainability, reducing greenhouse gas emissions is an ever-present goal. Advanced control systems are key enablers in achieving these targets for double extraction steam turbines. By optimizing the combustion process, reducing fuel consumption, and improving steam extraction efficiency, these systems help minimize CO2 emissions. The ability to fine-tune turbine operations also reduces the production of other pollutants, such as nitrogen oxides (NOx), particulate matter, and sulfur dioxide, all of which are byproducts of traditional fossil fuel combustion. This is particularly important in regions where stringent environmental regulations are in place or are expected to become more stringent in the future.
Moreover, as part of the broader trend toward digitalization in the energy sector, the incorporation of artificial intelligence (AI) and machine learning (ML) algorithms within advanced control systems is expected to play a significant role in improving turbine performance. AI and ML can continuously learn from historical data, real-time operations, and even predictive models to optimize turbine settings. These algorithms can identify patterns in energy production and consumption that are difficult for human operators to detect, allowing for more precise adjustments to turbine performance. Over time, the AI-driven control systems will become even more efficient at managing operations, further reducing energy waste, improving fuel efficiency, and enabling better integration with renewable energy sources.
With the growing focus on energy resilience, advanced control systems will also play an essential role in disaster recovery and business continuity planning. In regions susceptible to natural disasters such as hurricanes, floods, or earthquakes, the ability to quickly restore power generation is critical. Double extraction turbines, with their advanced control systems, can contribute to faster recovery times by quickly adjusting to fluctuating power demands or by integrating with emergency backup systems. The ability of these systems to predict potential disturbances and initiate corrective actions before a failure occurs is a key advantage in ensuring that turbines remain operational in challenging circumstances.
In addition to physical resilience, these control systems can improve the cybersecurity of turbine operations. As energy systems become more digitized and interconnected, they become more vulnerable to cyber threats. Advanced control systems incorporate sophisticated security measures to ensure that only authorized personnel can access and control turbine settings. These security protocols help prevent malicious attacks that could disrupt turbine operation or even damage critical infrastructure. The integration of encryption, intrusion detection, and real-time threat monitoring ensures that the turbine remains secure against cyber threats, making it a reliable and trusted component of modern energy infrastructure.
Finally, the integration of advanced control systems into double extraction steam turbines is not limited to power generation plants but extends to industrial applications as well. Many industries, including chemical, manufacturing, and food processing, rely on cogeneration systems to meet both their power and heat needs. The ability to efficiently control steam extraction and adjust turbine output based on real-time demands provides industrial facilities with a significant operational advantage. By utilizing advanced control systems, these industries can minimize energy costs, reduce emissions, and enhance overall operational efficiency. The flexibility offered by these systems also enables industries to respond quickly to changing production schedules or market demands, ensuring continuous and reliable energy supply for critical processes.
In conclusion, the future of double extraction steam turbines is closely tied to the continued advancement of control systems. These systems provide the flexibility, precision, and intelligence necessary to optimize turbine operations in increasingly complex and dynamic energy networks. Whether it’s integrating with renewable energy sources, optimizing fuel consumption, enhancing efficiency, or ensuring grid stability, advanced control systems will be pivotal in meeting the growing demand for sustainable and resilient energy solutions. As energy systems continue to evolve, the integration of AI, machine learning, and real-time data analytics will further empower operators to achieve greater operational performance and sustainability, ensuring that double extraction turbines remain at the forefront of modern energy technologies.
As energy systems evolve and demand for efficient, flexible, and sustainable power solutions increases, the role of advanced control systems in double extraction steam turbines will continue to expand. One of the primary challenges that double extraction turbines face is the increasing complexity of integrating them with diverse energy sources and systems. These turbines, which operate in both conventional and renewable-driven power generation environments, must adapt to varying supply and demand patterns. Advanced control systems are integral in managing these challenges, providing the necessary intelligence and responsiveness to optimize performance across all operating conditions.
The integration of renewable energy sources, particularly wind and solar, introduces a level of variability in power generation that must be carefully managed to ensure grid stability. Double extraction steam turbines can complement renewable energy generation by providing grid balancing services. Advanced control systems play a critical role here by dynamically adjusting the turbine’s operation to compensate for fluctuations in renewable power. When renewable energy production exceeds demand, turbines can reduce their electrical output, prioritizing heat extraction for district heating or industrial processes. Conversely, during times of low renewable generation, the control system can increase the turbine’s power output, helping to stabilize the grid and ensuring a consistent energy supply. This responsive operation enables double extraction turbines to act as flexible energy providers, capable of adjusting to the changing mix of renewable and conventional generation in real-time.
In addition to grid balancing, the ability to manage both power and heat production simultaneously is a core advantage of double extraction steam turbines in cogeneration applications. Advanced control systems enable turbines to optimize this dual-purpose function by ensuring that power and thermal energy are produced at the most efficient levels based on demand. This flexibility is particularly valuable in industrial applications, where both electricity and process heat are required. The control system can make real-time decisions about how to allocate steam between power generation and heat extraction, ensuring that the turbine’s operation remains balanced and efficient.
The control systems’ adaptability is also critical when turbines are retrofitted to work with new fuel types, which are becoming more common in the energy transition. As the global energy sector moves away from fossil fuels and adopts cleaner, more sustainable alternatives like hydrogen, biofuels, and synthetic fuels, the advanced control systems must be capable of adjusting turbine operation to accommodate these changes. Hydrogen, for example, has a different combustion profile compared to natural gas or coal, and this can impact how steam is produced and extracted from the turbine. Advanced control systems monitor the fuel quality and adjust the combustion process, steam flow, and temperature to ensure the turbine operates optimally while minimizing emissions. This versatility in fuel usage makes double extraction steam turbines highly adaptable to evolving energy markets and regulatory requirements.
As part of the push for carbon-neutral energy systems, the demand for decarbonization in industrial and power generation processes is growing. Double extraction steam turbines, with the help of advanced control systems, can contribute to this goal by improving overall system efficiency and reducing fuel consumption. The control system’s ability to optimize fuel usage, minimize energy waste, and adjust extraction rates to maximize heat recovery all contribute to a more sustainable operation. In industries where thermal energy recovery is critical, such as chemical manufacturing or food processing, the efficient use of steam for both power generation and heating can significantly reduce the overall carbon footprint of the operation.
Advanced control systems also play a pivotal role in ensuring the long-term reliability and lifespan of double extraction steam turbines. These systems use predictive maintenance technologies to monitor the health of critical components, such as bearings, valves, and turbines themselves, identifying early signs of wear and tear before they lead to significant issues or system failures. By leveraging real-time data from sensors and historical performance trends, the control system can predict when maintenance is needed, schedule downtime, and ensure that parts are replaced before they fail. This predictive approach to maintenance reduces unplanned outages, minimizes repair costs, and extends the operational life of the turbine. It also helps prevent safety incidents caused by mechanical failure, ensuring that turbines operate within safe and reliable parameters at all times.
Furthermore, these advanced systems enable remote monitoring and control, which is particularly valuable in large-scale operations or in geographically dispersed installations. Remote access to turbine operations allows for centralized management and oversight, enabling operators to monitor performance and make adjustments without the need for on-site presence. In cases where turbines are located in remote or offshore locations, this capability is crucial for ensuring efficient operations and minimizing operational disruptions. The ability to perform diagnostics, adjust operational parameters, and address potential issues remotely ensures that turbines can continue to run optimally with less downtime and intervention, which is especially valuable in critical infrastructure settings.
The integration of AI and machine learning into advanced control systems also enhances the ability of double extraction turbines to adapt to changing operational conditions. By analyzing vast amounts of operational data, AI and machine learning algorithms can identify patterns and correlations that help optimize turbine performance. These algorithms can predict potential efficiency losses, adjust settings to avoid inefficient operational states, and continuously refine the system’s performance over time. The more data the system collects, the better it becomes at optimizing operations in real-time, ensuring that turbines operate at their highest efficiency levels under all conditions.
As energy networks become more interconnected, the ability of advanced control systems to work in concert with other components of the energy infrastructure will become even more important. For instance, double extraction steam turbines may be part of a broader integrated system that includes combined heat and power (CHP) plants, renewable energy generators, and energy storage facilities. In such an environment, advanced control systems can act as the brain of the entire system, coordinating the actions of all components to ensure that power and heat are distributed efficiently across the network. This holistic approach to energy management reduces waste, increases system reliability, and ensures that the overall system operates in harmony to meet both energy demand and environmental goals.
Another advantage of advanced control systems is their ability to handle complex grid dynamics, including frequency regulation, load following, and demand response. In modern grids, where the balance between supply and demand is more fluid due to the influence of intermittent renewable generation, turbines must be capable of adjusting their output in real-time to maintain grid stability. Advanced control systems ensure that turbines can quickly respond to changes in grid frequency, providing ancillary services like voltage support and reactive power, which are essential for maintaining grid integrity. This capability allows double extraction turbines to contribute to the grid’s reliability, even as renewable sources become a larger part of the energy mix.
Moreover, these systems can enable the integration of distributed energy resources (DERs), which are increasingly being adopted in modern power systems. DERs, such as small-scale solar, wind, and battery systems, are often located at the point of consumption rather than in centralized power plants. Advanced control systems allow double extraction turbines to interact with these smaller, decentralized systems by managing the flow of energy between them, ensuring that power is dispatched where it is needed most. This capability is particularly important in microgrids, where local energy generation and storage must be closely coordinated to ensure reliable power supply in off-grid or remote locations.
The growing importance of energy efficiency and environmental sustainability is driving innovation in turbine control systems. Advanced control systems allow double extraction turbines to not only optimize fuel consumption but also reduce harmful emissions, which is critical in meeting global environmental standards. By adjusting operating parameters, controlling exhaust emissions, and ensuring that combustion is as efficient as possible, these systems contribute to a cleaner energy future. As the world moves toward stricter environmental regulations and carbon reduction targets, the ability of turbines to operate in an environmentally responsible manner will be a key factor in their continued viability.
In conclusion, advanced control systems are essential for ensuring the continued success and relevance of double extraction steam turbines in modern energy systems. These systems enable turbines to operate more efficiently, respond dynamically to fluctuating power demands, integrate with renewable energy sources, and optimize fuel use to meet sustainability goals. By leveraging predictive maintenance, AI, machine learning, and remote monitoring, these control systems enhance turbine performance, reduce downtime, and extend the operational life of the equipment. As the energy sector continues to evolve, the integration of double extraction steam turbines with advanced control systems will play a central role in achieving a flexible, resilient, and sustainable energy future.
Extraction Back-pressure Turbines
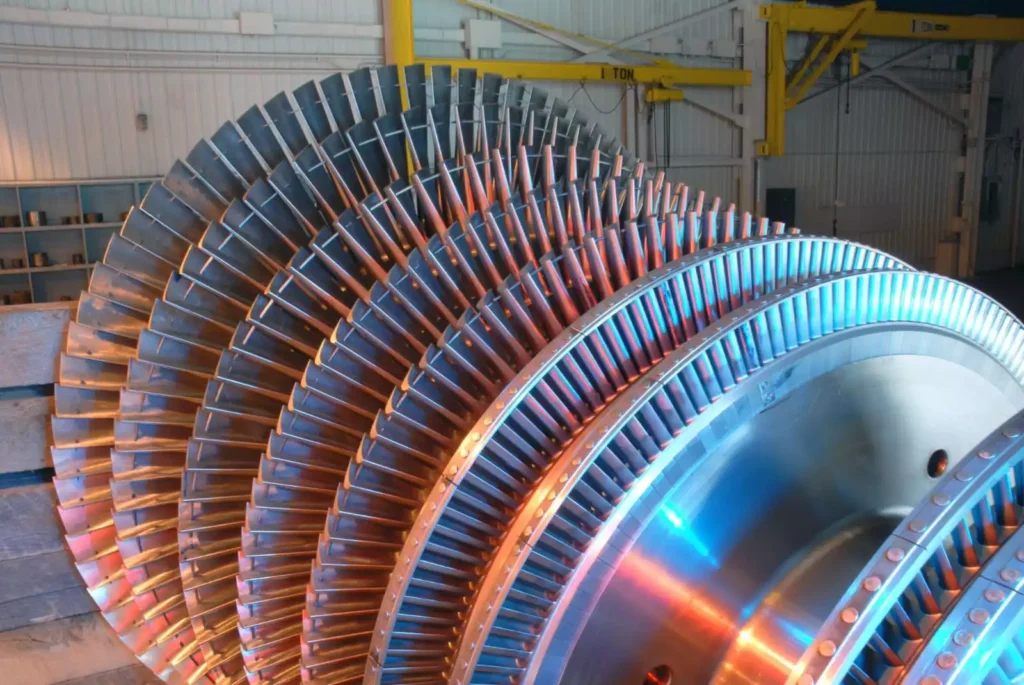
Extraction back-pressure steam turbines (EBTs) are widely used in power generation and industrial applications due to their ability to provide both electrical power and process steam in a highly efficient manner. These turbines operate by extracting steam from intermediate stages for use in various industrial processes, while the remaining steam continues through the turbine to generate power. The flexibility of extraction back-pressure turbines makes them particularly valuable in combined heat and power (CHP) systems, cogeneration, and district heating applications. The advanced control systems of these turbines are integral to their operation, ensuring both optimal performance and flexibility, enabling them to adapt to a range of operating conditions while maintaining efficiency and safety.
Advanced control systems for extraction back-pressure turbines help to monitor and optimize the extraction of steam for process applications while maintaining the turbine’s ability to generate electricity. These control systems are designed to balance the two functions of power generation and heat supply, which can fluctuate depending on external factors like demand changes, ambient conditions, or variations in the steam supply. Through real-time adjustments, the control system ensures that the turbine operates at its most efficient state, extracting the right amount of steam for industrial processes while maintaining an optimal level of power output. This ability to balance heat and power generation based on demand makes extraction back-pressure turbines an excellent choice for industries that require consistent thermal energy and electricity.
The adaptability of extraction back-pressure turbines is largely driven by the sophistication of their control systems, which are capable of responding to changes in process steam requirements and electrical load. In industries like chemical processing, food production, and paper manufacturing, where process steam is critical for production operations, the extraction of steam must be carefully controlled. The control system continuously monitors the pressure, temperature, and flow rates of steam at various extraction points to ensure that the desired thermal energy is available when needed, without compromising turbine efficiency or stability. In response to varying heat demand, the system can adjust the extraction levels to deliver the required steam while optimizing the turbine’s power generation capability.
One of the key advantages of extraction back-pressure turbines lies in their ability to integrate seamlessly into energy networks that rely on renewable energy sources. In regions with high renewable energy penetration, such as wind and solar, fluctuations in power generation can cause challenges in maintaining grid stability. Extraction back-pressure turbines, equipped with advanced control systems, provide a flexible solution to this problem by adjusting their output based on real-time grid conditions. For example, during periods of high renewable generation, when the demand for electricity may be lower, the turbine can prioritize the extraction of steam for industrial or district heating purposes. Conversely, when renewable energy generation is low, the control system can increase the turbine’s electrical output to help stabilize the grid and meet energy demands.
The advanced control systems in extraction back-pressure turbines enable better load management and the ability to operate in a variety of configurations to meet specific power and heat requirements. The flexibility of these systems allows them to respond to shifts in both electricity demand and process steam demand. For instance, during periods of low electricity demand, the turbine can shift more focus to steam extraction, supplying additional heat to processes that require consistent thermal energy. This operational flexibility helps to avoid waste by ensuring that the turbine always operates in the most efficient way possible, taking full advantage of available heat and power resources. In cogeneration systems, this adaptability is crucial for maintaining energy efficiency and maximizing the value of every unit of fuel consumed.
Another critical aspect of advanced control systems for extraction back-pressure turbines is their role in ensuring operational reliability and safety. These turbines often operate in high-stress industrial environments, where equipment failure or unplanned downtime can result in significant production losses. Advanced control systems continuously monitor key parameters such as steam pressure, temperature, and flow rate, and adjust the turbine’s operation accordingly to maintain safe and efficient performance. In the event of a deviation from normal operating conditions—such as an increase in steam pressure or a drop in extraction flow—the control system can initiate automatic protective actions, such as reducing power output or adjusting extraction levels, to avoid damage to the turbine or other components.
Predictive maintenance is another significant benefit of advanced control systems for extraction back-pressure turbines. By using real-time data and predictive analytics, these systems can identify potential issues before they become critical, reducing the likelihood of equipment failure and minimizing costly repairs or unplanned shutdowns. For example, advanced sensors and diagnostic tools can detect early signs of wear in critical components, such as the bearings or seals, and alert operators to perform maintenance before a failure occurs. This proactive approach ensures that the turbine remains in peak operating condition for longer periods, improving reliability and reducing the need for emergency interventions.
Furthermore, as extraction back-pressure turbines are increasingly being used in combined heat and power (CHP) systems, the role of advanced control systems in optimizing overall system performance becomes even more vital. In a CHP system, both electricity and thermal energy must be produced in the right proportions to meet demand. The control system must continuously adjust the turbine’s operation, balancing the extraction of steam for industrial or heating purposes with the need to generate electricity. This dynamic adjustment ensures that the system remains flexible and can meet both heat and power demands without wasting energy or resources. It also allows for greater integration of renewable energy sources, as the turbine can adjust its output to complement renewable generation when it is available and scale up power production when demand or grid conditions require it.
Energy storage systems, which are becoming more prevalent in modern grids, can also benefit from the advanced control systems of extraction back-pressure turbines. Energy storage allows for excess energy to be stored during periods of low demand and released when grid demand peaks. In some cases, turbines can be coupled with energy storage systems to provide additional grid balancing services. For example, when there is excess renewable energy on the grid, the turbine can be directed to extract more steam for industrial heating or district heating, while any excess electricity generated can be stored for later use. When renewable generation drops or grid demand rises, the turbine can ramp up power production, drawing on the stored energy to maintain grid stability. This interaction between turbines and storage systems enhances the flexibility of the overall energy network, allowing it to better accommodate renewable generation fluctuations.
Another important contribution of advanced control systems in extraction back-pressure turbines is their ability to integrate with smart grid technologies. As grids become smarter and more interconnected, the need for real-time monitoring, control, and decision-making becomes even more important. Advanced control systems enable turbines to communicate with other grid components, such as renewable generators, energy storage systems, and distribution networks. This connectivity allows for better coordination between power generation and demand, ensuring that the right mix of energy resources is used at the right time. The turbine can automatically adjust its output based on real-time data from the grid, helping to maintain balance and stability. This seamless integration with smart grids not only optimizes the turbine’s performance but also enhances the overall efficiency and reliability of the energy system.
As environmental regulations become more stringent, particularly regarding emissions, the advanced control systems in extraction back-pressure turbines are becoming increasingly important in helping operators meet sustainability goals. These control systems can adjust the combustion process, optimize fuel consumption, and manage exhaust emissions to ensure compliance with air quality standards. For instance, in some regions, there are regulations in place that limit the amount of NOx or particulate matter that can be emitted by power generation equipment. The control system can monitor emissions levels and adjust combustion parameters to reduce emissions, ensuring that the turbine operates within the prescribed limits. Furthermore, with the growing shift toward cleaner fuels, such as hydrogen, the control system must be capable of adjusting to different fuel profiles and ensuring efficient combustion without increasing emissions.
As industries seek to decarbonize their operations, extraction back-pressure turbines, with their advanced control systems, provide a flexible and efficient solution for meeting both power and thermal energy demands while minimizing environmental impact. By optimizing the extraction of steam, reducing fuel consumption, and improving overall system efficiency, these turbines help reduce greenhouse gas emissions and support the broader transition to a low-carbon energy future.
In conclusion, extraction back-pressure steam turbines, equipped with advanced control systems, offer significant advantages in terms of operational efficiency, flexibility, safety, and sustainability. These systems enable turbines to adapt to fluctuating demands for power and process steam, ensuring that both heat and electricity are generated efficiently and sustainably. Through real-time monitoring, predictive maintenance, and seamless integration with smart grids and energy storage systems, advanced control systems enhance the reliability and performance of extraction back-pressure turbines. As the energy sector continues to evolve, these systems will remain essential in enabling turbines to meet the growing demands of industrial, commercial, and residential energy consumers while reducing environmental impacts and supporting the transition to a greener energy future.
The role of advanced control systems in extraction back-pressure steam turbines is becoming even more critical as industries and power generation systems continue to evolve towards greater efficiency and sustainability. As the global energy landscape shifts towards decarbonization and the integration of renewable energy sources, the ability of these turbines to remain adaptable and flexible becomes a key factor in their continued success. The advanced control systems that govern the operation of extraction back-pressure turbines are designed to optimize performance in a variety of scenarios, ensuring that the turbines can provide a steady and reliable supply of both electrical power and process steam, while also reducing fuel consumption and minimizing emissions.
One of the core functions of these advanced control systems is to enhance operational efficiency through real-time monitoring and adjustments. Extraction back-pressure turbines are often integrated into complex energy systems where both electricity and steam are needed simultaneously. These systems may include industrial processes, district heating networks, or combined heat and power (CHP) applications, where the demand for thermal energy fluctuates depending on production schedules, weather conditions, and seasonal changes. The control system ensures that the turbine is operating at peak efficiency, making continuous adjustments to the steam extraction process to meet the thermal energy needs of the system while also maintaining electrical power generation. This dual optimization of heat and power is essential for maximizing the overall efficiency of the energy system.
The ability to integrate extraction back-pressure turbines with renewable energy sources, such as wind, solar, or geothermal, is another area where advanced control systems play a vital role. In systems where renewable energy sources are intermittent, advanced control systems allow the turbine to adapt its operation to fluctuations in renewable power generation. When renewable energy production exceeds demand, the control system can shift the turbine’s focus to steam extraction, providing additional thermal energy for industrial processes or district heating. Conversely, when renewable energy generation drops, the control system can adjust the turbine’s operation to increase electrical power output, ensuring that grid stability is maintained and that power demand is met efficiently.
Beyond optimizing for renewable energy integration, the advanced control systems in extraction back-pressure turbines also play a key role in improving grid flexibility and energy security. With the growing complexity of modern power grids, characterized by diverse energy sources, storage systems, and demand-side management strategies, turbines must be able to quickly respond to changing grid conditions. Advanced control systems enable the turbine to adjust its output rapidly, balancing the generation of electricity with the extraction of steam to meet the dynamic needs of the grid and local industrial applications. This flexibility helps to stabilize the grid, particularly during times of peak demand or when renewable generation is low, by ensuring a reliable and responsive power and heat supply.
The integration of predictive maintenance capabilities into advanced control systems further enhances the reliability and longevity of extraction back-pressure turbines. By using sensors and machine learning algorithms, these systems continuously monitor the turbine’s key components, such as the bearings, valves, and seals. They can detect early signs of wear or malfunction, predicting when maintenance is required before a failure occurs. This approach reduces the risk of unplanned downtime, minimizes repair costs, and extends the operational life of the turbine. Additionally, predictive maintenance allows operators to schedule repairs during planned maintenance windows, reducing the impact on production schedules and avoiding costly disruptions.
In terms of operational safety, advanced control systems in extraction back-pressure turbines are designed to protect both the equipment and personnel. Steam turbines operate under high-pressure conditions, and any deviations from normal operating parameters—such as pressure imbalances, overheating, or excessive vibration—can lead to catastrophic failure or safety hazards. The control system continuously monitors these parameters and, if necessary, activates automatic safety protocols to prevent accidents. For example, if there is an unexpected pressure rise, the system can initiate shutdown procedures, adjust extraction rates, or trigger safety valves to protect the turbine and the surrounding infrastructure. These safety features are critical in maintaining the reliability of the turbine, particularly in industries where process steam is a vital part of daily operations.
The integration of extraction back-pressure turbines into district heating systems, which are increasingly popular in urban areas, highlights the importance of advanced control systems in managing both thermal energy and power generation. In these systems, the turbine must adjust its operation based on demand from both the electrical grid and the district heating network. The advanced control system ensures that the turbine can simultaneously provide the necessary electricity to the grid while also supplying process steam to the district heating system. The ability to balance both power and heat generation efficiently is essential for reducing fuel consumption and lowering emissions, as it helps to optimize the overall energy use in urban environments.
As industries and governments continue to focus on reducing carbon emissions, the role of extraction back-pressure turbines in supporting sustainability efforts will become even more critical. Advanced control systems can adjust the turbine’s operation to minimize fuel consumption, optimize efficiency, and reduce harmful emissions. By optimizing steam extraction, improving combustion processes, and managing exhaust emissions, these systems help to ensure that the turbine meets stringent environmental standards. Additionally, as the energy transition moves towards cleaner fuels, such as hydrogen or biogas, the advanced control systems in extraction back-pressure turbines will need to adapt to these new fuel types, optimizing combustion and ensuring that emissions remain within acceptable limits.
In conclusion, the role of advanced control systems in extraction back-pressure steam turbines is crucial for maximizing operational efficiency, ensuring safety, and enhancing the flexibility of energy systems. These systems enable turbines to adapt to varying power and heat demands, integrate renewable energy sources, and respond to grid fluctuations, all while minimizing fuel consumption and emissions. As the energy sector continues to evolve, extraction back-pressure turbines, with their advanced control systems, will remain a key component in achieving a sustainable, efficient, and flexible energy future. Their ability to provide both electricity and thermal energy, combined with the benefits of real-time optimization and predictive maintenance, positions them as a vital tool in the transition to a low-carbon and resilient energy infrastructure.
As the role of advanced control systems in extraction back-pressure steam turbines continues to expand, their contributions to energy systems grow increasingly significant in achieving operational flexibility and cost-effectiveness. These systems not only optimize the turbine’s operation but also enable its integration with broader energy management strategies, supporting both industrial and residential energy demands. The continued development and refinement of control technologies will enhance the ability of these turbines to adapt to new challenges in the energy sector, such as fluctuating fuel prices, stricter emissions regulations, and the growing demand for renewable energy.
One of the critical areas where advanced control systems make a substantial difference is in managing fuel efficiency. Traditional power generation systems can suffer from fuel waste and inefficiency, especially when the demand for electricity and thermal energy fluctuates. Advanced control systems, however, can monitor steam conditions, combustion efficiency, and turbine output in real-time. By continuously optimizing the fuel combustion process, these systems ensure that the turbine operates at maximum efficiency, minimizing fuel consumption while still meeting both power and steam requirements. This becomes particularly beneficial in industries where high energy costs can significantly impact the bottom line, such as chemical manufacturing, steel production, and refining.
The integration of energy storage technologies is another area where advanced control systems shine. Many modern energy systems combine extraction back-pressure turbines with battery storage or thermal storage units to address the intermittency of renewable energy sources. During periods when renewable energy generation is high, excess energy can be stored, allowing the turbine to focus more on steam extraction and less on power generation. During times when renewable generation is low, the turbine can ramp up electricity production to meet grid demand, drawing on the stored energy to maintain a stable supply. This ability to integrate with energy storage not only enhances the system’s flexibility but also optimizes the use of available renewable resources, helping to reduce overall system costs and improve carbon emissions performance.
Additionally, as energy systems move towards greater decentralization with the advent of microgrids and distributed energy resources (DERs), the role of advanced control systems in extraction back-pressure turbines will evolve further. Microgrids, which often operate independently or in conjunction with larger utility grids, require high levels of flexibility and real-time monitoring to ensure they can balance energy supply and demand effectively. Advanced control systems enable turbines to communicate with microgrid controllers, allowing for dynamic adjustments in turbine operation. This flexibility is crucial for maintaining the stability of the microgrid, ensuring that both power and thermal energy are delivered to end-users without interruption, regardless of local fluctuations in renewable energy generation or fuel availability.
Moreover, as global regulations on greenhouse gas emissions become more stringent, advanced control systems are vital in helping industries comply with emission reduction targets. Many extraction back-pressure turbines are already equipped with technologies that optimize combustion, reduce NOx emissions, and lower particulate matter in exhaust gases. These systems also enable real-time monitoring of emission levels, allowing operators to adjust combustion parameters to ensure that the turbine remains within regulatory limits. Furthermore, control systems can help turbines adjust their operations to run on cleaner fuels, such as hydrogen or biofuels, ensuring that the transition to a low-carbon economy does not compromise energy efficiency or power generation capacity.
Advanced control systems also help improve the overall reliability of extraction back-pressure turbines by enabling predictive diagnostics. These systems continuously gather data from sensors placed throughout the turbine to monitor performance and identify any potential issues before they cause a failure. For example, advanced sensors can detect unusual vibrations, temperature changes, or pressure fluctuations that may signal mechanical wear, impending failures, or blockages. By identifying these issues early, predictive maintenance systems enable operators to address potential problems before they lead to unscheduled downtime, thereby minimizing costly repairs and improving turbine longevity. This predictive capability is especially important in industries where turbines operate continuously and are critical to maintaining production schedules, such as in chemical plants, paper mills, or food processing.
In addition to enhancing reliability, advanced control systems also contribute to turbine safety. Steam turbines, particularly those used in industrial applications, operate under high pressure and temperatures, posing potential risks to equipment and personnel. The real-time monitoring capabilities of modern control systems enable operators to quickly identify any unsafe operating conditions, such as pressure or temperature imbalances, and take corrective action before accidents occur. If an anomaly is detected, the system can automatically trigger safety protocols, such as reducing power output, shutting down the turbine, or adjusting steam extraction rates to alleviate pressure. By ensuring that the turbine is always operating within safe parameters, these systems help prevent catastrophic failures, protect workers, and reduce the risk of damage to expensive equipment.
Furthermore, as the energy sector continues to embrace digitalization, the integration of advanced control systems with digital twin technology is becoming more prevalent. A digital twin is a virtual model of a physical system, such as a steam turbine, that uses real-time data to simulate the turbine’s operation and performance. By incorporating digital twin technology into control systems, operators gain deeper insights into turbine performance and can make more informed decisions. The digital twin provides a detailed, data-driven representation of the turbine’s operation, enabling simulations that can predict how the turbine will respond to different scenarios, such as changes in steam flow or fuel type. This allows operators to proactively adjust turbine operations, optimize energy output, and minimize operational risks without waiting for real-time feedback.
Another emerging trend in the field of advanced control systems is the use of artificial intelligence (AI) and machine learning to further enhance the performance of extraction back-pressure turbines. By analyzing vast amounts of operational data, AI algorithms can identify patterns and trends that human operators may miss. For instance, machine learning models can predict future load demands based on historical data and weather forecasts, enabling the turbine’s control system to anticipate changes in energy needs and adjust its operations in advance. This level of predictive intelligence can greatly improve the turbine’s efficiency, reduce fuel consumption, and ensure that energy production is always aligned with demand. Additionally, AI-based control systems can be used to optimize turbine performance across entire fleets of turbines, identifying operational inefficiencies and recommending improvements based on the aggregated data of multiple turbines.
As industries continue to innovate and adopt more sustainable practices, the need for more flexible and efficient power generation solutions will only increase. Extraction back-pressure turbines, with their ability to simultaneously produce electrical power and process steam, will play an increasingly important role in meeting these needs. The integration of advanced control systems that optimize energy production, improve safety, enable predictive maintenance, and facilitate renewable energy integration will ensure that these turbines remain at the forefront of energy generation technology. By leveraging the latest advancements in control system design, operators can unlock new levels of efficiency and performance, contributing to a more sustainable and resilient energy future.
In conclusion, the role of advanced control systems in extraction back-pressure steam turbines is essential for optimizing their performance, enhancing safety, and enabling operational flexibility. These systems ensure that turbines operate efficiently, adapt to changing energy demands, integrate renewable energy sources, and maintain high levels of reliability and safety. As the global energy landscape evolves and the demand for cleaner, more efficient energy solutions grows, the continued development of advanced control systems will be crucial for maximizing the potential of extraction back-pressure turbines and ensuring that they remain a cornerstone of modern energy systems.
As the energy landscape evolves, the capabilities of advanced control systems in extraction back-pressure turbines will continue to expand, responding to new challenges such as stricter environmental standards, the integration of more variable renewable energy sources, and the increasing demand for energy resilience and security. These systems are becoming more sophisticated, with the integration of cutting-edge technologies like artificial intelligence (AI), machine learning (ML), and predictive analytics, which are enhancing the turbines’ efficiency, reliability, and adaptability.
One of the primary benefits of AI and ML in the control systems of extraction back-pressure turbines is their ability to predict performance and identify inefficiencies before they lead to problems. These technologies analyze vast amounts of operational data, including steam flow, temperature, pressure, and fuel consumption, to detect patterns that humans might not recognize. By learning from historical data, the system can predict future turbine performance, adjusting the operation to maximize efficiency and reduce unnecessary fuel consumption. For example, if the system detects that a specific operating condition is leading to suboptimal efficiency, it can automatically adjust parameters such as steam extraction or fuel mix to optimize performance. This predictive capability also extends to maintenance, where the control system can forecast when parts of the turbine might need servicing or replacement, preventing unplanned downtime and reducing the costs associated with emergency repairs.
Beyond operational efficiency, AI and machine learning also improve the ability of turbines to respond to external conditions, such as fluctuations in grid demand or the availability of renewable energy. As more renewable energy sources, such as wind and solar, are integrated into the grid, the demand for power becomes more variable. Advanced control systems in extraction back-pressure turbines can respond in real-time to these fluctuations, adjusting turbine output to complement the variability of renewable generation. During periods of high renewable energy production, for example, the system can focus more on steam extraction for industrial use or district heating, while minimizing electricity generation. When renewable production falls, the turbine can ramp up electrical output to fill the gap. This level of flexibility not only helps to stabilize the grid but also maximizes the utilization of both renewable and conventional energy sources, reducing reliance on fossil fuels and decreasing greenhouse gas emissions.
The role of advanced control systems in extraction back-pressure turbines also supports grid stability in distributed energy systems. As energy generation becomes more decentralized with microgrids, advanced control systems enable turbines to operate independently or in coordination with other distributed energy resources. Microgrids, which can operate autonomously or in parallel with the main grid, require real-time balancing of electricity and heat supply to meet local demand. Extraction back-pressure turbines, equipped with advanced control systems, are able to adjust their output in response to microgrid requirements, whether that means prioritizing steam extraction for industrial processes or increasing electrical output for local grid stabilization. This flexibility ensures that local energy systems remain reliable and efficient, even as they integrate more diverse energy sources.
In addition to their ability to manage grid variability and decentralization, advanced control systems also provide a solution to the increasing demand for energy storage. Energy storage systems, such as batteries or thermal storage units, are becoming more critical in balancing the intermittent nature of renewable generation. Extraction back-pressure turbines can be integrated with these storage systems, using their control systems to adjust turbine operation based on the availability of stored energy. For instance, when excess renewable energy is available, it can be stored, allowing the turbine to focus on steam extraction for industrial or district heating applications. When energy storage is needed to supply electricity during periods of low renewable generation, the turbine can increase its electrical output to ensure that grid demand is met. This dynamic response to energy storage needs helps reduce reliance on fossil fuel-based backup generation and supports a more sustainable energy system.
The increasing importance of sustainability and decarbonization has made advanced control systems in extraction back-pressure turbines an essential tool for meeting environmental goals. As industries and utilities strive to reduce their carbon footprints, advanced control systems can help minimize fuel consumption, optimize combustion processes, and reduce harmful emissions. For example, these systems can manage the turbine’s combustion process to reduce NOx and CO2 emissions by adjusting fuel injection, air-fuel ratios, and other parameters. In addition, as clean fuels such as hydrogen or synthetic gases become more viable, advanced control systems will be essential for ensuring the efficient combustion of these alternative fuels. By making real-time adjustments to optimize combustion, these systems ensure that emissions remain within regulatory limits while still maintaining high efficiency and power output.
As the transition to cleaner energy sources continues, extraction back-pressure turbines may also be called upon to integrate with carbon capture and storage (CCS) systems. These technologies are being developed to capture carbon emissions from industrial processes and power generation before they are released into the atmosphere. Advanced control systems in turbines will play a key role in integrating CCS technologies by adjusting turbine operations to optimize the capture and storage of CO2. For example, during times of low energy demand or when renewable generation is abundant, the turbine can reduce power output while focusing on steam extraction for other purposes, such as heating or industrial processes. In contrast, during peak demand, the turbine can ramp up electricity generation while ensuring that carbon capture systems continue to operate effectively. This coordination between the turbine’s operation and CCS systems will help mitigate the environmental impact of fossil-fuel-based power generation while maintaining energy supply reliability.
Another important development in the field of extraction back-pressure turbines is the growing use of digital twin technology. A digital twin is a virtual replica of a physical turbine, created using data from sensors embedded in the turbine itself. The digital twin simulates the turbine’s real-time performance, enabling operators to monitor its operation remotely and assess its condition without physically interacting with the equipment. This virtual model provides a deeper understanding of how the turbine behaves under different conditions and helps predict how changes in operating parameters or external factors will affect performance. Digital twin technology can also be used to simulate different scenarios, such as variations in steam demand or changes in grid conditions, allowing operators to fine-tune control system settings for optimal efficiency. As digital twin technology evolves, it will increasingly play a central role in the operation, maintenance, and optimization of extraction back-pressure turbines, ensuring that they continue to perform at their best throughout their operational lifespan.
Advanced control systems also provide a valuable tool for operators looking to improve the overall reliability of extraction back-pressure turbines. By continuously monitoring and adjusting turbine parameters such as temperature, pressure, and vibration, these systems can detect early signs of equipment failure and initiate corrective actions before a failure occurs. For example, if the control system detects abnormal vibrations in the turbine blades, it can trigger an automatic shutdown or adjust operating conditions to prevent damage. Predictive analytics also help operators schedule preventive maintenance, identifying when components such as bearings, seals, or valves are likely to wear out and need replacement. By minimizing the occurrence of unplanned downtime and extending the life of turbine components, these advanced control systems contribute to the long-term reliability and profitability of extraction back-pressure turbines.
In conclusion, the future of extraction back-pressure steam turbines, driven by advanced control systems, is bright and filled with opportunities for greater efficiency, flexibility, and sustainability. As industries and power systems continue to evolve, these systems will play an increasingly important role in managing fluctuating energy demands, integrating renewable sources, optimizing fuel use, and reducing emissions. The combination of AI, machine learning, predictive maintenance, digital twin technology, and carbon capture integration will continue to enhance the operational capabilities of these turbines, ensuring they remain at the forefront of energy production technology. By continually refining control strategies and embracing new innovations, extraction back-pressure turbines will remain an essential component in the pursuit of a cleaner, more reliable, and more sustainable energy future.
Extraction Back-pressure Turbines
The four main characteristics of extraction back-pressure turbines are:
- Dual-Functionality: Extraction back-pressure turbines serve a dual role, generating electricity while simultaneously extracting steam for industrial processes or district heating applications. This allows for efficient use of both the power and thermal energy produced, making them ideal for cogeneration systems.
- Pressure Regulation: These turbines operate under varying pressure conditions, with the steam extracted at different pressure levels to meet specific thermal energy requirements. The back-pressure operation ensures that the turbine can maintain the required pressure for steam extraction, which is vital for heating or industrial processes.
- Operational Flexibility: Extraction back-pressure turbines are highly flexible in terms of adapting to changes in electricity demand and thermal energy needs. They can adjust their operation to optimize the balance between power generation and steam extraction, making them suitable for fluctuating energy demands in industries or district heating networks.
- Efficiency and Cost-Effectiveness: By simultaneously producing electricity and usable steam, extraction back-pressure turbines maximize the overall energy efficiency of the system, reducing fuel consumption and operational costs. This combined heat and power (CHP) capability ensures that both energy outputs are fully utilized, making these turbines a cost-effective solution in many industrial applications.
Dual-Functionality of Extraction back-pressure Turbines
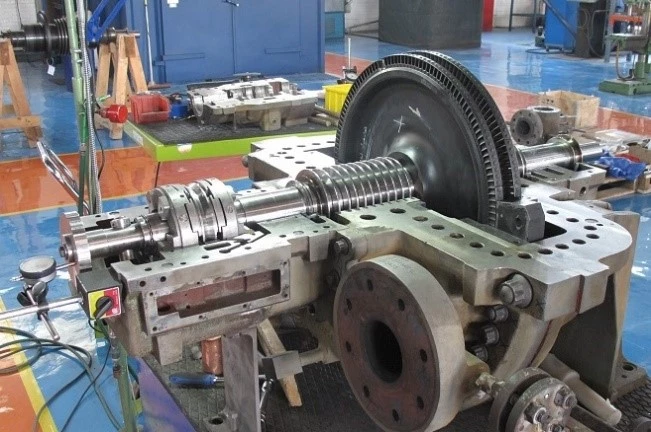
The dual-functionality of extraction back-pressure turbines is one of their most defining characteristics, combining the generation of electrical power with the extraction of steam for industrial use or district heating. This capability allows extraction back-pressure turbines to serve both as a power generation source and as a provider of thermal energy, enabling significant operational efficiencies in applications where both electricity and steam are required. This dual-purpose operation makes these turbines particularly valuable in cogeneration systems, where the goal is to maximize the use of energy resources by producing both power and heat from the same fuel source.
At the core of this dual-functionality is the turbine’s ability to extract steam at various pressure levels while simultaneously producing electrical power. As steam flows through the turbine, it expands and loses pressure, which can be tapped at different points to provide steam for heating or industrial processes. The remaining steam continues to pass through the turbine’s stages, eventually generating electricity through a generator connected to the turbine shaft. The steam extraction process allows the turbine to divert part of the steam flow, which would otherwise be used for power generation, for other applications where thermal energy is needed. These applications might include heating systems, chemical processes, or any industrial operation requiring large amounts of steam for mechanical work or heating purposes.
This versatility in both electrical and thermal energy production offers substantial benefits in terms of energy efficiency. In traditional power generation, a steam turbine typically converts the entire steam flow into electricity, with waste heat being discarded or underutilized. In contrast, extraction back-pressure turbines harness both the power and thermal potential of steam, ensuring that virtually all of the energy produced is used effectively. This means that no significant portion of the generated energy is wasted, as steam that would typically be vented or cooled off is now diverted for useful applications.
By tapping into both power and thermal energy, extraction back-pressure turbines allow for a much higher overall efficiency compared to conventional power-only turbines, particularly in industries where both electricity and heat are in demand. This is particularly true in sectors such as chemical manufacturing, paper mills, food processing, and oil refineries, where continuous or large-scale steam use is integral to the production process. Instead of relying on separate systems for power generation and steam production, a single extraction back-pressure turbine can fulfill both needs, simplifying infrastructure and improving energy consumption rates.
In cogeneration systems, this dual-functionality significantly reduces energy costs by minimizing fuel consumption. By providing both power and steam from a single source, operators can avoid the need for additional boilers or other heat generation units that would otherwise be required to meet the thermal energy demand. The ability to adjust the amount of steam extracted based on the specific needs of the industrial process also adds an element of flexibility, allowing the turbine to respond dynamically to variations in both power demand and steam requirements. This flexibility makes extraction back-pressure turbines ideal for industrial environments where energy demand is not constant, as they can ramp up or scale down steam extraction without affecting the turbine’s overall performance.
Moreover, the dual-functionality enhances the environmental sustainability of these systems. By utilizing both the thermal and electrical outputs of the turbine, energy losses are minimized, reducing the overall carbon footprint of operations. In comparison to systems that rely on separate boilers for heat and turbines for power generation, the extraction back-pressure turbine offers a more integrated and energy-efficient solution, contributing to reduced emissions and better utilization of fuel resources.
Furthermore, the adaptability of extraction back-pressure turbines makes them suitable for a range of applications beyond industrial settings. They can be employed in district heating systems, where large-scale steam is used to heat residential and commercial buildings. In these systems, the turbine extracts steam at lower pressure levels and channels it into the heating network, while simultaneously generating electricity for the grid. This dual-output capability allows utilities to optimize their energy generation, ensuring a stable supply of both power and heat while improving overall system efficiency.
The combined benefits of power and steam generation in a single turbine unit contribute to substantial economic advantages as well. Operating a single system that handles both electrical and thermal energy needs can lead to a reduction in capital expenditures for separate power and heat generation systems. The maintenance costs are also lower compared to systems that rely on multiple, independent components. Furthermore, the increased operational efficiency means that energy costs can be significantly reduced, providing a more competitive edge for industries that require consistent power and steam for their processes.
In summary, the dual-functionality of extraction back-pressure turbines makes them a highly efficient and flexible solution for cogeneration, offering both electrical power and thermal energy from the same source. This capability ensures that energy resources are maximized, reducing fuel consumption, lowering costs, and enhancing sustainability. Whether used in industrial applications, district heating systems, or other energy-intensive operations, the ability to harness both power and steam in a single turbine unit allows for optimal energy utilization, making extraction back-pressure turbines a valuable asset in modern energy systems.
The dual-functionality of extraction back-pressure turbines becomes even more valuable when considering the growing emphasis on energy efficiency and sustainability in industrial and power generation sectors. As energy prices rise and regulatory standards around emissions tighten, industries are increasingly turning to cogeneration systems to meet both their power and heat requirements in the most cost-effective and environmentally friendly manner possible. The ability of extraction back-pressure turbines to combine these two functions in one system offers a compelling solution for many businesses looking to reduce their overall energy consumption while maintaining high production standards.
This dual-purpose capability not only reduces the number of systems required to meet energy demands but also significantly cuts down on operational complexity. Traditionally, industrial facilities might have relied on separate boilers for steam generation and steam turbines for power generation, each requiring their own maintenance, control systems, and fuel inputs. Extraction back-pressure turbines eliminate the need for these additional systems, simplifying the infrastructure and lowering maintenance costs. In many cases, companies can achieve a higher return on investment (ROI) because they only need to maintain one central energy generation unit rather than multiple systems working independently.
Furthermore, the ability to extract steam at varying pressure levels provides operators with significant flexibility in how they use the turbine. This flexibility is critical for industries that experience fluctuating steam demands throughout the day or week. For example, in manufacturing environments where processes require high amounts of steam at certain times (e.g., during peak production hours) and less at others, extraction back-pressure turbines can adjust their steam extraction rates accordingly. At the same time, the turbine will continue to generate electricity, ensuring that the power output is aligned with demand, regardless of the thermal requirements. This adaptability ensures that the system operates at maximum efficiency, with no wasted energy.
Another aspect of the dual-functionality of extraction back-pressure turbines is their ability to integrate with renewable energy systems. As the world moves toward cleaner energy sources, industries and power plants are increasingly seeking ways to incorporate renewable energy into their energy mix. Extraction back-pressure turbines can complement renewable energy generation by providing a steady and reliable power supply, even when renewable sources like wind or solar are not generating enough electricity. By adjusting steam extraction based on grid demand, the turbine can operate more efficiently, filling gaps in renewable power output while reducing reliance on fossil fuels. This synergy between renewable energy and cogeneration systems will become increasingly important as countries strive to meet decarbonization goals and transition to more sustainable energy systems.
Moreover, the flexibility to adjust steam extraction without significantly affecting power output also contributes to the stability of the electricity grid. Many industrial applications using extraction back-pressure turbines operate in settings where the demand for steam is relatively constant, but power generation needs can vary throughout the day. The ability of these turbines to shift between steam extraction and electricity generation based on demand helps maintain grid stability by enabling power plants to quickly ramp up or down depending on fluctuations in power demand. This capability is especially crucial in regions with high penetration of variable renewable energy sources, where grid operators need flexible and responsive power generation units to maintain balance.
In district heating applications, extraction back-pressure turbines are also highly effective. The demand for heat is often seasonal, with peak demand during colder months and lower demand in warmer months. The ability to extract steam at varying pressures allows these turbines to adjust their operation seasonally, increasing steam extraction during the winter when demand for heating is high, and reducing it during the summer when heat demand drops. This flexibility ensures that the turbine operates efficiently year-round, adjusting to the specific needs of the district heating system without overloading the system during periods of low demand. Additionally, because the turbine is still generating electricity even while it is providing steam, it contributes to a more consistent and reliable energy supply for the local grid, further enhancing its value in energy networks.
The dual-functionality of extraction back-pressure turbines also offers potential for long-term cost savings. By consolidating the roles of power generation and thermal energy production into a single turbine, companies can reduce the need for additional infrastructure and the associated operating costs. The turbine can serve as both a power generator and a provider of heat, reducing fuel consumption and cutting down on the need for external boilers, steam systems, or heating equipment. This integration of both functions also helps reduce energy losses associated with conventional systems, where the heat from electricity generation is often wasted. With extraction back-pressure turbines, almost all the produced energy—both electrical and thermal—is put to good use, contributing to a more cost-effective and energy-efficient solution.
Additionally, the ability to modify steam extraction based on real-time requirements ensures that the turbine is always operating at an optimal efficiency level. Operators can continually adjust the turbine’s output to match changing production needs or fluctuating thermal energy demands, helping to minimize energy waste and maximize the utilization of fuel resources. This dynamic flexibility can be further enhanced by integrating advanced control systems that use real-time data to automatically adjust the turbine’s settings for maximum performance.
In conclusion, the dual-functionality of extraction back-pressure turbines is a key attribute that makes them invaluable in industries and energy systems where both electricity and thermal energy are required. By generating both power and steam simultaneously, these turbines offer unparalleled energy efficiency, flexibility, and cost-effectiveness. The ability to tailor steam extraction based on demand, while still producing electricity, ensures that these turbines can operate efficiently under a wide range of conditions, from fluctuating industrial needs to grid balancing and district heating applications. With growing energy demands and the increasing need for sustainable, low-carbon solutions, extraction back-pressure turbines are positioned to play a critical role in helping industries meet both their energy and environmental goals.
The dual-functionality of extraction back-pressure turbines extends beyond just energy efficiency and flexibility; it also offers significant environmental benefits, aligning with global efforts to reduce carbon footprints and promote sustainable energy use. In an era where industries are facing increasing pressure to reduce emissions and improve their energy efficiency, the combined power generation and steam extraction capability of these turbines represents a key advantage in the transition toward greener, more efficient energy systems.
One of the major environmental benefits of extraction back-pressure turbines is their ability to reduce fuel consumption. By integrating power and heat generation in a single system, these turbines eliminate the need for separate boilers or heating systems, which would otherwise require additional fuel inputs to generate steam for industrial processes or district heating. The efficiency gains from this integration mean that less fuel is required to meet the same energy demand, leading to reduced consumption of fossil fuels. As a result, industries that use extraction back-pressure turbines can significantly lower their carbon emissions while maintaining a reliable energy supply. This is especially important in sectors where high energy consumption is unavoidable, such as in manufacturing, chemical processing, and food production.
Additionally, because extraction back-pressure turbines are designed to operate at high efficiency, they can reduce energy waste. In traditional power generation systems, a significant amount of heat is often lost to the environment, either through exhaust gases or cooling systems. In contrast, the steam extracted from the turbine for industrial use or heating purposes is utilized, meaning less energy is wasted as unused heat. This reduction in waste heat not only contributes to overall efficiency but also helps minimize the environmental impact of the energy system, reducing the need for additional heat sources or backup power generation.
The environmental advantages of extraction back-pressure turbines become even more pronounced when they are used in cogeneration systems that incorporate renewable energy sources. In regions where renewable energy sources such as wind or solar power are being integrated into the energy mix, these turbines can complement the variability of renewable generation by providing a reliable backup power supply. During times when renewable generation is insufficient to meet demand, the turbine can adjust its operation to generate electricity while simultaneously supplying steam for heating or industrial processes. This helps ensure grid stability and reduces the need for additional fossil-fuel-based power generation. As a result, the combination of extraction back-pressure turbines and renewable energy sources can contribute to decarbonizing the energy sector, reducing reliance on fossil fuels and helping to meet global climate goals.
Moreover, in district heating applications, extraction back-pressure turbines provide a particularly efficient solution for urban areas looking to reduce emissions associated with heating. Traditional heating systems, which often rely on individual gas boilers or other fossil fuel-based systems, can contribute significantly to air pollution and carbon emissions. By using extraction back-pressure turbines for district heating, these emissions are greatly reduced, as the turbine uses the same fuel source to generate both electricity and heat. This not only reduces overall fuel consumption but also eliminates the need for additional heating infrastructure, leading to a more sustainable energy model for cities and municipalities.
The flexibility of extraction back-pressure turbines in meeting both power and thermal demands also helps improve energy resilience in local communities. For example, during times of peak energy demand or disruptions to the electricity grid, these turbines can be used to generate additional power and steam, ensuring that critical services such as hospitals, water treatment plants, or manufacturing facilities continue to receive the energy they need. In situations where renewable energy sources are not available, the ability to seamlessly switch between generating power and providing thermal energy ensures that these turbines can continue to operate efficiently, without disruption.
Furthermore, the use of advanced control systems in extraction back-pressure turbines enhances their ability to respond dynamically to changing operating conditions, contributing further to their environmental performance. These control systems can adjust the operation of the turbine in real time, optimizing steam extraction and power generation to match demand while minimizing energy waste. For example, when there is a surge in demand for heating or industrial steam, the control system can direct more steam to be extracted from the turbine, while still ensuring that electricity generation continues without efficiency losses. Similarly, if there is a drop in demand for heat, the system can adjust to prioritize power generation, ensuring that the turbine operates at peak efficiency regardless of the energy demands placed on it.
The overall result of these environmental benefits is that extraction back-pressure turbines are an essential part of the move toward cleaner and more sustainable energy systems. By reducing fuel consumption, improving energy efficiency, and minimizing emissions, these turbines offer a compelling solution for industries and utilities looking to reduce their environmental impact while meeting both power and thermal energy needs. Moreover, their ability to integrate with renewable energy sources and improve grid stability further solidifies their role in the transition to a low-carbon energy future.
In conclusion, the dual-functionality of extraction back-pressure turbines not only enhances operational efficiency and flexibility but also provides significant environmental benefits. By generating both electrical power and thermal energy from the same fuel source, these turbines reduce fuel consumption, minimize waste heat, and contribute to decarbonizing the energy sector. Their role in cogeneration systems, combined with their adaptability to renewable energy integration, makes them an important tool in achieving global sustainability goals. As industries and power systems continue to evolve, the dual-functionality of extraction back-pressure turbines will remain a key driver of energy efficiency, cost savings, and environmental stewardship.
The versatility of extraction back-pressure turbines in cogeneration systems further extends to their economic advantages, which make them a valuable asset for industries and utilities seeking to optimize their energy production and reduce operational costs. By integrating both power generation and steam extraction into a single turbine, these systems offer considerable savings in both capital expenditure and operational expenses. This economic benefit becomes especially apparent when compared to traditional systems where separate turbines and boilers would be required for electricity generation and thermal energy production.
The primary economic advantage of extraction back-pressure turbines lies in their ability to reduce the need for additional energy generation infrastructure. In conventional systems, industries must invest in separate boilers for steam generation and standalone turbines or other equipment for electricity generation. These systems require different sets of maintenance, operation, and fuel consumption, which leads to higher upfront costs and ongoing operational expenses. By consolidating both functions into a single system, extraction back-pressure turbines eliminate the need for these separate components, lowering the initial capital outlay for energy generation infrastructure.
Moreover, the reduced fuel consumption of extraction back-pressure turbines contributes to significant long-term savings. In traditional systems, energy production is often inefficient, with much of the thermal energy being wasted. By using the same steam to generate both power and heat, extraction back-pressure turbines maximize the use of fuel, ensuring that energy is utilized efficiently. This means that industries can achieve a higher energy output from the same amount of fuel, leading to lower fuel costs over time. This reduction in fuel consumption is not only beneficial from a financial standpoint but also contributes to the overall sustainability of the operation, as less fuel usage translates into fewer emissions and a smaller environmental footprint.
The flexibility inherent in extraction back-pressure turbines also provides operational cost savings. The ability to adjust steam extraction rates based on real-time demand for thermal energy means that the system can respond to changes in production schedules or seasonal energy requirements without requiring costly downtime or overcapacity. For example, during periods of low thermal demand, the turbine can prioritize power generation while minimizing steam extraction. Conversely, during periods of high steam demand, the turbine can adjust to extract more steam without affecting electricity production. This adaptability ensures that the turbine operates at peak efficiency, avoiding energy waste and reducing unnecessary fuel consumption.
In addition, the ability to operate at different pressure levels for steam extraction enables more precise control over the thermal energy output. This precision means that the turbine can be tailored to meet the specific needs of the industrial process, avoiding situations where excess heat or steam would be generated unnecessarily. This ability to fine-tune energy production helps prevent overproduction of thermal energy, which can be a significant source of waste in traditional systems. As a result, companies can ensure that only the required amount of steam is produced and used, further improving energy efficiency and reducing costs.
Another important aspect of the economic benefits of extraction back-pressure turbines is their ability to integrate with existing infrastructure. Many industries already operate with some form of steam generation system, and adding an extraction back-pressure turbine can enhance the overall energy production process without requiring a complete overhaul of existing equipment. This integration reduces installation costs and minimizes disruptions to ongoing operations. In some cases, the turbine can be incorporated into existing steam systems, allowing businesses to increase their energy production without the need for large capital investments in new infrastructure. This flexibility makes extraction back-pressure turbines a viable option for both new installations and retrofitting existing facilities.
Furthermore, the ability of these turbines to supply both electricity and steam creates opportunities for revenue generation in industries with fluctuating energy needs. For example, companies that generate excess power during periods of low internal demand can sell that electricity back to the grid, contributing to additional revenue streams. In areas where district heating is common, excess thermal energy can be directed into the heating network, providing heat to local communities or businesses, thereby generating further value. This ability to sell surplus energy or steam adds another layer of financial benefit, especially in markets where energy prices are high or there are incentives for cogeneration and renewable energy production.
The integration of advanced control systems in extraction back-pressure turbines also enhances their economic performance. These systems can monitor and optimize turbine operations in real-time, adjusting parameters to match energy demand and ensuring the system operates as efficiently as possible. By using sophisticated algorithms and sensors, these control systems can predict energy needs, prevent waste, and improve system reliability, reducing downtime and the associated costs of maintenance and repairs. Moreover, predictive maintenance enabled by these control systems can help extend the lifespan of the turbine, reducing the long-term capital costs associated with equipment replacement.
In terms of long-term financial viability, extraction back-pressure turbines also help to mitigate the impact of fluctuating fuel prices. With the global market for energy increasingly subject to volatility, businesses that rely on energy-intensive processes can benefit from the stability provided by efficient cogeneration systems. The ability to maximize fuel usage and reduce dependency on external energy sources helps companies better manage their energy costs, even in the face of rising fuel prices. Additionally, extraction back-pressure turbines support energy diversification strategies by integrating with renewable sources, enabling businesses to hedge against fuel price volatility by supplementing traditional fuel sources with cleaner, more stable energy.
In conclusion, the economic benefits of extraction back-pressure turbines are substantial, offering cost savings, improved efficiency, and revenue-generating opportunities. The integration of power and thermal energy production into a single turbine system eliminates the need for separate infrastructure, reducing capital costs and operational expenses. By maximizing fuel utilization, improving energy efficiency, and offering operational flexibility, these turbines provide long-term financial advantages for industries and utilities. As energy prices continue to fluctuate and sustainability becomes a greater focus, the ability to optimize both power and thermal energy production in a single system will remain a key factor in driving cost-effective and sustainable energy solutions across multiple sectors.
Pressure Regulation of Extraction back-pressure Turbines
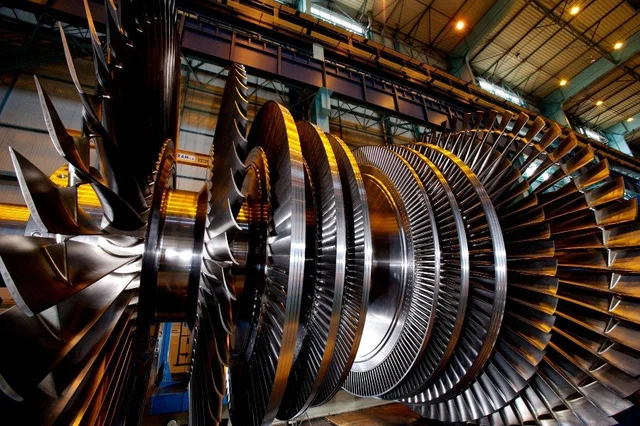
Pressure regulation in extraction back-pressure turbines is an essential aspect of their operation, ensuring the turbine can efficiently extract steam at various pressure levels while maintaining optimal performance for both electricity generation and thermal energy provision. The ability to precisely control the pressure at which steam is extracted from the turbine is crucial for balancing the demands of both power generation and industrial or district heating needs. This regulation is not only fundamental for the turbine’s operational efficiency but also contributes to the overall stability and performance of the energy system in which the turbine is integrated.
In an extraction back-pressure turbine, steam is typically extracted at intermediate stages during the expansion process. As steam passes through the turbine’s various stages, its pressure drops, and this decline can be harnessed at different extraction points to provide steam for heating or industrial processes. However, the turbine must regulate the pressure at these extraction points to ensure that the extracted steam is at the correct pressure for the intended use. For example, steam extracted for use in a district heating system may need to be at a higher pressure than steam extracted for use in a manufacturing process. Maintaining precise pressure control is critical to ensure that the steam meets the specific needs of the end-use application without wasting energy or overproducing steam.
To achieve effective pressure regulation, extraction back-pressure turbines are typically equipped with sophisticated control systems. These systems monitor the pressure and flow of steam at various stages of the turbine and adjust the operation of the turbine to match the required extraction pressures. The regulation of extraction pressure is typically accomplished through a combination of control valves, which are used to modulate the flow of steam and ensure it is extracted at the desired pressure. These valves can be adjusted dynamically based on real-time data provided by the control system, allowing for flexible operation that responds to varying energy demands.
Pressure regulation is particularly important in cogeneration systems, where both electrical and thermal energy are required. The turbine must balance the need to generate electricity with the need to supply steam at various pressures for heating or industrial purposes. If the pressure at the extraction points is not properly regulated, it could lead to inefficiencies in energy production. For instance, if the steam pressure is too high, it may not be suitable for certain industrial applications, leading to unnecessary energy consumption or the need for additional pressure-reduction equipment. On the other hand, if the pressure is too low, the steam may not meet the energy demands of the heating or industrial process, causing the system to underperform and potentially requiring additional heating sources or backup systems.
Moreover, effective pressure regulation ensures that the turbine operates at its most efficient load point. The efficiency of a steam turbine is closely tied to the pressure differential between the inlet steam and the exhaust steam. When the pressure is regulated properly, the turbine can maximize its ability to convert thermal energy into mechanical power, which is then used to generate electricity. Without accurate pressure regulation, the turbine may experience pressure imbalances that reduce its efficiency, leading to higher fuel consumption and greater wear and tear on the turbine components. Proper regulation helps to ensure that the steam flow is optimized, reducing the risk of inefficiency and the need for excessive maintenance.
In addition to regulating extraction pressures for various applications, the turbine’s exhaust pressure is also a key factor in overall system performance. In extraction back-pressure turbines, the exhaust pressure is determined by the load conditions of the system. The exhaust pressure must be maintained at a level that allows the turbine to continue producing electricity while ensuring that steam is effectively extracted for the necessary purposes. This is typically achieved through a combination of exhaust pressure control valves and back-pressure settings, which help balance the pressure in the turbine’s exhaust stages. Maintaining the correct exhaust pressure is particularly important in systems where the turbine is part of a larger network, such as a district heating system or an industrial facility that requires continuous steam supply. If the exhaust pressure is too high, it can result in a decrease in the turbine’s ability to generate power, whereas if it is too low, it can cause excessive steam extraction, leading to steam shortages for heating or industrial processes.
The ability to adjust steam extraction points and pressure settings in real-time also contributes to the turbine’s operational flexibility. Industries often face fluctuating demands for both electricity and thermal energy throughout the day, with periods of high demand for heating and others requiring more electricity. By having precise control over extraction pressures, extraction back-pressure turbines can dynamically adjust to these changing conditions. For example, during times of high thermal demand, the turbine can be set to extract steam at higher pressures, increasing the availability of steam for heating systems. Conversely, during periods of lower thermal demand, the turbine can reduce the extraction pressure and shift its focus toward electricity generation, optimizing the overall efficiency of the system.
In addition to the operational flexibility, pressure regulation in extraction back-pressure turbines also contributes to the longevity of the equipment. Turbines that operate with poorly regulated pressure levels may experience excessive wear on their components due to pressure fluctuations, which can lead to higher maintenance costs and potential equipment failure. By maintaining consistent and accurate pressure levels throughout the turbine’s operation, the wear and tear on components such as steam nozzles, blades, and seals are minimized, extending the turbine’s operational life. This reduced wear also translates into lower maintenance costs, fewer unplanned downtime events, and a more reliable system overall.
Furthermore, advanced pressure regulation can help extraction back-pressure turbines integrate with other systems within a larger energy network. In district heating networks, for example, the pressure regulation ensures that the turbine can supply steam at the appropriate pressure levels to heat residential and commercial buildings while simultaneously generating electricity for the grid. The turbine’s ability to adjust steam extraction based on the specific needs of the heating system or power grid is a crucial feature that allows the turbine to operate as part of a broader energy management system. This integration with other energy networks is particularly valuable in systems that rely on variable renewable energy sources, such as wind or solar, as it enables the turbine to provide a steady source of power and heat even when renewable energy production is intermittent or fluctuating.
Overall, pressure regulation is a fundamental aspect of the operation of extraction back-pressure turbines, ensuring that steam is extracted at the right pressure for both electrical generation and thermal applications. This regulation maximizes efficiency, minimizes energy waste, and extends the life of the turbine by reducing mechanical stress. By integrating advanced control systems, pressure regulation offers operational flexibility, allowing the turbine to adjust to changing energy demands in real-time while maintaining optimal performance. In systems where both electricity and steam are required, effective pressure regulation is crucial for maintaining balance, minimizing costs, and ensuring the turbine operates at peak efficiency.
Effective pressure regulation in extraction back-pressure turbines not only optimizes energy production but also plays a crucial role in enhancing system stability and ensuring safety during operation. The ability to control pressure at various stages of steam extraction is fundamental in preventing potential issues such as pressure surges, which could lead to equipment damage, or steam starvation, which could disrupt the overall operation. To maintain safe and reliable operation, advanced pressure regulation mechanisms work in conjunction with other safety systems to ensure that the turbine operates within its designed pressure parameters.
In scenarios where unexpected fluctuations in steam demand or power generation occur, precise pressure regulation helps to avoid dangerous pressure build-ups or drops. If steam extraction demands change suddenly, such as during load shifts or unexpected changes in external heating or cooling needs, the turbine must quickly adapt. If the system does not respond promptly and efficiently, it could result in a sharp drop in steam quality or cause excessive expansion of the steam, leading to mechanical stress on components and ultimately premature failure. The pressure control system’s ability to respond dynamically to these changes ensures that the turbine remains within its operational limits and that pressure imbalances do not negatively affect the overall system’s performance.
Furthermore, pressure regulation plays a role in optimizing fuel consumption, contributing directly to cost reductions. Maintaining a stable pressure throughout the turbine ensures that steam is extracted at the most efficient rate, preventing unnecessary energy waste that could arise from over-extraction or under-extraction of steam. By keeping pressure levels at the required set points, the turbine minimizes the amount of fuel needed to meet the electrical and thermal energy demands of the system. This optimization not only lowers operational costs but also helps to extend the lifespan of fuel resources, a critical consideration in industries with high energy demands.
Another key benefit of pressure regulation is its contribution to balancing multiple energy outputs, such as electricity and heat, with minimal waste. In industrial settings or district heating systems, there may be simultaneous demand for both thermal energy and electrical power. The pressure regulation system ensures that the turbine can simultaneously supply steam at the correct pressure for heating purposes while generating electricity efficiently. This dynamic balance is essential for ensuring that both energy demands are met without compromising the efficiency or reliability of the turbine.
This feature also allows the turbine to respond flexibly to fluctuations in external demand. For example, if electricity demand increases and the turbine is required to produce more electricity, the pressure system can adjust extraction points and modulate the steam flow accordingly. Conversely, if the thermal demand for steam increases (such as in colder months when heating requirements rise), the system can prioritize the extraction of steam at the required pressure without hindering the turbine’s electricity production. The adaptability of the pressure regulation system enables extraction back-pressure turbines to maintain high operational efficiency while simultaneously ensuring optimal energy supply to meet variable demands.
The precise control over steam extraction pressure also helps in improving operational safety by reducing the risks of overpressure situations. Overpressure conditions can lead to dangerous consequences, including damage to the turbine, piping, and connected systems. Effective pressure regulation mechanisms, including pressure relief valves and automated control systems, ensure that pressure is continuously monitored and maintained within safe operating limits. If pressure readings exceed predefined safety thresholds, the system can trigger automatic shutdown procedures or engage safety relief systems to prevent potential damage. This protective aspect is crucial for ensuring the long-term reliability of the turbine and the broader energy system.
Advanced sensors and automated control systems enable more detailed monitoring of steam pressure at each extraction point. These sensors provide real-time feedback, allowing for fine-tuned adjustments to be made based on immediate operational requirements. This allows for greater precision and minimizes human error, ensuring that pressure regulation is maintained without the need for manual intervention. With such systems, operators are provided with continuous data that informs decision-making and helps optimize the turbine’s performance, leading to smoother operation and improved overall efficiency.
Moreover, the pressure regulation system contributes to the seamless integration of extraction back-pressure turbines with other components in the energy network. In combined heat and power (CHP) systems or district heating networks, extraction back-pressure turbines must work in harmony with other heat generation and distribution systems. By maintaining the correct extraction pressure, these turbines can ensure that steam is delivered to the grid or local heating network at a consistent and usable pressure, improving the reliability and stability of the overall system. In turn, this ensures that both power plants and heating systems can operate together efficiently, supporting the overall energy infrastructure.
To sum up, pressure regulation is integral to the effective and safe operation of extraction back-pressure turbines. It enables the turbine to meet the varying energy demands of both power generation and thermal applications, ensuring that steam is extracted at the required pressure for maximum efficiency. Advanced pressure control systems help avoid mechanical stress, optimize fuel consumption, and maintain safe operating conditions, while also contributing to system flexibility and adaptability. By allowing for dynamic adjustments to steam extraction based on real-time demands, pressure regulation ensures that the turbine can provide reliable energy for both electricity and heat, supporting industries and communities with a stable and efficient power supply.
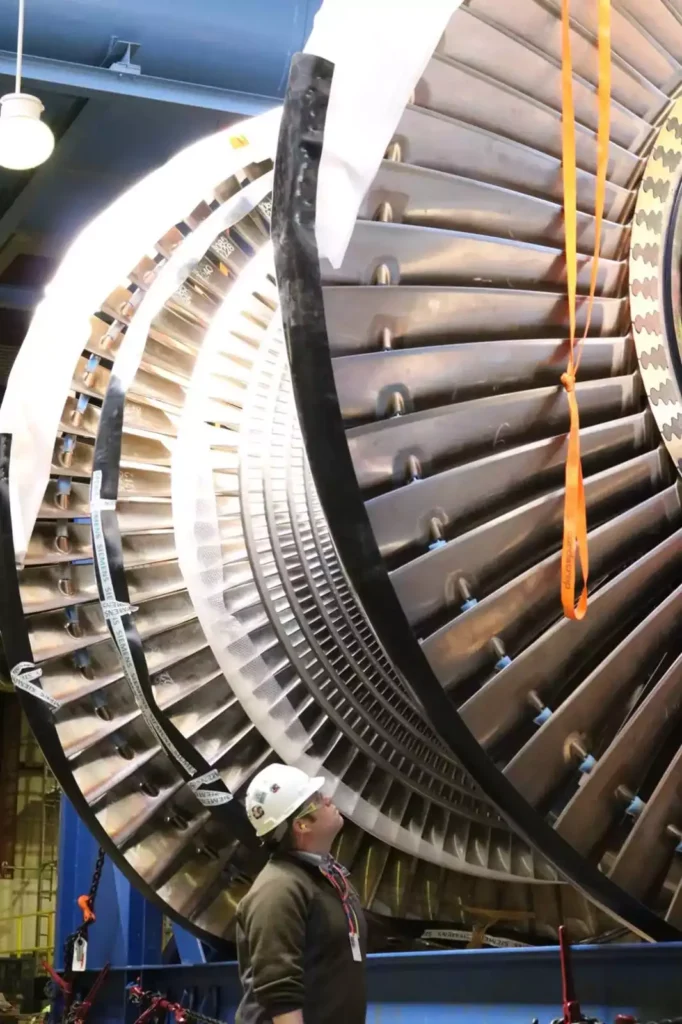
Pressure regulation in extraction back-pressure turbines also has a significant impact on operational maintenance and long-term reliability. The precision with which pressure levels are controlled directly influences the wear and tear on critical turbine components, such as blades, seals, and valves. By maintaining steady and appropriate pressures during turbine operation, the system minimizes the risk of erosion, vibration, and other mechanical stresses that can lead to premature component failure. This helps reduce the frequency and cost of maintenance activities, leading to longer intervals between scheduled downtimes and a reduction in unplanned maintenance events.
Moreover, the wear on components like steam nozzles and blades is minimized when steam is extracted at consistent pressure levels. Excessive fluctuations in steam pressure can cause uneven flow distribution within the turbine, which can result in localized hot spots, irregular steam velocities, or unwanted stress on specific areas of the turbine. Such issues can cause material fatigue and accelerate the deterioration of turbine parts, leading to costly repairs or replacements. The pressure regulation system’s ability to keep extraction pressure stable is key to ensuring that the turbine’s components experience uniform operating conditions, thereby extending their lifespan and ensuring that the turbine remains reliable over the long term.
Another significant aspect of pressure regulation is its role in reducing the risk of energy waste. When steam is extracted at inappropriate pressures, it can lead to both underutilization of energy and excessive energy consumption. For instance, if steam is extracted at too high a pressure when a lower pressure would suffice, it means that more steam is generated than is needed, requiring the system to handle surplus steam and potentially leading to increased fuel consumption to maintain high levels of steam production. Conversely, if the pressure is too low, the turbine may fail to meet the necessary thermal requirements, requiring the use of additional fuel-burning equipment to make up for the shortfall.
By continuously regulating steam extraction pressures, the turbine is able to provide the exact amount of steam needed for the process or heating requirements, without generating excess or inadequate amounts. This efficient use of energy directly translates into fuel savings, reducing operational costs and minimizing environmental impact. It also ensures that the turbine operates within the optimal load range, avoiding the inefficiencies associated with running at off-design conditions.
Pressure regulation systems also support more advanced operating modes, such as partial load operation, which is common in industries that require flexible energy generation. For instance, in cogeneration systems where both electrical and thermal energy are produced, the turbine must be able to adjust its steam extraction based on the changing needs of the process. During times of high electricity demand, the turbine may prioritize power production, reducing steam extraction to a minimum. Conversely, during times of peak thermal demand, such as during the winter months when heating requirements increase, the turbine can be configured to extract more steam at higher pressures, reducing power generation output.
This flexibility allows extraction back-pressure turbines to operate efficiently across a wide range of conditions without sacrificing performance in either electricity generation or thermal energy supply. The pressure regulation system helps achieve this by continuously monitoring and adjusting steam extraction pressures in real-time, providing a high degree of operational flexibility. This is particularly valuable in industries or systems where energy demand is highly variable, such as in large manufacturing plants, district heating networks, or power plants with fluctuating grid demand.
Furthermore, pressure regulation can help extraction back-pressure turbines achieve better responsiveness in systems where energy demand changes rapidly. For instance, if a sudden increase in heating demand occurs, the turbine can quickly adjust to provide the necessary steam at the appropriate pressure without waiting for a manual intervention. Likewise, if the electricity grid requires more power, the turbine can prioritize electricity generation by adjusting the extraction pressure accordingly. The ability to make these adjustments quickly ensures that the turbine can meet changing demand while maintaining optimal efficiency, all without incurring the cost and downtime associated with reconfiguration or significant manual intervention.
In terms of integration with external systems, pressure regulation is essential for ensuring that the turbine is compatible with other energy sources. For example, in a hybrid system that combines renewable energy generation (such as solar or wind) with conventional power generation, pressure regulation ensures that steam extraction is consistent, even if renewable energy generation fluctuates. This helps maintain the overall stability of the system, as steam extraction pressure can be adjusted to complement changes in renewable energy output, ensuring a steady and reliable supply of thermal and electrical energy.
The ability of the turbine’s pressure regulation system to integrate with grid management systems is also critical. Many modern energy grids are designed to incorporate a mix of renewable and non-renewable energy sources, which can be intermittent and subject to variability. In these cases, pressure regulation can help maintain system balance by ensuring that steam extraction is adjusted in response to fluctuations in energy demand or supply. This can be particularly important in regions with high renewable energy penetration, where the turbine’s ability to shift its focus between thermal and electrical energy can help stabilize the grid and reduce the need for backup fossil fuel generation.
Lastly, pressure regulation systems in extraction back-pressure turbines are often linked to broader digital control systems that leverage data analytics, artificial intelligence, and machine learning algorithms to optimize turbine performance. By analyzing historical data on steam extraction pressures, turbine efficiency, and fuel consumption, these advanced systems can predict future operational needs, enabling the turbine to adjust in advance of any changes in demand. This predictive capability helps prevent inefficiencies before they occur and ensures that the turbine is operating at its most efficient point at all times, further enhancing fuel savings and system reliability.
In conclusion, pressure regulation in extraction back-pressure turbines is a critical component that impacts every aspect of turbine performance, from operational efficiency to long-term reliability and system stability. The ability to maintain consistent and precise steam extraction pressures helps minimize wear on turbine components, optimize fuel consumption, and prevent energy waste. It also enables greater operational flexibility, allowing the turbine to respond quickly and efficiently to changes in energy demand, ensuring that both electrical and thermal energy needs are met without compromising performance. Advanced pressure regulation systems not only improve operational efficiency but also support integration with other energy sources and systems, providing a stable and adaptable solution for modern, dynamic energy markets. Through continuous monitoring, real-time adjustments, and predictive analytics, pressure regulation helps extraction back-pressure turbines achieve peak performance and extend their operational life, making them a key asset for industries and utilities seeking reliable and efficient energy solutions.
In addition to the operational and efficiency benefits, pressure regulation in extraction back-pressure turbines significantly contributes to enhancing the overall sustainability of energy systems. As the global focus on reducing carbon emissions and improving energy efficiency intensifies, the role of pressure regulation becomes increasingly important in helping turbines meet these environmental objectives. By optimizing steam extraction pressures, turbines can work at their highest efficiency, reducing the overall fuel consumption and, consequently, minimizing carbon emissions associated with energy generation. This is particularly crucial in industries or regions where energy demand is high and the reliance on fossil fuels is substantial.
When pressure regulation is effectively implemented, it enables the turbine to extract steam at the lowest possible pressure while still meeting the required thermal or process energy needs. This minimizes the need for supplementary energy sources, such as boilers or additional fuel-burning equipment, which might otherwise be required to compensate for inefficiencies in steam extraction or pressure imbalances. By ensuring that the turbine operates within an optimal range, fuel consumption is reduced, which directly translates into fewer emissions per unit of energy produced. This not only supports environmental goals but also aligns with regulatory requirements and energy sustainability targets set by governments and organizations.
Additionally, pressure regulation enhances the integration of renewable energy sources in hybrid or combined systems, further supporting sustainability goals. For example, in systems that incorporate both fossil fuel and renewable energy generation, pressure regulation allows the turbine to adjust to fluctuating renewable energy output, ensuring that steam extraction and energy production are balanced even when renewable energy sources, such as wind or solar power, experience variability. This flexibility helps reduce reliance on backup fossil fuel generation, thus decreasing the carbon footprint of the energy system. As renewable energy sources become more prevalent, the ability of extraction back-pressure turbines to adapt to these changes in real-time will be crucial for maintaining grid stability while minimizing environmental impact.
Another way that pressure regulation supports sustainability is by reducing the frequency and intensity of maintenance and repairs. Turbines that operate with fluctuating pressures are at greater risk of experiencing mechanical wear, component failure, or even safety incidents due to overpressure or underpressure situations. In contrast, precise pressure regulation ensures that the turbine remains within its designed operating parameters, reducing the mechanical stress on its components. This not only improves the overall reliability and lifespan of the turbine but also lowers the need for spare parts, replacements, and extensive maintenance. Fewer repairs and downtime mean that the turbine can continue to operate efficiently over a longer period, reducing the energy consumption and resources required for its upkeep.
Moreover, advanced pressure regulation systems can be integrated with smart grid technologies and advanced monitoring systems, further enhancing the sustainability and flexibility of the overall energy system. These systems can help operators predict energy demand trends, adjust extraction pressures in advance, and better manage energy production and consumption across the grid. By automating pressure regulation and integrating it with grid management platforms, the turbine can operate with greater foresight and precision, ensuring that energy production is always aligned with demand while minimizing fuel consumption and emissions. The implementation of such smart systems also allows for real-time optimization of turbine operation, improving both short-term efficiency and long-term sustainability.
Pressure regulation also plays a critical role in improving system-wide resilience. As energy systems become more interconnected and complex, the ability of turbines to adapt to a wide range of operational conditions becomes crucial for ensuring grid stability and reliability. In regions prone to extreme weather events or fluctuations in energy demand, the pressure regulation system in an extraction back-pressure turbine can help ensure a consistent steam supply for heating or industrial processes, even when renewable energy generation or grid conditions fluctuate. This resilience is particularly important in areas where there may be limited access to backup power generation sources. By maintaining pressure stability during such fluctuations, the turbine helps stabilize the overall energy system and ensures that end-users continue to receive a steady supply of power and thermal energy.
Lastly, pressure regulation contributes to the cost-effectiveness of the system, which, in turn, can support the economic sustainability of the energy operation. While the initial installation of pressure control systems may incur a capital cost, the long-term benefits of reducing fuel consumption, improving efficiency, minimizing maintenance costs, and extending the lifespan of the turbine far outweigh these initial expenses. Moreover, in a competitive energy market, the ability to optimize performance and reduce operational costs provides a significant advantage, as the turbine can generate more energy for less cost, improving profitability and helping operators stay competitive. Additionally, when the turbine operates more efficiently, it can meet energy demands more effectively, reducing the need for supplemental, often more expensive, energy sources.
The integration of pressure regulation with energy storage systems also enhances the overall sustainability of the turbine. In grid-connected systems where energy storage technologies are deployed to store excess renewable energy, pressure regulation can help manage the steam extraction process to match the availability of stored energy. This can be especially beneficial during periods of low demand, where excess energy can be used to produce steam for heating or other industrial processes. By adjusting the steam extraction pressure based on the availability of stored energy, the system can further reduce fuel consumption, making the turbine even more sustainable.
In summary, pressure regulation in extraction back-pressure turbines not only ensures the efficient, reliable, and safe operation of the turbine itself but also contributes to the sustainability of the broader energy system. By optimizing fuel use, minimizing emissions, and extending the lifespan of turbine components, pressure regulation helps meet both environmental and economic sustainability goals. The integration of advanced monitoring and control systems further enhances the turbine’s ability to adapt to changing energy demands, improving system flexibility and reducing the reliance on less sustainable energy sources. The ability to manage steam extraction pressures dynamically also ensures that turbines can be seamlessly incorporated into hybrid or renewable-heavy energy systems, improving grid stability and supporting the transition to a more sustainable, low-carbon energy future.
Operational Flexibility of Extraction back-pressure Turbines
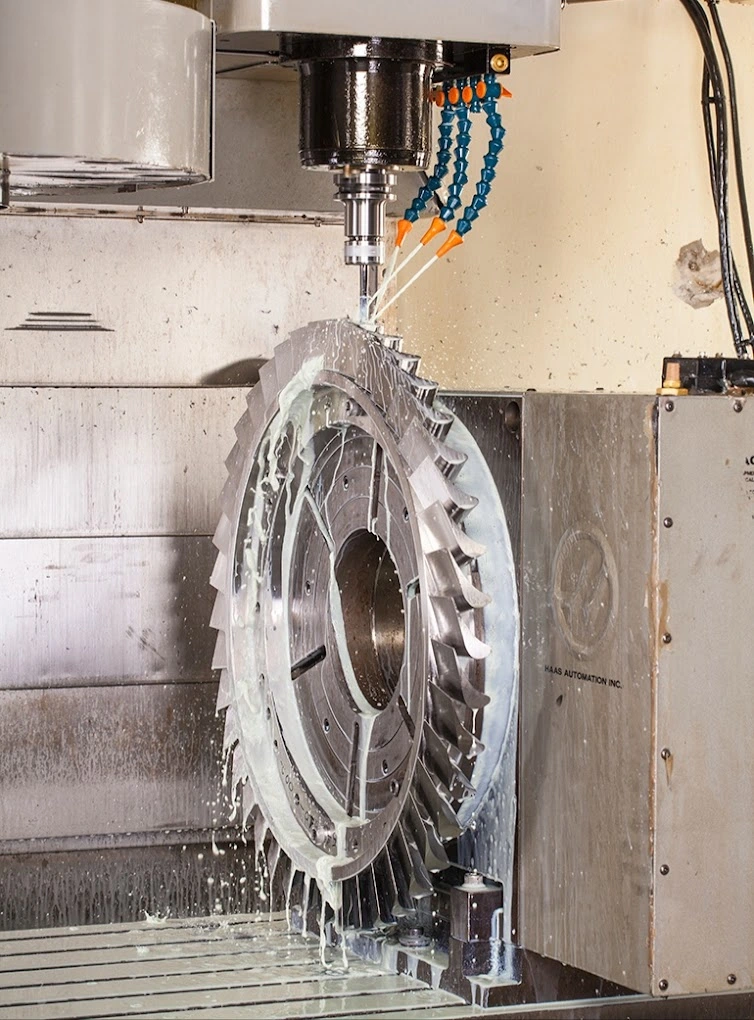
The operational flexibility of extraction back-pressure turbines is one of their most defining characteristics, allowing them to adapt to a wide range of energy demands and operational conditions. This flexibility is critical in ensuring that these turbines can function effectively across different sectors, particularly in industries and systems where both electrical power generation and thermal energy are required simultaneously. The ability to adjust steam extraction rates and pressure according to changing conditions enhances the turbine’s overall efficiency, allowing it to respond to fluctuating energy needs without compromising on performance or safety.
Extraction back-pressure turbines are designed to accommodate variations in both the quantity and the quality of steam extracted. Unlike conventional steam turbines, which are typically designed for a fixed load, extraction back-pressure turbines can dynamically adjust to differing levels of steam demand. This makes them particularly useful in cogeneration systems, where steam is extracted at intermediate pressures for heating applications while the remaining steam is used for power generation. In these systems, the extraction back-pressure turbine must be capable of adjusting its steam extraction points in response to variations in both thermal and electrical energy needs. Whether demand for heat increases during colder months or electricity needs spike due to changing grid conditions, the turbine can quickly modulate its performance to ensure that the necessary steam pressure is maintained for both applications.
One of the key aspects of this flexibility is the turbine’s ability to modify its extraction pressure across multiple stages. Extraction points in the turbine can be configured to provide steam at different pressures, allowing the turbine to serve both high- and low-pressure steam applications simultaneously. This multi-stage extraction process is particularly valuable in industries such as district heating, chemical processing, or food manufacturing, where varying levels of steam pressure are required for different processes. The turbine adjusts the pressure at each extraction point depending on the operational requirements, enabling the system to optimize steam distribution based on real-time needs. The result is a system that can provide an uninterrupted and reliable supply of both thermal and electrical energy, even as operational conditions fluctuate.
Operational flexibility also comes into play when external factors such as fuel availability, energy prices, or grid conditions change. For instance, when electricity prices rise or grid demand peaks, the turbine can shift its operation to prioritize electricity generation by adjusting its steam extraction profile. In contrast, when the need for thermal energy increases, such as during periods of high heating demand, the turbine can redirect more steam to the heating system without significantly affecting electricity generation. This level of operational adaptability ensures that extraction back-pressure turbines can maintain efficiency and reliability under varying conditions, helping to optimize energy use and reduce costs.
Another factor contributing to the operational flexibility of extraction back-pressure turbines is their ability to handle variable load conditions. Many industrial processes and utilities face fluctuating energy demand throughout the day, with periods of high and low load. The turbine’s ability to adjust steam extraction pressures and power output in response to these variations allows it to continue operating at optimal efficiency, even under less-than-ideal load conditions. During periods of low electricity demand, for example, the turbine can reduce its output without causing a loss of efficiency, ensuring that steam is still supplied for heating applications. Conversely, when demand rises, the turbine can ramp up power production to meet the increased need for electricity, seamlessly adjusting to the new load conditions.
The system’s flexibility is further enhanced by the integration of advanced control technologies. These systems enable real-time monitoring of both electrical and thermal energy demands, allowing operators to adjust turbine settings quickly and automatically. Modern extraction back-pressure turbines are often equipped with digital control systems that allow for automated optimization of steam extraction pressure, based on both immediate operational requirements and long-term performance goals. These systems can continuously adjust the turbine’s performance, ensuring that the turbine operates efficiently across a range of scenarios, from fluctuating load conditions to sudden changes in process requirements. Moreover, these control systems can also communicate with broader energy management systems, enabling more sophisticated coordination of power and thermal generation across a network of turbines and energy assets.
One of the most significant benefits of this operational flexibility is the potential for energy savings and cost reductions. By allowing the turbine to adapt to fluctuating demand patterns, it can avoid running at inefficient or off-design conditions. In traditional systems, if the energy demand for either heat or electricity suddenly drops, the turbine would be forced to continue running at full capacity, wasting fuel and increasing operational costs. In contrast, extraction back-pressure turbines can adjust their operation to match real-time energy needs, optimizing fuel consumption and reducing waste. This dynamic optimization of both electrical and thermal energy production allows operators to achieve significant savings over time, both in terms of energy costs and fuel consumption.
The ability to scale production up or down in response to changing demand is especially beneficial in systems with mixed energy sources. In combined heat and power (CHP) systems, for example, extraction back-pressure turbines can work in tandem with other generators, such as natural gas turbines or renewable energy sources like solar or wind power. When renewable energy sources are abundant, the turbine can reduce its load, allowing excess energy from renewables to be used for other purposes. Conversely, when renewable energy production decreases or electricity demand increases, the turbine can quickly ramp up its output to compensate, ensuring a reliable energy supply while minimizing reliance on fossil fuels.
This adaptability also extends to situations where steam production might need to be adjusted due to external environmental or operational factors. In applications where there are varying heating demands based on external temperatures, for example, extraction back-pressure turbines can modulate steam output accordingly. If external temperatures drop and the demand for heating increases, the turbine can adjust its extraction pressures to provide more steam for heating, ensuring that thermal energy is available even during peak usage periods. Similarly, if the demand for heating decreases, the turbine can scale back steam extraction without impacting electricity production, maintaining optimal energy efficiency.
Furthermore, the operational flexibility of extraction back-pressure turbines also enables them to operate effectively in diverse geographical regions. Whether in remote areas where infrastructure may be limited or in industrial zones where high levels of energy demand are constantly changing, these turbines can adjust to local conditions, providing a reliable and efficient energy supply. In some cases, turbines may need to operate in environments with varying fuel qualities or variable heat sources, and the flexibility to adapt to these conditions is vital for maintaining optimal performance.
Overall, the operational flexibility of extraction back-pressure turbines ensures that they can meet the varying demands of modern, dynamic energy systems. Their ability to adjust steam extraction pressures, prioritize either electrical or thermal energy generation, and respond to external fluctuations in fuel availability or demand allows them to provide reliable and efficient power and heat. By incorporating advanced control systems and automation technologies, these turbines offer significant potential for cost savings, fuel optimization, and enhanced operational efficiency. Whether in industrial, utility, or district heating applications, extraction back-pressure turbines play a vital role in maintaining system stability and meeting the complex energy needs of today’s world.
Continuing from where we left off, the operational flexibility of extraction back-pressure turbines also allows for more sophisticated load management strategies, particularly in systems that are part of a larger, integrated energy network. In such systems, extraction turbines can be managed in conjunction with other power generation units, thermal storage, or backup generators, forming part of a holistic approach to energy optimization. When connected to district heating networks, for instance, the turbine’s ability to adjust steam extraction based on fluctuating heating demands ensures that thermal energy is available during peak demand periods, without overproducing and wasting fuel during off-peak hours. This flexibility becomes even more crucial in areas that rely on seasonal heating or cooling, where the energy requirements can vary significantly.
In addition to heating, extraction back-pressure turbines can contribute to peak shaving and grid stabilization. In regions with high demand fluctuations or where the grid is under stress, these turbines can adjust their operation to provide additional power when needed. This is particularly valuable during periods of peak electricity consumption, such as during summer afternoons when air conditioning use surges or in winter when heating needs are high. Instead of requiring operators to rely on less-efficient peaking power plants, which are typically more expensive and environmentally taxing, the extraction turbine can quickly ramp up its electrical generation capacity by adjusting the steam flow and extraction pressures. This helps stabilize the grid, ensuring that there is enough capacity to meet demand without relying on carbon-intensive energy sources.
Furthermore, the increased flexibility in managing power and heat generation can also enhance the integration of renewable energy sources into the energy mix. For example, wind or solar power is intermittent by nature, meaning that electricity generation from these sources can fluctuate throughout the day. In such cases, extraction back-pressure turbines can be adapted to respond to these changes, increasing or decreasing their output as needed. During periods of high renewable generation, the turbine may reduce its power output, prioritizing thermal energy generation. Alternatively, when renewable generation is low, the turbine can ramp up electrical production to ensure that there is no disruption in energy supply. This ability to balance both thermal and electrical generation not only supports a more stable grid but also reduces the reliance on fossil fuels, enhancing the sustainability of the overall energy system.
In terms of fuel flexibility, extraction back-pressure turbines are capable of adjusting to variations in fuel quality or supply. For example, in industrial processes where the fuel used to produce steam may vary, the turbine can maintain its performance by adjusting operational parameters based on the quality or type of steam entering the system. If the fuel used changes—for example, from natural gas to biomass or from one type of coal to another—the turbine’s control system can recalibrate the system to optimize combustion efficiency, steam generation, and extraction pressures. This adaptability helps mitigate the risks associated with fuel price volatility or supply disruptions, allowing facilities to continue operating efficiently and without costly interruptions.
Moreover, in many industries, extraction turbines can be employed in systems where co-generation or tri-generation (combined cooling, heating, and power) takes place. The flexibility of the turbine allows it to shift between different modes of operation depending on which aspect of energy generation is most in demand. For instance, during colder months, the turbine may shift focus to prioritizing steam extraction for heating purposes, while during warmer months, the need for cooling or electricity may increase, leading the turbine to adapt and produce more electrical power. This shift in operational modes can be performed seamlessly, ensuring that the system continues to operate efficiently, without the need for frequent reconfiguration or downtime.
The enhanced flexibility also means that extraction back-pressure turbines can be utilized effectively in applications where load is highly variable. For instance, in processes with intermittent steam requirements—such as batch processes or those that operate on shift-based schedules—the turbine can adapt its steam extraction to match the process demand. This results in improved efficiency and cost savings, as the turbine will not be forced to operate at full capacity during periods of low demand. Instead, it can scale its output in accordance with real-time needs, avoiding energy waste while maintaining system reliability.
Operational flexibility in extraction back-pressure turbines also extends to their ability to integrate into modern digital ecosystems. Today’s turbine systems are increasingly equipped with sensors, data analytics, and predictive maintenance technologies that enhance their adaptability. Through continuous monitoring of parameters such as steam pressure, temperature, flow rates, and turbine health, these systems can make real-time adjustments to optimize performance. If a change in load or operating conditions is anticipated, the system can proactively adjust turbine settings to prepare for the shift, maintaining optimal performance and preventing inefficiencies before they occur. Predictive analytics can also forecast maintenance needs, ensuring that the turbine is properly serviced before issues arise, preventing downtime and further enhancing operational flexibility.
As part of this digital transformation, extraction back-pressure turbines can be remotely monitored and controlled, which provides an added layer of flexibility, especially for facilities that are spread across multiple locations or are operating in challenging environments. Remote control allows for quick adjustments to turbine settings in response to external factors such as weather conditions, grid instability, or process changes, all without the need for on-site intervention. This ease of control can significantly improve operational efficiency, as facility managers can make adjustments based on up-to-the-minute data from anywhere, at any time.
In industries where the demand for energy is highly time-sensitive, such as in hospitals, chemical plants, or data centers, the ability of extraction back-pressure turbines to quickly adapt to changes in energy needs becomes even more crucial. In these settings, disruptions in either thermal or electrical energy supply can lead to significant operational challenges or even safety hazards. With operational flexibility, extraction turbines ensure that energy demand is met without interruption, allowing processes to continue smoothly, even when conditions fluctuate unexpectedly.
In summary, the operational flexibility of extraction back-pressure turbines enables them to adjust seamlessly to changing operational conditions, ensuring that both electrical power and thermal energy needs are met without compromise. Whether through real-time steam pressure adjustments, integration with renewable energy sources, or the ability to adapt to varying fuel qualities, these turbines offer a level of versatility that makes them ideal for a wide range of applications. Their adaptability to fluctuating energy demands, load conditions, and operational schedules enhances their efficiency, reduces costs, and supports the sustainability of energy systems. As energy management becomes increasingly complex and dynamic, the role of flexible turbines in maintaining grid stability, optimizing energy use, and ensuring system resilience becomes ever more critical.
Continuing further, the operational flexibility of extraction back-pressure turbines offers significant advantages in the context of future energy systems. As the global energy landscape shifts toward cleaner, more sustainable energy sources, extraction turbines are uniquely positioned to play a key role in facilitating this transition. With renewable energy technologies like wind and solar becoming more integrated into national and regional energy grids, the variability and intermittency of these sources necessitate energy systems that can quickly respond to fluctuations in power generation and demand. Extraction back-pressure turbines, with their ability to adjust to both varying steam extraction pressures and fluctuating power needs, offer a crucial mechanism to stabilize the grid and balance out these renewable energy fluctuations.
One of the ways these turbines contribute to grid stability is by serving as a flexible source of backup power. During periods of high renewable generation, such as on windy or sunny days, the turbine can scale down its electrical output, effectively allowing the system to store excess energy or export it elsewhere without overloading the grid. Conversely, when renewable power generation falls, such as when the wind slows down or cloud cover reduces solar output, the turbine can ramp up its output to compensate for the deficit, ensuring that there is no gap in energy supply. This capability is vital in areas with high penetration of renewables, as it allows for the smooth integration of intermittent energy sources without compromising grid reliability.
In addition, extraction back-pressure turbines provide operational flexibility through their role in thermal storage integration. In many modern systems, especially those incorporating combined heat and power (CHP) configurations, thermal storage is used to store excess heat generated during periods of low demand and release it when demand spikes. This is particularly useful in industries that require continuous heat but have fluctuating needs for steam, such as in chemical processing or district heating applications. The turbine’s ability to adjust its steam extraction dynamically ensures that stored thermal energy can be deployed effectively, allowing for consistent heating output when needed without disrupting electricity generation. By enabling the integration of thermal storage, these turbines further enhance the overall flexibility of the system and support the efficient use of both thermal and electrical energy.
Moreover, the ability of extraction back-pressure turbines to function in combined systems with other types of generation units, such as natural gas turbines, biomass boilers, or even hydrogen-based power plants, allows for diverse operational modes. This dual- or multi-fuel operation enables operators to select the most cost-effective or sustainable energy sources at any given time. When natural gas prices are low or availability is high, for instance, the turbine can operate in concert with gas turbines to produce electricity. When renewable or biomass generation becomes more favorable, the turbine can adjust its operation to prioritize thermal energy production. This flexibility not only helps optimize the use of available fuel resources but also provides a way to reduce overall carbon emissions by leveraging cleaner fuels when possible.
Additionally, the turbine’s ability to handle varying load conditions is increasingly valuable in the context of microgrids and decentralized energy systems. As more regions move towards distributed generation models, extraction back-pressure turbines can serve as a key component in maintaining a stable, reliable energy supply in smaller, localized grids. These smaller grids, often powered by a mix of local renewable sources and traditional generation, require components that can adjust to rapidly changing conditions. Extraction turbines, through their flexible operation, can meet local energy needs with minimal disruption, supporting both electrical and thermal load requirements. This is particularly relevant in remote or off-grid locations where grid connectivity may be limited or where energy systems need to be self-sufficient, such as in remote mining operations or large industrial plants.
The integration of advanced sensors and real-time data analytics enhances the operational flexibility of extraction back-pressure turbines. With the advent of the Internet of Things (IoT) and machine learning, turbine systems are becoming more intelligent, capable of continuously monitoring and analyzing performance data to optimize operation. These systems can predict fluctuations in energy demand, assess the condition of turbine components, and adjust operations proactively. By analyzing real-time data from the turbine and the broader energy system, operators can anticipate changes in power needs or steam requirements and make the necessary adjustments before inefficiencies or disruptions occur. This proactive approach further enhances the turbine’s ability to adapt to dynamic conditions and ensures that energy production remains efficient, cost-effective, and sustainable.
Furthermore, extraction turbines provide value in highly regulated markets where operational flexibility can be a key competitive advantage. Many energy markets impose regulations that incentivize the reduction of fuel consumption, CO2 emissions, and waste. The operational flexibility of these turbines allows for optimized fuel use and reduced environmental impact. By adjusting their operation to minimize waste and maximize output based on real-time needs, these turbines help meet stringent emissions standards and reduce the carbon footprint of energy generation. In competitive markets where energy providers must continually optimize their operations to meet regulatory requirements and customer demands, the ability to flexibly manage both heat and power generation offers significant economic and operational benefits.
In the long term, as industries transition toward decarbonization goals, the role of operational flexibility in reducing greenhouse gas emissions becomes increasingly important. Extraction back-pressure turbines are essential in this transition due to their ability to minimize fuel consumption and maintain efficiency even as energy systems become more complex and diverse. By adapting to varying renewable energy inputs, managing fuel resources effectively, and maintaining optimal operation even during fluctuating demand, these turbines reduce reliance on fossil fuel-based backup generation. In doing so, they support both carbon reduction and the integration of cleaner, low-carbon energy sources into the grid.
Finally, as industries and energy systems continue to evolve, the ability to incorporate new technologies into existing turbine systems enhances their operational flexibility. For example, the integration of hydrogen as a fuel source or the adoption of advanced combustion technologies can be managed through the adaptable design of extraction back-pressure turbines. These turbines can be modified to operate with hydrogen or a mix of fuels, supporting the transition to greener energy solutions and enabling industries to meet future energy demands in a sustainable manner. The ability of these turbines to incorporate such innovations ensures that they remain relevant in the ever-changing energy landscape, providing a versatile and long-term solution for meeting both current and future energy needs.
In conclusion, the operational flexibility of extraction back-pressure turbines makes them indispensable in today’s dynamic energy environment. Their ability to adapt to fluctuating energy demands, integrate with renewable energy sources, optimize fuel usage, and maintain system stability in decentralized grids positions them as key players in sustainable energy systems. As energy systems become more complex and the transition to cleaner energy accelerates, these turbines will continue to offer the flexibility needed to ensure reliable, cost-effective, and environmentally friendly energy generation across a wide range of applications.
Continuing with the discussion on the operational flexibility of extraction back-pressure turbines, another key aspect is their role in facilitating energy system resilience and reliability, particularly in the face of unexpected disruptions or extreme events. In regions prone to natural disasters, such as hurricanes, floods, or earthquakes, energy infrastructure can be severely impacted, leading to power outages or disruptions in the supply of steam for industrial processes. In such situations, the ability of extraction back-pressure turbines to quickly adjust to changing operating conditions can be crucial in ensuring continued energy supply.
For instance, when a natural disaster leads to a disruption in the primary power grid or in the fuel supply chain, extraction back-pressure turbines can be integrated with backup generation systems or renewable energy sources to maintain a stable supply of electricity and steam. Their flexibility enables these turbines to seamlessly shift between different fuel sources or generation modes, which can be critical when traditional fuel supplies are unavailable or unreliable. In many cases, turbines can operate on alternative fuels, such as biofuels or waste-derived energy, to continue generating power and thermal energy, supporting vital infrastructure or industrial operations in the aftermath of a disaster.
Additionally, as energy systems are increasingly subject to cyber threats and vulnerabilities, the flexibility of extraction back-pressure turbines plays a role in enhancing the security and resilience of critical infrastructure. The ability to monitor and control turbine systems remotely using advanced digital control systems means that operators can rapidly adjust operations in response to cybersecurity threats or system anomalies. If a cyberattack disrupts grid operations or the functionality of other energy assets, extraction turbines can be quickly reconfigured to maintain power and heat production, minimizing the impact on essential services. The continued development of advanced monitoring, protection, and automation systems ensures that these turbines can respond to a broad range of potential security threats, contributing to the overall resilience of the energy system.
This ability to withstand disruptions is further supported by the use of predictive maintenance and real-time diagnostics, which are becoming increasingly prevalent in turbine systems. These advanced technologies enable the turbine to predict failures or potential malfunctions before they occur, allowing for preemptive adjustments that prevent downtime. By analyzing data from a variety of sensors embedded in the turbine and surrounding systems, operators can anticipate mechanical wear, inefficiencies, or potential failures, scheduling maintenance during non-peak hours and avoiding unplanned outages. This predictive capability ensures that the turbine operates at its highest efficiency while reducing the risk of unexpected downtime due to mechanical issues.
Moreover, the operational flexibility of extraction back-pressure turbines plays an essential role in the broader transition to a decentralized energy model. As more industries, municipalities, and even households seek to become energy self-sufficient by adopting microgrids or local power generation systems, these turbines can be integrated into such systems to provide localized energy solutions. In decentralized grids, where power generation and distribution are not centralized but instead occur in smaller, localized nodes, the turbine’s flexibility allows it to respond to local energy needs without compromising the reliability of the system. These turbines can provide both power and heat to local users, adjusting to fluctuating demands while maintaining the balance of energy supply and consumption within the microgrid.
In particular, this integration is valuable in areas where there is a push for energy independence or for reducing reliance on a centralized grid that might be prone to failure or inefficiencies. For example, in remote communities, mining operations, or offshore facilities, where access to the larger grid may be limited or expensive, extraction back-pressure turbines provide a solution for reliable and self-sustaining energy generation. These systems, which can seamlessly adapt to changes in energy demand and supply, help reduce the need for external energy imports and offer a cost-effective, reliable source of power and thermal energy, even in challenging conditions.
The flexibility of these turbines also supports their role in industrial applications where operational shifts or seasonal variations in energy demand are common. Industries such as food processing, paper manufacturing, and petrochemicals often experience fluctuating heating or power needs based on production schedules, seasonal output, or process changes. The ability of extraction back-pressure turbines to adjust to these dynamic requirements ensures that energy consumption is optimized, avoiding waste or overproduction. For example, during periods of low production or off-peak seasons, turbines can reduce their output, minimizing fuel use and operational costs. When production ramps up or heating demand increases, the turbines can quickly adjust to provide the necessary steam and power.
As industries continue to adopt more sophisticated production technologies, the demand for precise control over energy usage and quality is also growing. Modern extraction turbines are equipped with enhanced control systems that allow for highly refined adjustments in both steam extraction pressures and electrical output. This degree of control helps industrial operators fine-tune the energy supply to meet exact specifications for different processes, improving overall production efficiency and product quality. For example, certain chemical processes may require steam at very specific pressures or temperatures, which can be achieved through the fine-tuning capabilities of these turbines. Similarly, industries that rely on both heat and power for simultaneous processes can adjust the turbine’s operation to meet the needs of both without sacrificing efficiency or output.
Another significant benefit of the operational flexibility of extraction back-pressure turbines is their ability to support the implementation of energy-saving strategies and carbon reduction goals. As energy efficiency becomes a higher priority across sectors, these turbines are uniquely positioned to support initiatives aimed at reducing energy consumption and lowering carbon footprints. Through their adaptable steam extraction capabilities, turbines can adjust their operation to optimize both electrical and thermal generation, ensuring that energy is used only when and where it is needed. This can help avoid unnecessary energy waste, reduce fuel consumption, and lower greenhouse gas emissions.
For instance, in industrial applications, the integration of energy management systems with extraction turbines allows for real-time tracking of energy usage and the identification of inefficiencies. By monitoring the turbine’s performance and adjusting operational parameters accordingly, industries can achieve significant reductions in energy consumption. Similarly, in district heating systems, where thermal energy is often used for residential or commercial heating, turbines can adapt to changes in ambient temperatures and heating needs, reducing overall energy use and emissions.
As regulatory frameworks around carbon emissions become stricter and more companies and governments commit to achieving net-zero emissions, extraction back-pressure turbines can play a central role in meeting these targets. By adjusting their operation to optimize both thermal and electrical production, they contribute to a reduction in fossil fuel consumption and support the transition to cleaner energy sources. Whether by integrating renewable energy, improving fuel efficiency, or enhancing overall system performance, these turbines offer an effective tool for industries aiming to reduce their environmental impact while maintaining reliable energy supply.
In conclusion, the operational flexibility of extraction back-pressure turbines continues to provide essential benefits across a wide range of industries and applications. From their ability to adapt to fluctuating energy demands, integrate with renewable and alternative energy sources, and optimize fuel consumption, these turbines offer a versatile solution for modern, dynamic energy systems. As the global energy transition progresses, and as industries demand increasingly flexible, resilient, and sustainable energy solutions, extraction back-pressure turbines will remain a key component in achieving these goals. Their ability to adjust to real-time conditions, support decentralized energy systems, and reduce energy waste ensures their continued relevance in an evolving energy landscape.
Efficiency and Cost-Effectiveness of Extraction back-pressure Turbines
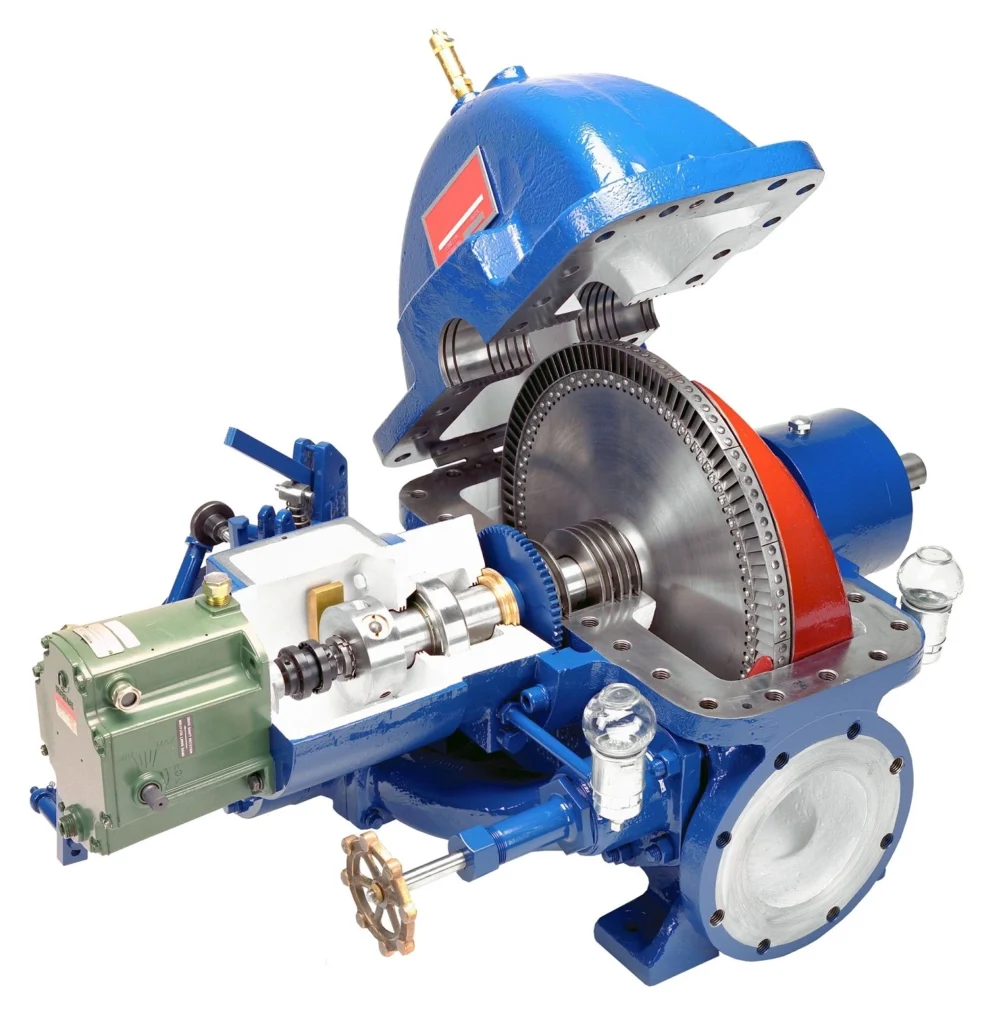
Extraction back-pressure turbines are integral to improving the efficiency and cost-effectiveness of industrial and energy generation systems. These turbines have been designed to meet the dual demands of power and thermal energy production, making them highly suitable for applications where both forms of energy are required simultaneously. The efficiency of extraction back-pressure turbines stems from their ability to produce electricity and useful heat from a single steam source, thus maximizing energy recovery from the fuel used to generate steam.
One of the primary advantages of these turbines is their ability to extract steam at multiple pressure levels during their operation, which allows them to adapt to different energy needs. By extracting steam at intermediate pressures, these turbines can provide a consistent flow of steam for industrial processes, while simultaneously generating electricity at the full back-pressure of the turbine. This extraction process allows for highly efficient use of steam, as it can be employed for various applications, including heating, cooling, or powering other machinery, without needing separate boilers or steam production systems. The result is a significant reduction in energy consumption, as steam is used more effectively throughout the system, rather than being wasted.
The efficiency of extraction back-pressure turbines also plays a key role in minimizing fuel consumption. Traditional power generation systems, which use separate steam boilers for electricity generation and industrial heating, often face inefficiencies because they produce steam for one purpose (electricity) and then discard or waste the excess thermal energy. In contrast, the dual function of the extraction back-pressure turbine—delivering both power and thermal energy from the same steam source—means that no energy is wasted. This approach enables a much higher overall system efficiency, reducing the need for additional fuel to meet heating or electricity demands. For industries where steam is already a critical part of the process, such as in chemical manufacturing, food processing, or paper production, using an extraction back-pressure turbine to generate both power and heat can lead to considerable savings in fuel costs.
In addition to enhancing overall system efficiency, extraction back-pressure turbines are also highly adaptable to varying load demands, which contributes to their cost-effectiveness. Many industries experience fluctuating energy demands throughout the day, with periods of high electricity consumption followed by times when power needs are lower. Extraction turbines can adjust their steam extraction levels based on the immediate needs of the process, optimizing both steam and electrical output. This ability to match energy production with demand ensures that turbines are only generating what is needed at any given time, reducing unnecessary energy production and associated fuel consumption. As a result, the turbine’s operational costs are lower, as energy is produced more efficiently and in response to actual demand, rather than being generated in excess.
The cost-effectiveness of extraction back-pressure turbines is further enhanced by their ability to integrate with existing infrastructure. Rather than requiring the construction of separate systems for steam and electricity generation, the turbine can be retrofitted into existing facilities that already use steam for industrial purposes. This eliminates the need for new infrastructure investments, such as building separate heating systems or additional power generation units, which can be costly and time-consuming. By leveraging existing steam systems and retrofitting a turbine, companies can realize immediate cost savings, avoiding the capital expenses associated with building new infrastructure. Additionally, the streamlined operation of these systems reduces maintenance costs, as there is less need for multiple boilers and separate generators to be maintained and operated.
The fuel flexibility of extraction back-pressure turbines also contributes to their cost-effectiveness. These turbines can operate on a variety of fuels, including natural gas, coal, biomass, or even waste heat, which gives operators the ability to choose the most economical or environmentally friendly option available. For example, when fuel prices fluctuate or when a facility has access to low-cost or waste-based fuels, the turbine can be adjusted to operate efficiently using the available resource, further lowering operational costs. In some cases, the use of biomass or waste products can even generate additional cost savings by reducing the need for disposal or transportation of waste, turning what would be a by-product into a valuable energy source.
Moreover, the ongoing operational costs of extraction back-pressure turbines are minimized through advanced control systems and digital technologies that help optimize performance and reduce inefficiencies. These systems can monitor the performance of turbines in real-time, identifying areas where improvements can be made, such as adjusting steam pressures or optimizing load balancing. Through predictive maintenance and diagnostic tools, potential issues can be detected before they result in significant downtime, thus reducing repair costs and extending the lifespan of the turbine. By proactively addressing maintenance needs, operators can ensure that turbines continue to operate at peak efficiency, thereby minimizing repair costs and avoiding unexpected outages.
In terms of lifecycle costs, the longevity of extraction back-pressure turbines also plays a significant role in their cost-effectiveness. These turbines are designed for durability and can operate for extended periods with minimal maintenance. The robust design and materials used in these turbines ensure that they are capable of withstanding the harsh operating conditions typically encountered in industrial applications, where temperatures, pressures, and mechanical stresses can be high. This durability translates into lower replacement and repair costs over the turbine’s operational life, which contributes to long-term cost savings for operators. Furthermore, the ability to adjust steam extraction pressures or change fuel sources allows operators to extend the turbine’s useful life by adapting to new energy requirements or advances in technology.
The economic benefits of extraction back-pressure turbines are particularly evident when considering their integration into combined heat and power (CHP) systems, which are increasingly being adopted to improve energy efficiency and reduce greenhouse gas emissions. CHP systems, which simultaneously generate electricity and useful heat, have been proven to offer one of the most efficient ways to produce energy. By using the heat produced during power generation for industrial processes, heating systems, or district heating networks, CHP systems reduce the need for additional fuel consumption, thereby cutting costs. Extraction back-pressure turbines are ideal for such applications because of their ability to provide both electrical and thermal energy in a coordinated, highly efficient manner. As energy efficiency standards become more stringent and carbon taxes become more prevalent, CHP systems equipped with extraction back-pressure turbines will become increasingly valuable for industries aiming to reduce costs while meeting regulatory requirements.
In regions where energy prices fluctuate or where energy security is a concern, extraction back-pressure turbines offer a buffer against volatility. By optimizing the use of available resources and reducing the reliance on external power supplies, these turbines allow facilities to mitigate the risks associated with rising energy prices or supply disruptions. Additionally, as industries are increasingly pressured to reduce their carbon footprint, the ability of these turbines to efficiently use renewable and low-carbon fuels provides a significant advantage in achieving sustainability goals while controlling operational costs.
In conclusion, the efficiency and cost-effectiveness of extraction back-pressure turbines make them a valuable component in modern energy systems. Their ability to simultaneously produce power and thermal energy from a single steam source significantly reduces fuel consumption and minimizes waste. The flexibility to adjust to varying demands and fuel sources enhances their adaptability and allows for further cost savings. Additionally, their integration with existing infrastructure, fuel flexibility, predictive maintenance capabilities, and long operational lifespan ensure that these turbines provide both short-term and long-term economic benefits. As industries continue to seek ways to optimize energy use and reduce costs while meeting sustainability targets, extraction back-pressure turbines offer a practical, efficient, and cost-effective solution.
Continuing with the discussion of the efficiency and cost-effectiveness of extraction back-pressure turbines, one of the notable advantages is their contribution to the overall reduction of operational waste. Traditional systems often generate excess heat that must be dissipated, leading to energy losses. With extraction back-pressure turbines, however, much of this heat is directly harnessed for other uses, such as heating processes in industrial operations or supplying district heating networks. This ability to utilize thermal energy that would otherwise be wasted ensures that nearly every unit of fuel burned is converted into useful energy, whether in the form of electricity or heat.
This reduction in waste not only enhances the efficiency of energy systems but also supports environmental goals. By minimizing the loss of energy, extraction back-pressure turbines reduce the carbon footprint of operations. This is particularly important as industries face increasing pressure to meet stringent environmental regulations and carbon emission targets. For companies seeking to demonstrate their commitment to sustainability, the integration of these turbines offers a straightforward and effective way to enhance energy efficiency while simultaneously lowering emissions.
The economic impact of reduced energy waste is substantial. In industries with high energy demands—such as those in chemical processing, steel production, and food manufacturing—significant amounts of energy are required for both electrical power and heating. The extraction back-pressure turbine’s ability to provide both in a single system reduces the need for separate heating systems or additional power generation equipment, leading to considerable savings in capital expenditures. Moreover, by maximizing the use of available steam, companies can reduce the amount of energy they need to purchase from the grid or other external suppliers, further lowering operational costs.
Another critical aspect of their efficiency is the turbine’s ability to operate in a range of conditions and at varying loads without sacrificing performance. The versatility of extraction back-pressure turbines allows them to adjust to fluctuations in demand for both steam and electricity, maintaining operational stability without requiring frequent shutdowns or changes in fuel supply. This load-following capability reduces the need for backup systems, which are typically used in systems without the operational flexibility of extraction turbines. By reducing dependency on supplementary power generation methods, extraction back-pressure turbines help lower the costs associated with maintaining additional equipment, further contributing to overall cost savings.
In addition to their inherent efficiency, extraction back-pressure turbines are compatible with digital control systems, which further enhance their performance. Advanced sensors, real-time monitoring, and data analytics allow for continuous optimization of the turbine’s operation. This means that even minor inefficiencies can be detected and corrected immediately, ensuring that the turbine is always operating at peak efficiency. Additionally, predictive maintenance technologies ensure that potential issues are identified before they lead to downtime or expensive repairs. This proactive approach to maintenance not only helps reduce the need for unscheduled maintenance but also extends the turbine’s lifespan, resulting in long-term savings on repair costs and equipment replacement.
Furthermore, the cost-effectiveness of extraction back-pressure turbines is amplified in the context of long-term investments. While the initial capital cost of installing such a turbine can be substantial, the return on investment (ROI) is typically realized over the course of several years through operational savings. The turbine’s high efficiency means that the system pays for itself through fuel savings, reduced energy purchases, and minimized maintenance costs. This is especially true for facilities with high energy consumption or those that are operating in regions with high fuel costs. When factoring in the additional environmental benefits and the potential to comply with regulatory requirements regarding emissions and energy efficiency, the installation of extraction back-pressure turbines becomes an attractive proposition for many industries.
The ability to harness waste heat and integrate with other systems, such as combined heat and power (CHP) setups, further enhances the turbine’s economic value. By using waste heat for additional processes, companies can offset their energy costs, reduce their environmental impact, and improve the overall economics of their operations. Whether through improving the thermal efficiency of industrial processes or providing district heating to local communities, extraction back-pressure turbines provide a wide array of opportunities for companies to optimize their energy use while simultaneously benefiting from cost reductions.
The cumulative effect of these efficiency and cost-saving measures creates a sustainable, economically viable energy solution. As industries continue to seek ways to reduce operational costs and increase sustainability, extraction back-pressure turbines offer a clear path toward achieving these objectives. Their ability to provide both power and heat from a single steam source, combined with their adaptability to varying operational conditions and fuel types, ensures they remain a central component in the energy strategies of industries worldwide.
In conclusion, the efficiency and cost-effectiveness of extraction back-pressure turbines are key reasons for their widespread adoption across various industries. These turbines maximize the use of steam, reducing energy waste and minimizing the need for additional fuel. Their ability to adjust to fluctuating energy demands, coupled with their integration with existing systems and modern digital technologies, makes them a highly adaptable and economically beneficial solution for power and thermal energy production. With long-term cost savings, environmental benefits, and improved operational flexibility, extraction back-pressure turbines offer a practical, efficient, and cost-effective energy generation solution for a wide range of applications.
Continuing from the previous discussion on the efficiency and cost-effectiveness of extraction back-pressure turbines, another critical benefit is the turbine’s role in enhancing overall system optimization. As industries face increasing pressure to improve energy efficiency while lowering costs, extraction back-pressure turbines contribute significantly to optimizing the entire energy system. By integrating both power generation and thermal energy production in a single system, these turbines reduce the need for separate, less efficient systems, resulting in lower operating costs. In many cases, the heat generated during power production can be used for additional heating or industrial processes, eliminating the need for independent boilers or heat exchangers.
This integrated approach also allows for improved load management. By combining steam extraction for both thermal and electrical uses, the turbine can modulate its output based on real-time demand fluctuations. Whether it’s adjusting to shifts in heating needs or electricity requirements, the turbine ensures that energy production is always aligned with the actual needs of the system, rather than operating at full capacity continuously. This dynamic load-following capability allows industries to avoid overproduction, reducing fuel consumption and operating costs, and ensuring that resources are utilized as efficiently as possible.
For industries where peak demand periods are common—such as in manufacturing facilities that experience spikes in energy usage during specific production cycles—the turbine’s ability to respond to demand changes quickly without compromising efficiency is crucial. Instead of relying on costly peak load power sources, which tend to be more expensive and less efficient, extraction back-pressure turbines provide a cost-effective solution. By producing only the necessary amount of energy at the right time, the turbines ensure that industries are not overspending on electricity during periods of high demand.
Moreover, the increased focus on decarbonization efforts and the transition to cleaner energy sources further enhances the value proposition of extraction back-pressure turbines. These turbines are well-suited for integration with renewable energy systems, such as biomass, geothermal, or solar thermal energy. Biomass, in particular, is an ideal fuel source for many industries that are looking to reduce their carbon footprint. Biomass can be used as a carbon-neutral energy source, helping industries meet sustainability targets while still benefiting from the efficiency and cost-effectiveness of extraction back-pressure turbines.
Additionally, as renewable energy technologies become more integrated into the broader energy grid, extraction back-pressure turbines can help balance the intermittent nature of renewable power generation. For instance, when renewable sources such as wind or solar are producing excess electricity, the turbine can be used to store thermal energy or adjust its operational parameters to accommodate the fluctuating supply. By providing flexibility and the ability to modulate energy output, extraction back-pressure turbines help stabilize the grid and ensure a continuous, reliable energy supply, particularly in regions where renewable energy plays a significant role in the energy mix.
On the economic side, the cost savings resulting from the efficient operation of extraction back-pressure turbines can lead to improved profitability for businesses. For industrial operations that rely heavily on steam for heating or power generation, reducing the need to purchase additional energy from external suppliers translates into substantial cost reductions over time. Furthermore, by operating more efficiently and with less waste, these turbines help improve the bottom line by lowering overall fuel consumption.
Beyond the direct savings from fuel and energy costs, extraction back-pressure turbines can also contribute to greater financial flexibility. By integrating heat recovery systems and enhancing the overall performance of energy infrastructure, these turbines can create new revenue opportunities. For instance, excess thermal energy can be used for district heating or sold to nearby facilities that require steam or heat, creating a secondary income stream. This ability to generate additional revenue from energy by-products enhances the financial feasibility of these turbines and provides a competitive advantage for companies in industries with fluctuating energy costs.
As companies become more focused on long-term cost reduction and environmental responsibility, the ability of extraction back-pressure turbines to deliver on both fronts makes them an attractive choice. The combination of lower capital investment requirements (due to integration with existing infrastructure), reduced fuel consumption, improved system optimization, and the potential for new revenue streams makes these turbines a highly cost-effective energy solution for a wide range of industrial applications.
For smaller operations or those just beginning to adopt energy-efficient technologies, extraction back-pressure turbines also present a relatively low-risk investment. The proven operational advantages, low maintenance requirements, and ability to provide both electrical power and useful thermal energy from a single source make them a wise choice for companies looking to enhance their energy systems without incurring excessive upfront costs. Over time, these turbines generate tangible cost savings that far exceed the initial investment, making them a financially sound and sustainable energy solution.
Another significant factor contributing to the cost-effectiveness of extraction back-pressure turbines is their relatively low maintenance costs compared to other types of turbines or energy generation systems. The advanced design of these turbines reduces wear and tear, extending their lifespan and reducing the frequency and expense of repairs. Moreover, the integration of digital monitoring and predictive maintenance technologies allows operators to detect potential issues before they result in costly repairs or system failures. By predicting when maintenance is required, businesses can plan downtime efficiently, avoiding disruptions to production and minimizing the impact of maintenance on overall operations.
The ability to maintain high efficiency over an extended operational life is another reason why extraction back-pressure turbines remain an attractive investment. The durability of the turbine and its components ensures that companies will continue to benefit from efficient performance for years, delivering ongoing savings on energy costs while requiring less frequent capital expenditures on replacements. This long-term financial advantage contributes to the overall economic appeal of these turbines, particularly for industries with high energy usage and a need for consistent, reliable power and thermal energy.
As energy markets continue to evolve, the role of extraction back-pressure turbines in helping industries reduce their operating costs while optimizing energy use is likely to become even more important. With rising energy prices, stricter environmental regulations, and a growing emphasis on sustainability, the demand for energy-efficient, cost-effective solutions will only increase. Extraction back-pressure turbines, with their ability to provide simultaneous power and thermal energy, optimize fuel use, and integrate with renewable sources, will continue to be a vital technology for industries looking to stay competitive in a rapidly changing energy landscape.
In conclusion, the efficiency and cost-effectiveness of extraction back-pressure turbines make them a critical asset for industries seeking to optimize energy use, reduce operational costs, and enhance sustainability. Their ability to generate both electricity and thermal energy from a single steam source significantly improves overall system efficiency and reduces waste, leading to lower fuel consumption and operational expenses. The turbines’ adaptability to fluctuating demands, ability to integrate with renewable energy sources, and capacity to reduce maintenance costs further enhance their cost-effectiveness. As industries increasingly prioritize efficiency and sustainability, extraction back-pressure turbines offer a practical, long-term solution for reducing energy costs while improving operational performance.
Continuing with the discussion of the efficiency and cost-effectiveness of extraction back-pressure turbines, another significant aspect that enhances their value proposition is their capacity to offer energy savings not just in the long term, but in the operational day-to-day running of a facility. This is particularly crucial in industries where energy consumption is a major part of operational expenditure. Through their design, extraction back-pressure turbines help companies minimize fuel consumption, thereby decreasing energy costs that would otherwise be spent on traditional power generation methods. By extracting steam at various pressure points, the turbine efficiently meets both the electricity and heating demands of an industrial system, all while avoiding the need for redundant or inefficient energy generation units.
One of the core advantages of extraction back-pressure turbines is their scalability. Whether applied to small manufacturing plants or large-scale industrial processes, these turbines can be sized to meet specific needs. This adaptability makes them an excellent option for facilities of varying capacities. For smaller operations, extraction back-pressure turbines can be used to ensure a balanced, efficient supply of both thermal energy and electricity without the need for multiple, costly systems. For larger, more complex systems, the turbines can be scaled up to handle significant volumes of steam, offering optimized performance that supports large-scale operations. The ability to scale turbines according to load requirements helps companies avoid the pitfalls of overpaying for energy capacity they don’t require or investing in systems that will eventually become underutilized.
Another contributing factor to their cost-effectiveness is the turbine’s enhanced reliability. Extraction back-pressure turbines are typically built to be more robust than conventional turbines, with a focus on long-term durability. This reliability leads to fewer unexpected maintenance issues or system failures, ultimately reducing downtime and the costs associated with repairs. In industries where production is continuous and downtime can result in significant financial losses, the operational stability of extraction back-pressure turbines is especially valuable. With fewer breakdowns and longer intervals between required maintenance, companies can keep their operations running smoothly without having to worry about costly interruptions.
When examining the total cost of ownership (TCO), which includes initial capital outlay, operational and maintenance costs, as well as the longevity of the system, extraction back-pressure turbines present a clear financial benefit. The upfront investment in a high-quality turbine may be higher than some other forms of energy generation equipment. However, the return on this investment is typically seen in the form of lower operational costs over time. Fuel savings, combined with reductions in maintenance and energy procurement costs, create a positive ROI, which becomes increasingly evident as the turbine continues to operate over the years. Additionally, the turbine’s ability to contribute to both heat and power generation optimizes operational costs, turning energy savings into more profitable operations.
The turbine’s environmental benefits further enhance its cost-effectiveness. As environmental regulations become stricter and companies face pressure to reduce emissions, adopting energy-efficient solutions like extraction back-pressure turbines helps organizations avoid penalties or carbon taxes. By optimizing fuel use and reducing emissions, companies can comply with sustainability standards and earn incentives, such as tax credits or subsidies, further enhancing the economic benefits of turbine adoption. Many regions offer incentives for adopting energy-efficient technologies, and extraction back-pressure turbines can make a company eligible for such rebates or benefits, reducing initial capital expenditures and boosting the overall financial viability of the project.
Another critical point is the potential for improved energy security. Many industries rely on stable and predictable energy costs to maintain their competitiveness and profitability. Fluctuations in energy prices can undermine profitability, especially for energy-intensive operations. Extraction back-pressure turbines, particularly when integrated with renewable or alternative energy sources, can provide a level of energy security and predictability. By reducing reliance on the grid and offering a more self-sufficient energy solution, companies are better insulated from energy price spikes or supply disruptions. This energy security is especially beneficial for industries located in areas with unstable power grids or regions where energy prices are volatile. Additionally, with the flexibility to switch between fuel types (such as natural gas, biomass, or waste materials), companies can further mitigate the risks associated with fuel supply disruptions or price fluctuations.
Moreover, the use of extraction back-pressure turbines in combined heat and power (CHP) systems ensures that both thermal and electrical energy needs are met without the need for separate systems. CHP systems are known for their efficiency, and when coupled with extraction back-pressure turbines, they can further optimize energy recovery. In industries such as chemical plants, food processing, or paper mills—where significant quantities of steam are required—these turbines can make full use of waste heat, turning it into additional useful energy. This heat recovery is another factor that helps keep operational costs low while simultaneously improving environmental performance. The efficient use of energy allows for the reduction of fuel needs, cutting operational expenses and lowering a company’s overall carbon footprint.
The operational flexibility of extraction back-pressure turbines plays a significant role in improving their cost-effectiveness, especially as industries face variable energy demands. Unlike traditional power plants that must maintain constant generation levels, extraction back-pressure turbines can adjust to the dynamic energy needs of the facility. If electricity demand is low, the turbine can divert more steam toward industrial heating needs, allowing for energy optimization. Conversely, if heating demand decreases, the turbine can increase electricity generation. This capacity to adjust to varying loads ensures that no energy is wasted, optimizing fuel usage and minimizing inefficiencies. Over time, this dynamic flexibility reduces the need for costly peak-load power generation or fuel purchases, directly lowering operational costs.
Furthermore, the integration of extraction back-pressure turbines with digital control systems and advanced monitoring technologies ensures that the turbine operates at peak efficiency throughout its lifecycle. These smart systems can track performance, predict failures, and automatically adjust operations to maximize output while minimizing fuel consumption. Predictive analytics can help detect performance deviations before they lead to costly downtime or repairs. This continuous optimization enhances the cost-effectiveness of the turbine, ensuring that operators are always working within the most efficient operating parameters.
In terms of installation, extraction back-pressure turbines offer significant savings when retrofitted into existing systems. Instead of requiring entirely new infrastructure, these turbines can often be integrated into existing steam systems, providing a cost-effective solution for companies looking to improve energy efficiency without the need for significant capital investment. This ease of retrofitting is particularly important in industries with established infrastructure that does not have the financial resources to overhaul their entire energy generation systems. By simply installing an extraction back-pressure turbine to replace or supplement existing equipment, companies can achieve substantial operational savings without undertaking extensive renovations or construction projects.
As industries continue to face the challenges of increasing energy demands, rising fuel prices, and stricter environmental regulations, the value of extraction back-pressure turbines as a reliable, efficient, and cost-effective energy solution will only continue to grow. With their ability to reduce waste, optimize fuel use, and generate both electricity and thermal energy from a single steam source, these turbines provide companies with a comprehensive solution that improves operational efficiency, reduces costs, and supports sustainability goals. As energy markets evolve and companies strive for energy independence, the role of extraction back-pressure turbines in enhancing both economic and environmental performance is more critical than ever.
Extraction Condensing Turbines
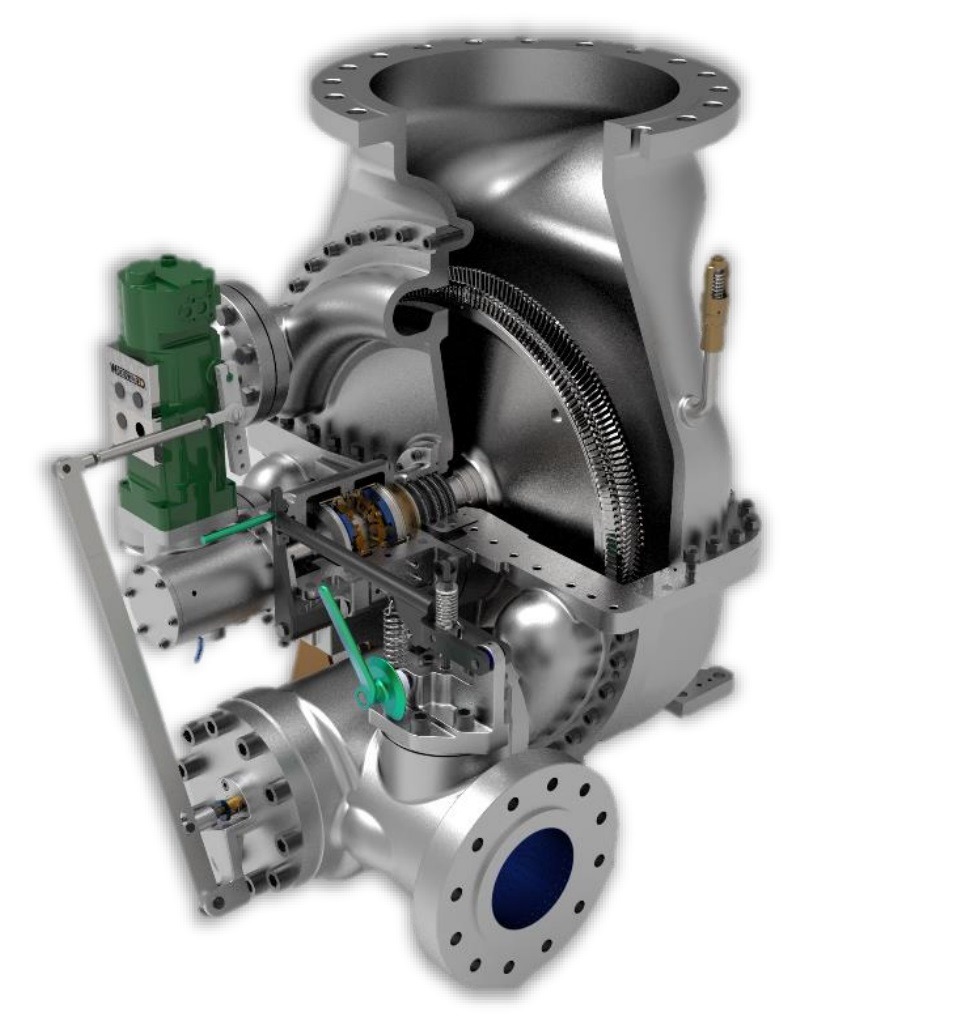
Extraction condensing turbines are widely used in power generation applications and industrial processes that require large amounts of electricity. These turbines operate on steam produced by a boiler or other heat source, where the steam expands through multiple stages in the turbine to generate power. The defining feature of extraction condensing turbines is the ability to extract steam from different stages within the turbine to be used for heating purposes or other industrial needs, while the remaining steam is allowed to condense at the final stage, often in a condenser, producing electricity.
The principle behind an extraction condensing turbine is based on thermodynamics, where the steam is first expanded through the turbine stages, losing pressure and temperature as it moves through. At certain points in the expansion process, steam is “extracted” for use in external processes, such as district heating, chemical processes, or any application that requires significant amounts of thermal energy. This ability to extract steam while still producing power makes the extraction condensing turbine highly versatile and suitable for facilities that need both electricity and thermal energy.
One of the key benefits of extraction condensing turbines is their efficiency in utilizing available energy. As steam expands through the turbine, it undergoes a drop in pressure and temperature, which can be harnessed in multiple ways. The extracted steam can be diverted to heat exchangers or industrial applications, allowing the plant to recover heat that would otherwise be wasted in a typical condensing turbine setup. This heat recovery mechanism improves overall system efficiency by utilizing the thermal energy for productive purposes, minimizing energy loss, and optimizing the use of fuel sources.
In addition to enhancing thermal efficiency, extraction condensing turbines are also capable of operating in various load conditions, which is a crucial factor in modern power generation. The flexibility of these turbines allows them to adjust the amount of extracted steam based on real-time demand for heating or electricity. This dynamic operation ensures that the turbine operates efficiently under a wide range of conditions, which is especially important for facilities with fluctuating energy needs. For instance, when electricity demand is high, more steam can be directed towards electricity generation, while during periods of low electricity demand, more steam can be diverted for heating purposes without compromising system performance.
Another important aspect of extraction condensing turbines is their contribution to grid stability and the balance between electricity and thermal energy demands. In regions where combined heat and power (CHP) systems are prevalent, these turbines allow for seamless integration into existing infrastructure, providing both heat and power in a synchronized manner. In CHP applications, the heat that would otherwise be wasted in the condensation process is repurposed, creating a more efficient energy system. This dual functionality significantly reduces the reliance on external power sources for heating, providing a more sustainable and cost-effective solution.
Moreover, the integration of extraction condensing turbines with advanced control systems and digital technologies can optimize the turbine’s performance in real-time. Sensors, monitoring equipment, and predictive maintenance systems can track the turbine’s operational efficiency, identify potential issues, and enable adjustments before problems arise. These technologies can also help ensure that the turbine operates within its optimal performance parameters, reducing the risk of mechanical failure, extending the lifespan of the turbine, and reducing operational costs.
The use of extraction condensing turbines is not limited to power plants alone. Many industrial sectors, such as chemical, petrochemical, and food processing industries, benefit from the dual purpose of these turbines. In chemical plants, for example, the ability to provide both power and heat makes the extraction condensing turbine a valuable tool for maintaining continuous production processes. Similarly, in food processing plants, the extraction of steam for heating purposes helps in maintaining stable operating conditions, especially when processes such as sterilization or drying are required. These applications enhance the overall economic viability of the turbine, as it not only generates electricity but also serves as a critical component of an industry’s heating system.
One of the most significant advantages of extraction condensing turbines is their ability to adapt to varying fuel types. While traditionally powered by fossil fuels, such as coal, oil, or natural gas, these turbines can also be adapted to work with renewable fuels, such as biomass or biogas. The ability to switch between different fuels enables operators to adjust their energy sources based on availability, cost, and sustainability goals. This flexibility is crucial as industries seek to reduce their carbon footprints and embrace cleaner, more sustainable energy practices. As renewable energy becomes more widely adopted, the extraction condensing turbine’s compatibility with these fuel types allows it to remain relevant and effective in a rapidly changing energy landscape.
Despite the clear benefits, the initial capital investment required for extraction condensing turbines can be a significant consideration. The installation of these turbines requires substantial infrastructure, including steam generation systems, condensers, and control systems, which can add to the overall cost of the project. However, these costs are often mitigated by the long-term savings in fuel and operational efficiency, as well as the potential to generate additional revenue through the sale of excess thermal energy. In regions where energy costs are high or where there is strong demand for district heating, the financial benefits of extraction condensing turbines can outweigh the initial investment.
In conclusion, extraction condensing turbines are an effective solution for industries and power plants that require both electricity and thermal energy. By extracting steam from different stages of the turbine to be used for heating or industrial processes, these turbines increase energy efficiency by utilizing thermal energy that would otherwise be wasted. Their adaptability to fluctuating energy demands, compatibility with various fuel types, and integration with advanced control systems make them highly versatile and cost-effective. Whether used in large-scale power generation or smaller industrial applications, extraction condensing turbines offer a reliable, sustainable, and efficient way to meet both electricity and heating needs. Their ability to optimize fuel use, recover waste heat, and provide energy security positions them as a key technology in the ongoing transition towards cleaner and more efficient energy systems.
Continuing with the discussion on extraction condensing turbines, it is important to highlight the operational flexibility that these turbines offer in terms of adapting to varying conditions. One of the core reasons they are highly valued in energy systems is their ability to balance the needs of electricity generation with thermal energy demands. As previously mentioned, the extraction of steam at different pressure stages allows for the delivery of both power and heat without requiring separate systems for each, thus streamlining the overall energy infrastructure. This flexibility is especially important in environments where energy demand fluctuates throughout the day or during seasonal changes.
For instance, during periods of high electricity demand, the extraction condensing turbine can prioritize the generation of electrical power by extracting less steam or redirecting more steam to the condensing process, where it is cooled and converted back into water for reuse. Conversely, during low electricity demand, the turbine can adjust by redirecting more steam for heating or other industrial applications, reducing the waste of potentially valuable energy and ensuring that the system remains adaptable to changing requirements. This responsiveness to load changes is a key factor that enhances operational efficiency and reduces costs, as it allows the turbine to optimize its output based on real-time needs.
Furthermore, extraction condensing turbines can offer significant benefits in terms of grid stability and energy management. The ability to adjust the steam extraction and condensing rates means that the turbine can help smooth out fluctuations in electricity generation, which is particularly valuable when integrated with renewable energy sources. For example, in regions where solar or wind energy is prevalent, energy production can be variable depending on weather conditions. The extraction condensing turbine can act as a balancing mechanism, compensating for these fluctuations by either adjusting its power generation capacity or redirecting excess thermal energy to other uses, helping to stabilize the overall grid and improve energy security.
Another key characteristic of extraction condensing turbines is their ability to integrate with advanced energy management and automation systems. These systems can monitor the turbine’s performance in real-time, optimizing steam extraction rates and adjusting operational parameters as needed to ensure maximum efficiency. By providing operators with detailed, data-driven insights into the turbine’s performance, these systems can help minimize energy losses and reduce the risk of operating the turbine outside its optimal performance range. Predictive maintenance algorithms can also alert operators to potential issues before they result in expensive breakdowns or downtime, reducing maintenance costs and extending the turbine’s service life.
The integration of digital technologies not only enhances operational efficiency but also supports the broader push towards sustainability. By optimizing the use of thermal and electrical energy, extraction condensing turbines help reduce the reliance on non-renewable energy sources and minimize greenhouse gas emissions. Additionally, the recovery of waste heat for use in other processes contributes to the overall sustainability of industrial operations, allowing facilities to operate more efficiently and with a smaller environmental footprint. As industries face increasing regulatory pressures to reduce emissions and improve their environmental performance, extraction condensing turbines provide a viable solution that balances energy generation with environmental stewardship.
The cost-effectiveness of extraction condensing turbines is further emphasized when considering their adaptability to various fuel types. In addition to traditional fossil fuels such as coal, oil, or natural gas, these turbines can be operated using alternative fuels, including biomass, biogas, or even waste materials. This flexibility provides facilities with the opportunity to reduce their reliance on expensive and carbon-intensive fossil fuels, enabling them to explore more sustainable and locally sourced alternatives. This not only helps lower energy costs but also supports a transition toward more sustainable energy systems.
Additionally, the ability to generate electricity and thermal energy simultaneously makes extraction condensing turbines particularly valuable in combined heat and power (CHP) applications. In industries where both electricity and heat are required, the simultaneous generation of both forms of energy from a single turbine reduces the need for separate power and heating systems. This not only improves energy efficiency but also minimizes capital investment and maintenance costs. By optimizing the use of fuel and heat recovery, extraction condensing turbines ensure that industries can meet their energy needs with fewer resources, resulting in long-term financial savings.
In terms of installation and integration into existing systems, extraction condensing turbines are highly versatile. While the initial capital investment required for these turbines can be significant, their ability to be retrofitted into existing steam systems makes them an attractive option for facilities looking to improve energy efficiency without overhauling their entire infrastructure. Many plants, particularly in sectors such as chemical manufacturing or food processing, already have steam generation systems in place. By replacing or supplementing existing turbines with extraction condensing turbines, these facilities can enhance their overall energy efficiency without incurring the high costs of building entirely new energy systems.
Moreover, the ability to recover waste heat through extraction condensing turbines can also open up new revenue streams for businesses. In industries where there is excess thermal energy, this heat can be sold to neighboring facilities or used in district heating systems. This additional revenue stream can help offset the initial installation costs of the turbine, further improving its cost-effectiveness and enhancing the financial benefits of adopting this technology.
The combination of electricity and heat generation in a single system also allows extraction condensing turbines to offer a high level of energy independence. By reducing reliance on external electricity or heating sources, facilities can better control their energy costs, insulate themselves from price fluctuations, and ensure a more stable energy supply. This is particularly important in regions where energy prices are volatile or where the availability of reliable energy is a concern.
Another significant advantage of extraction condensing turbines is their ability to deliver consistent and reliable performance over time. With proper maintenance and regular monitoring, these turbines can operate for many years, providing a steady source of power and heat while keeping operational costs low. The long lifespan of extraction condensing turbines, combined with their relatively low maintenance requirements, makes them an attractive investment for businesses seeking long-term solutions to their energy needs.
In conclusion, extraction condensing turbines represent a highly effective and flexible solution for industries and power plants that require both electricity and thermal energy. Their ability to extract steam for external use, recover waste heat, and adjust to varying energy demands makes them an ideal choice for optimizing energy systems. With the integration of advanced control systems, predictive maintenance technologies, and the ability to operate with a range of fuel types, extraction condensing turbines provide substantial cost savings, operational flexibility, and environmental benefits. By improving energy efficiency, reducing emissions, and offering a reliable source of both electricity and heat, these turbines play a crucial role in helping industries meet their energy and sustainability goals. As the energy landscape continues to evolve, extraction condensing turbines will remain an important technology for achieving energy security, reducing costs, and advancing toward a more sustainable future.
As we continue exploring extraction condensing turbines, it becomes evident that their versatility goes beyond energy efficiency and cost-effectiveness. Another significant factor is their contribution to optimizing resource utilization, particularly in industries where steam and power are integral to the production process. This optimized use of resources is achieved through the turbine’s ability to efficiently generate both electricity and thermal energy from the same steam source, which minimizes energy wastage and reduces the need for separate, dedicated systems for power and heat generation.
Industries that rely heavily on steam for their processes, such as the paper and pulp, chemical, and food processing sectors, benefit from extraction condensing turbines because of their ability to deliver steam at the required pressures for both power generation and heating. In these industries, heat is often used for heating liquids, maintaining process temperatures, drying, or even sterilizing products. By using the turbine to extract steam at various points, facilities can ensure that the right amount of steam is directed where it is most needed, improving the overall balance between power and heat demand. This balance can be particularly valuable in systems where load requirements fluctuate, such as in district heating or seasonal production processes, where energy needs may vary throughout the year.
One of the main advantages in industries where these turbines are applied is the reduction of waste heat. In typical power generation systems, the heat left over after generating electricity is usually discarded through cooling towers or other means, which is an inefficient process. However, with extraction condensing turbines, waste heat is recovered and used for productive purposes, reducing energy consumption and making processes more sustainable. This heat recovery mechanism directly contributes to reducing fuel consumption by ensuring that the thermal energy produced during the turbine’s operation is not wasted. The use of this waste heat for process heating or district heating can significantly cut down the overall energy costs for companies, particularly in industries where heat is a major part of the production process.
Moreover, extraction condensing turbines contribute to reducing the environmental impact of industrial operations. Given the increasing emphasis on environmental sustainability and meeting stricter emissions regulations, many industries are looking for ways to reduce their carbon footprint. The ability of extraction condensing turbines to maximize the use of thermal energy by diverting it for external use helps reduce the overall demand for fuel, lowering the emissions associated with power generation. This heat recovery feature makes extraction condensing turbines a more environmentally friendly option, as the systems can provide both heat and power without increasing fuel consumption or carbon emissions. In a world where companies are increasingly being held accountable for their environmental impact, these turbines offer a practical solution to meet both economic and regulatory demands.
As the energy landscape evolves, the need for flexibility in power generation systems continues to grow, and extraction condensing turbines meet this demand with their adaptable nature. These turbines can be employed in both base-load and peaking power applications, providing flexibility in managing variable energy sources like solar and wind. When integrated into combined heat and power (CHP) systems, extraction condensing turbines allow for efficient operation, even when the supply of renewable energy is intermittent. For example, when solar or wind generation is high, the turbine can focus more on producing electricity, while at times when renewable generation is lower, it can produce both heat and power as required.
Furthermore, extraction condensing turbines are increasingly being coupled with advanced energy storage systems. The ability to store energy when production exceeds demand and release it when required allows facilities to better manage their energy usage and reduce reliance on external sources. In combination with battery storage systems or thermal energy storage, these turbines help maintain a continuous, stable supply of energy, smoothing out fluctuations in demand and generation. This integration with energy storage systems ensures that companies can make the most efficient use of their energy resources, reducing their reliance on grid-supplied electricity and lowering energy costs.
Another emerging trend is the role of extraction condensing turbines in the transition to more sustainable and decentralized energy systems. As the global energy market shifts toward renewables and distributed generation, these turbines can be utilized in smaller, decentralized energy systems, such as microgrids. Microgrids allow for the generation of energy at the point of use, enabling facilities to become more self-sufficient in terms of power and heat production. By integrating extraction condensing turbines into these systems, businesses can not only increase their energy resilience but also take advantage of renewable or locally sourced fuels to further reduce their environmental impact. This decentralized approach enhances energy security and ensures that facilities are less dependent on external power sources, contributing to a more reliable and sustainable energy system overall.
The technological advancements in turbine design and control systems have also made extraction condensing turbines more efficient and easier to operate. Modern turbines are often equipped with advanced sensors, automation systems, and artificial intelligence (AI)-driven optimization software that enable operators to monitor performance in real time and make adjustments as necessary. These systems provide continuous feedback on parameters such as steam flow rates, turbine efficiency, and temperature, ensuring that the turbine operates within the most efficient parameters. The ability to make data-driven adjustments not only improves the efficiency of the turbine but also allows for predictive maintenance, reducing the risk of unexpected breakdowns and costly repairs. Predictive maintenance tools can analyze the performance data and detect anomalies before they lead to mechanical issues, enabling maintenance teams to address problems proactively, minimizing downtime, and extending the life of the turbine.
The combination of energy efficiency, operational flexibility, and environmental benefits makes extraction condensing turbines particularly well-suited for industries seeking to meet both economic and sustainability goals. As industries face increasing pressure to reduce energy consumption and limit their environmental impact, the ability of these turbines to provide both heat and power from a single system offers a significant advantage. By optimizing energy use and reducing waste heat, extraction condensing turbines contribute to improved financial performance while supporting environmental initiatives.
In conclusion, extraction condensing turbines represent a powerful and flexible solution for industries looking to meet their energy needs efficiently. By integrating power generation and heat recovery into a single system, these turbines provide significant cost savings, improve energy security, and reduce environmental impact. Their adaptability to fluctuating energy demands, compatibility with renewable fuels, and integration with advanced control systems make them an essential component of modern energy systems. Whether used in large-scale power generation or smaller industrial applications, extraction condensing turbines offer a reliable and sustainable way to generate both electricity and heat, helping industries achieve their energy, economic, and environmental goals.
As we continue to explore the numerous advantages and functionalities of extraction condensing turbines, it becomes apparent that these systems are integral to the long-term sustainability and efficiency of energy production, particularly in industries where both heat and power are required. The growing focus on sustainability, resource optimization, and reducing environmental impact presents new challenges and opportunities for industries, and extraction condensing turbines provide an effective means to address these needs.
In the context of industrial processes, one of the major benefits of extraction condensing turbines is their ability to serve as a cost-effective solution for facilities that require continuous or large-scale steam for various processes. Industries such as chemicals, pharmaceuticals, textiles, and food processing often require steam at different pressures for different stages of their production processes. By incorporating extraction condensing turbines, these industries can optimize steam generation and supply to meet the varying demands without needing to invest in multiple heating systems or separate boilers for different pressure levels. This integrated system provides significant cost savings by minimizing the need for multiple energy sources and reducing operational complexity. Furthermore, steam extraction ensures that thermal energy is not wasted but rather utilized for productive purposes, creating additional value for the facility.
Another advantage of extraction condensing turbines is their ability to scale with the energy demands of industrial applications. In many large-scale industrial settings, energy demands fluctuate due to changes in production schedules, seasonal factors, or varying operational hours. Extraction condensing turbines, with their flexible steam extraction capability, can be easily adjusted to accommodate these changing requirements. Whether more power is needed or additional steam is required for heating, these turbines provide the ability to adapt to the shifting needs of the facility. This ensures that industries can maintain stable operations while optimizing fuel use, reducing waste, and ensuring that both power and heat are produced as efficiently as possible.
The ability to produce both power and thermal energy also enhances the overall resilience of industrial operations. In industries where energy disruptions or unreliable grid connections can lead to costly downtime or interruptions, extraction condensing turbines provide a reliable and self-sufficient energy source. For example, in remote locations where the electrical grid may be unstable or difficult to access, a self-contained energy system that produces both power and heat from a single turbine can ensure that operations continue smoothly. Furthermore, in regions where energy prices are subject to significant fluctuations, extracting steam for industrial heating can provide an alternative to more expensive heating fuels or grid-based electricity, thereby reducing operating costs and increasing the overall stability of the energy supply.
The integration of extraction condensing turbines into combined heat and power (CHP) systems has been another game changer for industries looking to increase energy efficiency while minimizing environmental impact. CHP systems are designed to capture the waste heat from power generation and use it for other productive purposes, such as space heating, process heating, or water heating. This “cogeneration” concept ensures that the maximum amount of energy is recovered and used productively, instead of being wasted. In this context, the extraction condensing turbine’s ability to generate both power and heat simultaneously makes it an essential component of any CHP system. The turbine’s dual function ensures that a greater portion of the fuel’s energy is converted into usable work, reducing the overall energy consumption of the facility.
As industries increasingly embrace sustainable practices and work to reduce their carbon footprints, extraction condensing turbines align well with these goals by offering a more environmentally friendly alternative to traditional heating and power generation systems. By maximizing energy efficiency, reducing fuel consumption, and minimizing emissions, extraction condensing turbines help companies meet stricter environmental regulations while simultaneously improving their bottom line. In industries where fuel costs represent a significant portion of operational expenses, the efficiency of these turbines directly translates into cost savings that can be reinvested into other areas of the business.
The role of extraction condensing turbines in supporting the transition to renewable energy sources cannot be overstated. As industries and utilities seek to integrate renewable energy into their operations, extraction condensing turbines can be adapted to work with various renewable fuels, such as biomass, biogas, or even waste-to-energy systems. These turbines can operate on renewable resources while still maintaining the same high level of efficiency that is typically associated with fossil fuel-based systems. In areas where access to renewable fuels is abundant, such as regions with large-scale agricultural or forestry operations, extraction condensing turbines provide a viable option for sustainable power and heat generation.
Additionally, the integration of these turbines with renewable energy systems enhances grid stability by providing a flexible backup power source. When intermittent renewable energy sources like wind or solar are unavailable or unable to meet demand, the turbine can step in to provide both electricity and heat to the system. This dual-functionality helps stabilize the grid and ensures that energy production continues even when renewable sources are insufficient. This is particularly valuable in areas that rely heavily on renewable energy and require backup systems to prevent power shortages or blackouts.
In addition to being environmentally friendly, extraction condensing turbines also offer significant operational advantages due to their ability to be remotely monitored and controlled through advanced digital technologies. Modern turbines are often equipped with sensors, real-time monitoring systems, and predictive analytics that allow operators to optimize turbine performance, identify potential problems early, and reduce the risk of system failure. This capability not only improves efficiency but also reduces the need for manual intervention, enabling operators to focus on more strategic tasks. Furthermore, predictive maintenance technologies help extend the lifespan of the turbine, ensuring that it continues to operate at peak efficiency throughout its service life.
The long-term financial advantages of extraction condensing turbines make them a smart investment for industries aiming to improve energy efficiency, reduce costs, and enhance sustainability. By generating both electricity and thermal energy in a single system, these turbines eliminate the need for separate power and heating systems, reducing capital and operational costs. In addition, their ability to operate with a variety of fuel sources, including renewable fuels, positions them as a key technology for industries seeking to diversify their energy supply while reducing their environmental impact.
Moreover, as global energy prices continue to rise and the demand for more sustainable energy solutions intensifies, extraction condensing turbines offer industries the opportunity to gain greater control over their energy usage and costs. With their ability to optimize steam production, recover waste heat, and provide flexible, reliable energy solutions, these turbines allow businesses to remain competitive in an increasingly cost-conscious and environmentally aware market.
In conclusion, extraction condensing turbines are a critical technology for industries looking to optimize energy production, reduce costs, and meet sustainability goals. By integrating electricity generation and heat recovery into a single system, these turbines maximize fuel efficiency and minimize waste, providing both economic and environmental benefits. Their ability to operate with various fuel types, their flexibility in meeting fluctuating energy demands, and their role in supporting renewable energy integration make them essential components of modern energy systems. As industries face increasing pressure to improve energy efficiency, reduce emissions, and adapt to changing energy landscapes, extraction condensing turbines will continue to be a valuable solution for balancing power and heat needs in a sustainable and cost-effective manner.
Here are the four main parts of extraction condensing turbines:
- Turbine Rotor and Blades: The rotor and blades are the primary components responsible for converting the energy from steam into mechanical energy. The steam flows over the turbine blades, causing the rotor to spin, which in turn generates mechanical power. The design of the blades and rotor is critical for optimizing the efficiency of the turbine, as they need to be able to handle varying steam pressures and temperatures across different stages of extraction.
- Extraction System: The extraction system allows steam to be removed from the turbine at specific pressure levels for external use, such as process heating or district heating. Depending on the turbine design, steam can be extracted at one or more points, providing flexibility in managing both electricity generation and thermal energy needs. This system enables the turbine to serve dual functions, producing power while also supplying steam for industrial processes or other applications.
- Condenser: The condenser is responsible for cooling the exhaust steam after it has passed through the turbine and turning it back into water. This process maximizes the efficiency of the turbine by maintaining low back-pressure and ensuring that the steam can be reused in the system. The condenser is typically water-cooled and plays a crucial role in maintaining the thermal cycle by allowing the turbine to operate efficiently over long periods.
- Control and Monitoring Systems: Advanced control systems are integrated into extraction condensing turbines to monitor and optimize performance in real-time. These systems ensure that the turbine operates within its optimal efficiency range by adjusting parameters such as steam flow, temperature, and pressure. Modern turbines often include digital sensors and predictive maintenance tools, allowing operators to detect issues early, make data-driven adjustments, and maximize the lifespan and reliability of the turbine.
These four parts work together to ensure that extraction condensing turbines can efficiently produce both electricity and heat, while offering flexibility in energy generation and contributing to overall system efficiency.
Turbine Rotor and Blades

The turbine rotor and blades are the most fundamental components of any steam turbine, including extraction condensing turbines. These parts are responsible for converting thermal energy from steam into mechanical energy, which can then be used for power generation or other industrial processes. The rotor is essentially the core of the turbine, as it is the rotating shaft that drives the generator or connected mechanical systems. The blades, which are attached to the rotor, are the critical elements that interact with the steam to extract energy.
The function of the turbine blades is to direct the flow of steam onto the rotor in such a way that the force of the steam causes the rotor to rotate. As steam expands through the turbine stages, its energy is transferred to the blades, which are designed with a specific geometry to maximize efficiency. These blades must be engineered to withstand the high temperatures and pressures of the steam, as well as the mechanical stresses generated by the forces of rotation. Because the efficiency of a steam turbine is highly dependent on the interaction between the steam and the blades, the design and materials of the rotor and blades play a pivotal role in the overall performance of the turbine.
The rotor itself is usually constructed from high-strength alloys that can endure the mechanical stresses and thermal conditions encountered during turbine operation. These materials must also be resistant to corrosion and erosion, given the nature of steam and the impurities it may carry, such as moisture or particles. In some designs, the rotor may be divided into several sections, allowing for multiple stages of steam expansion, which enables the turbine to extract energy more efficiently. Each stage consists of a set of blades that progressively reduce the steam’s pressure and temperature while converting its thermal energy into rotational mechanical energy.
Turbine blades are typically made from advanced materials like stainless steel, nickel-based alloys, or other high-performance materials that can resist the harsh operating conditions of the turbine. These materials are chosen not only for their strength and durability but also for their ability to maintain performance under high temperatures. The blades are often coated or treated with specialized surface coatings to improve their resistance to oxidation, corrosion, and wear, especially in areas where steam enters the turbine with a higher moisture content. The blade design itself is optimized through sophisticated computational methods to ensure that the steam flows smoothly over the surface, minimizing losses due to turbulence and maximizing energy extraction.
The blades are also designed to operate at varying steam pressures and temperatures as they progress through different stages of the turbine. In an extraction condensing turbine, where steam is extracted at various points for external use, the blades are designed to handle the changing conditions of steam flow. For example, when steam is extracted at an intermediate stage for heating purposes, the remaining steam will have lower pressure and temperature as it enters subsequent stages of the turbine. The blades in these later stages must therefore be optimized to handle lower pressure steam without sacrificing efficiency.
Over time, turbine blades are subject to wear and tear, as the mechanical stresses of rotation and the high-velocity steam flow gradually erode the surfaces of the blades. This erosion can be caused by factors such as water droplets in the steam, which can physically impact the blades, or by corrosion from the chemical composition of the steam. To mitigate these effects, regular maintenance and inspection of the turbine rotor and blades are essential. In many turbines, blades are designed with replaceable tips or coatings that can be refreshed or replaced as needed, extending the operational life of the turbine.
Another critical aspect of the turbine blades and rotor is the maintenance of the optimal balance between steam velocity and blade rotation speed. If the steam is allowed to flow too quickly over the blades or at the wrong angle, it can cause the blades to wear out more quickly or reduce the efficiency of the turbine. Similarly, if the blades are not properly aligned with the steam flow, energy is lost, and the turbine operates less efficiently. For this reason, modern turbine designs often employ advanced computational fluid dynamics (CFD) models and sophisticated sensors to continually monitor the flow of steam and make adjustments to optimize performance.
The rotor and blades are not only essential for the power generation aspect of the turbine but also contribute to the overall operational efficiency of the system, especially when part of a combined heat and power (CHP) system. In extraction condensing turbines, where steam is extracted at different points for external use, the rotor and blades play a crucial role in ensuring that the turbine delivers the right amount of energy to meet the dynamic demands of both electricity generation and thermal applications. The ability of the blades to handle varying steam conditions ensures that the turbine remains adaptable to the shifting needs of the process while maintaining high levels of efficiency.
The performance of the turbine rotor and blades is also affected by the control systems and sensors that monitor the operation of the turbine. These systems ensure that the turbine is operating within its optimal parameters by adjusting steam flow, temperature, and pressure in real-time. For example, if the steam entering the turbine becomes too dry or too moist, the control systems can adjust the operation to prevent blade erosion or mechanical damage. Through these automated systems, the turbine’s rotor and blades can be kept in an ideal operating condition, which in turn maintains the overall efficiency and longevity of the turbine.
In summary, the turbine rotor and blades are crucial to the functioning of steam turbines, particularly in extraction condensing turbines, where they serve a central role in converting steam energy into mechanical power. The design, materials, and maintenance of these components directly affect the turbine’s efficiency, durability, and adaptability to varying steam conditions. Their ability to withstand high temperatures, pressures, and mechanical stresses while maintaining optimal performance makes them vital for the long-term success of steam turbine systems.
The rotor and blades in a steam turbine play a vital role not only in energy conversion but also in ensuring the turbine operates efficiently under a variety of conditions. Their ability to extract energy from steam at various pressures and temperatures is crucial for meeting the specific energy demands of the system. In the case of extraction condensing turbines, which extract steam at various points for external use, these components are designed to handle fluctuating steam conditions without compromising performance.
One of the key design features of turbine blades is their aerodynamic shape, which is carefully engineered to optimize steam flow. The steam entering the turbine undergoes a significant drop in pressure and temperature as it moves through the stages of the turbine. The blade design is tailored to harness the energy from this expanding steam and transfer it efficiently to the rotor. To achieve maximum energy conversion, the blades are shaped to control the steam’s velocity and pressure, ensuring that it flows smoothly over the blade surface while minimizing energy losses due to turbulence.
The rotor, on the other hand, is designed to rotate smoothly as it is acted upon by the steam flow. The rotation of the rotor is what ultimately drives the generator or mechanical load connected to the turbine. The rotor must be balanced carefully to ensure that it operates without vibration, which could cause mechanical damage or reduce the efficiency of the turbine. Precision in balancing the rotor is achieved through careful design, material selection, and alignment during manufacturing, as well as through ongoing monitoring and maintenance during operation.
In high-performance turbines, the rotor and blades are subject to extreme operating conditions, including temperatures that can reach several hundred degrees Celsius and pressures that can be many times higher than atmospheric levels. As such, the materials used for the rotor and blades must be carefully selected for their ability to withstand these harsh conditions. High-temperature alloys, such as nickel-based or stainless-steel alloys, are commonly used for their strength, durability, and resistance to corrosion and oxidation. These materials ensure that the rotor and blades maintain their structural integrity over time, even in the face of continuous exposure to high-temperature steam.
Another important factor in rotor and blade design is the control of thermal stresses. As the blades are subjected to both high temperatures from the steam and mechanical stresses from the rotating rotor, managing the temperature gradient across the blade material is critical. Sudden temperature changes can lead to thermal expansion or contraction, which can cause cracks or deformations in the blades. To minimize this risk, advanced cooling techniques, such as internal cooling passages or coatings, are sometimes employed to regulate the temperature distribution across the blades. This helps to maintain the longevity and operational reliability of the turbine.
The rotor and blades also undergo constant wear and tear due to the high-speed rotation and the abrasive effects of the steam. The steam in the turbine may contain moisture or particles that can erode the surface of the blades over time. To combat this, blades are often designed with wear-resistant coatings or are made from materials that can better resist erosion. Additionally, the blades are periodically inspected and replaced as needed to ensure the turbine maintains its peak efficiency.
In summary, the rotor and blades in extraction condensing turbines are highly engineered components that are essential for efficient steam energy conversion. Their design, material selection, and ongoing maintenance ensure that the turbine can handle the varying steam conditions, high pressures, and temperatures, ultimately delivering power and heat with minimal losses. These components must also withstand mechanical stresses and environmental factors such as erosion and corrosion, which requires careful engineering and periodic maintenance. The rotor and blades, as key parts of the turbine system, are critical for optimizing the turbine’s performance, ensuring reliability, and maximizing energy efficiency.
Continuing with the turbine rotor and blades, another critical aspect is their role in the overall thermodynamic efficiency of the turbine. The way the steam interacts with the blades influences how effectively the turbine converts thermal energy into mechanical work. A well-designed rotor and blade system allows for maximum steam expansion and efficient energy extraction, which directly impacts the turbine’s overall performance.
As steam enters the turbine at high pressure and temperature, it expands and loses energy as it flows through the stages. The design of the rotor and blades in each stage is tailored to handle specific pressure and temperature levels of steam. In the initial stages of the turbine, where steam pressure is the highest, the blades are designed with larger surface areas and more robust construction to handle the intense forces. These stages are designed to capture a significant amount of energy from the steam by harnessing the steam’s pressure drop. As the steam progresses to subsequent stages, the blades become progressively smaller and more finely tuned to accommodate the lower-pressure steam.
The stage-by-stage design of the rotor and blades in an extraction condensing turbine ensures that each stage extracts the optimal amount of energy, without overloading the later stages. By maintaining this balance, the turbine can operate at peak efficiency, converting the maximum amount of thermal energy into mechanical energy at every stage. The precise control of steam velocity and blade angle at each stage allows the turbine to extract energy efficiently, regardless of the fluctuating steam conditions associated with energy extraction for industrial processes or heating.
The rotor itself is designed with precision to ensure minimal friction and wear. Friction can cause losses in energy conversion, as it reduces the mechanical efficiency of the rotor. To minimize these losses, the rotor is supported by bearings that allow it to rotate smoothly with minimal resistance. These bearings are typically made of materials that can withstand the high rotational speeds and temperatures of the turbine. Advanced lubrication systems are also employed to reduce friction and wear on the rotor, ensuring that the turbine operates at optimal efficiency for longer periods of time.
The rotor and blades also need to be highly durable to handle the stresses of continuous operation. In many cases, these components are exposed to extreme mechanical forces caused by the high rotational speeds of the rotor and the thermal stresses from the steam. Over time, these forces can cause fatigue in the rotor and blade materials, leading to cracks or other forms of mechanical failure. To prevent this, rotor and blade designs often incorporate materials and construction methods that help distribute these stresses more evenly. For example, the rotor may be constructed with multi-stage or layered designs to reduce the risk of fatigue, while the blades may be equipped with specialized coatings or reinforcements to ensure they can withstand the mechanical forces without cracking or breaking down.
In addition to mechanical stresses, the rotor and blades must also contend with thermal stresses that result from the constantly changing temperature of the steam. As steam enters the turbine, it typically has a high temperature, which causes the materials of the rotor and blades to expand. As the steam exits the turbine, the temperature drops, leading to contraction of the components. This continual expansion and contraction can lead to the formation of cracks or warping over time if not managed properly. To counteract these issues, turbine manufacturers use advanced thermal management techniques, such as heat-resistant coatings, cooling channels within the blades, and the careful design of thermal gradients. These measures ensure that the rotor and blades maintain their structural integrity and perform effectively throughout the turbine’s operational life.
Furthermore, extraction condensing turbines often operate under variable loads and conditions, meaning the steam conditions may change throughout the day or season. The rotor and blades need to be flexible enough to handle these variations in steam quality without compromising turbine efficiency. When steam is extracted at various points to meet the varying heating demands of industrial processes, the turbine experiences changes in the flow rate and temperature. The rotor and blades are designed to maintain stability and optimal efficiency even as these fluctuations occur, adapting to different operational conditions.
To ensure optimal performance and extend the life of the rotor and blades, regular maintenance is essential. Technicians perform detailed inspections to monitor for signs of wear, erosion, or thermal damage. Common issues that are monitored include cracks, surface pitting, and material fatigue. As part of routine maintenance, the blades may be coated with protective layers to prevent corrosion and oxidation, which can degrade their performance over time. Additionally, the rotor and blades may be replaced periodically depending on the extent of wear and tear. These maintenance practices ensure that the turbine operates smoothly and efficiently, minimizing downtime and maximizing the return on investment.
The rotor and blades also play a key role in ensuring that the turbine operates safely. Given the high pressures and temperatures involved in steam power generation, any failure in the rotor or blades could lead to catastrophic damage to the turbine or other connected systems. For this reason, turbines are designed with safety features such as pressure relief valves, emergency shut-off mechanisms, and real-time monitoring systems to detect any deviations from optimal operating conditions. These systems ensure that the turbine can be shut down quickly in the event of a malfunction, preventing further damage and protecting the safety of the operators and the equipment.
Finally, the advancements in digital technology have further enhanced the role of rotor and blade design. Modern turbines often include sensors and control systems that allow for real-time monitoring of performance parameters such as steam flow, pressure, and temperature. These systems provide data that can be used to make adjustments to the turbine operation, ensuring that the rotor and blades are always operating within optimal parameters. Predictive maintenance tools can also be employed to anticipate when the rotor and blades will need attention, further reducing the risk of unplanned outages and extending the life of the turbine.
In conclusion, the rotor and blades of a steam turbine are vital components that directly influence the turbine’s ability to efficiently convert steam energy into mechanical work. Their design must account for a range of operational factors, including steam pressure and temperature, mechanical stresses, thermal conditions, and maintenance needs. By carefully selecting materials, optimizing geometries, and employing advanced technologies, turbine manufacturers ensure that the rotor and blades can provide reliable, high-efficiency performance over long periods of operation. Through proper maintenance and ongoing advancements in design, these components continue to play a key role in the performance, durability, and efficiency of modern steam turbines.
The turbine rotor and blades continue to be the heart of a steam turbine’s operation, with their ability to effectively manage the interaction between steam and mechanical energy crucial to the overall system’s performance. As turbines evolve and technological advancements continue, further enhancements to rotor and blade design are helping to push the boundaries of efficiency and operational capacity. These improvements can be broadly categorized into areas such as material innovations, aerodynamic advancements, and the application of advanced computational techniques.
One area of significant progress has been the development of advanced materials for rotor and blade construction. In the past, turbine blades were predominantly made from stainless steel and alloys with relatively good resistance to heat and wear. However, today, more advanced materials such as superalloys (including those based on nickel, cobalt, and titanium) are commonly used in blade and rotor production. These superalloys are specifically designed to endure the extreme conditions within the turbine, with excellent heat resistance, oxidation resistance, and fatigue strength at high temperatures. Superalloys also possess the ability to maintain their strength and performance even under high-temperature steam conditions, making them ideal for the most demanding turbine applications.
In addition to improved material science, advancements in surface coatings have further improved rotor and blade longevity. High-performance coatings can be applied to turbine blades to provide protection against oxidation, corrosion, and wear from moisture droplets and abrasive particles in the steam. These coatings also act as thermal barriers, reducing the amount of heat transferred to the material beneath. The use of advanced coatings is particularly important for turbines operating in corrosive environments or those with high moisture content in the steam, as the blades are exposed to conditions that can accelerate degradation. This layer of protection significantly extends the life cycle of rotor and blade components, ensuring turbines remain efficient and reliable for longer periods.
Another major advancement in turbine rotor and blade design is the improved aerodynamic shaping of blades. Computational fluid dynamics (CFD) software has revolutionized the design process, enabling engineers to simulate and optimize steam flow over the blades in ways that were not possible in the past. Through CFD, blade geometry can be optimized for reduced drag, minimized turbulence, and enhanced energy extraction. The ability to predict how steam interacts with the blades at different stages allows for more efficient blade designs, which in turn can improve turbine efficiency. Modern turbine blades are often more finely tuned in terms of angle, curvature, and length, taking into account the variations in steam conditions they will face throughout the turbine’s operating range. By reducing energy losses caused by inefficient steam flow, optimized aerodynamic blade designs improve overall turbine performance.
Furthermore, blade designs now often incorporate more sophisticated cooling techniques to mitigate the extreme thermal stresses they encounter. Cooling techniques are employed not only within the blade material but also through the use of advanced internal cooling channels, which circulate coolant (such as air or water) through the blades to lower their operating temperature. This cooling technology is vital in high-efficiency turbines where steam temperatures can be incredibly high, potentially leading to thermal fatigue if not properly managed. By keeping the blades at an optimal temperature, the turbine can operate more efficiently and reduce the risk of damage over time. Some turbines also employ film cooling, where a thin layer of coolant is applied to the surface of the blades to create a protective barrier against the hot steam, further enhancing the lifespan and performance of the blades.
Another area of focus for turbine rotor and blade enhancement is the incorporation of more precise sensors and monitoring technologies. These sensors can track the operating conditions of the blades in real-time, measuring parameters such as temperature, pressure, vibration, and stress. This data is crucial for predictive maintenance, allowing operators to identify any irregularities or potential issues before they lead to system failure. For example, vibrations that exceed safe limits may indicate that a blade is experiencing excessive wear or fatigue, enabling early intervention to prevent further damage. The integration of monitoring systems into turbine rotors and blades adds another layer of operational reliability and ensures that the turbine can be operated safely and efficiently over the long term.
Moreover, the efficiency of the rotor and blades can be further enhanced through the use of more advanced, automated control systems that regulate the steam flow and pressure across different stages of the turbine. By actively controlling the flow of steam to different blade stages, the turbine can ensure that it always operates within its optimal performance parameters, regardless of fluctuating energy demand or steam supply conditions. These systems adjust the steam flow in real-time, optimizing the performance of the blades by maintaining the correct steam pressure and temperature across each stage. This level of dynamic control helps minimize losses and maintains the highest possible efficiency throughout the turbine’s operation.
Maintenance and repair practices are evolving as well. In the past, when rotor or blade damage occurred, the turbine would often need to be taken offline for extended periods of time while repairs were made. However, with the rise of advanced materials, sensor technology, and predictive analytics, maintenance is becoming more proactive rather than reactive. Components can now be inspected remotely using advanced diagnostic tools such as ultrasonic testing, infrared imaging, and eddy current testing. These technologies allow for the detection of microscopic cracks or material fatigue that may not be visible through traditional inspection methods, ensuring that any issues are identified before they cause significant damage. In many cases, damaged blades or rotors can be repaired on-site with minimal disruption to turbine operation, reducing downtime and maintenance costs.
The role of the rotor and blades in an extraction condensing turbine is also influenced by the changing nature of steam cycles and energy demands. In modern turbines, where the production of both power and heat is required, the rotor and blades must handle varying amounts of extracted steam and maintain efficiency over a wide operating range. As industrial processes or heating demands fluctuate, steam flow rates and temperatures can change significantly. The rotor and blades must be adaptable to these shifts in order to optimize energy extraction while minimizing losses. This adaptability is increasingly supported by sophisticated control systems, which allow the turbine to dynamically adjust to varying operating conditions without sacrificing efficiency.
In conclusion, the rotor and blades of extraction condensing turbines are constantly evolving as advancements in materials, aerodynamics, and control systems continue to improve turbine performance. These components are designed to extract maximum energy from steam while withstanding extreme thermal and mechanical stresses. The adoption of new technologies such as superalloys, advanced surface coatings, optimized blade geometries, and real-time monitoring systems ensures that turbines operate at peak efficiency and reliability. As energy demands grow and environmental considerations become more critical, the continued innovation in rotor and blade design will play a pivotal role in achieving higher efficiency and more sustainable energy generation.
Extraction System
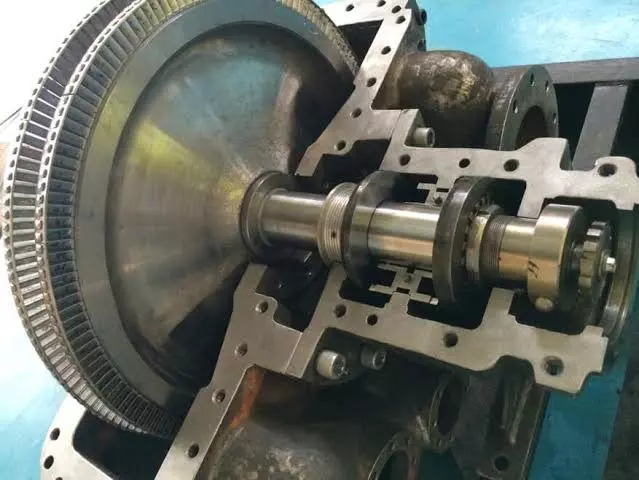
The extraction system in a steam turbine is a critical component designed to capture steam at various pressure points throughout the turbine and direct it to external processes, such as heating systems, industrial applications, or district energy networks. This functionality allows the steam turbine to serve multiple purposes, not just power generation, but also the simultaneous production of heat or other forms of energy. The design of the extraction system and its integration into the turbine must ensure that the steam is extracted efficiently while maintaining optimal turbine performance and meeting the needs of the external systems.
In a typical extraction system, steam is drawn off from the turbine at specific pressure levels through extraction points or ports, each strategically placed within the turbine’s stages. These points are often located between the expansion stages where steam pressure decreases, allowing for the controlled extraction of steam at varying pressures and temperatures. The extracted steam is then routed through a series of pipes or ducts to the intended external process, such as a heating system, industrial process, or even back to the turbine for further expansion in a reheating process.
The design of the extraction system must be able to balance multiple factors. For one, the extraction of steam should not significantly affect the turbine’s overall performance. The extraction points must be carefully placed to avoid disrupting the pressure and flow of steam through the turbine stages, ensuring that the turbine continues to operate at optimal efficiency. This is typically achieved by using advanced flow management techniques that regulate steam extraction based on real-time conditions, allowing for precise control over the extraction process.
The efficiency of the extraction system is influenced by how effectively the steam is diverted without causing pressure drops that would reduce the turbine’s overall power output. In the case of extraction condensing turbines, where steam is extracted for use in heating or industrial processes, the extracted steam must maintain the desired temperature and pressure levels to serve its intended function. If the steam is too cool or its pressure too low, it may not meet the requirements of the external system, which could lead to inefficiency and a reduction in the overall effectiveness of the cogeneration system.
To maintain the turbine’s efficiency and reliability, the extraction system must be carefully controlled and monitored. Many modern systems are equipped with advanced sensors and control systems that allow operators to monitor steam conditions and adjust extraction rates as necessary. These systems can automatically adjust extraction volumes to maintain the necessary pressure levels in the turbine stages, ensuring that the turbine continues to generate power efficiently while supplying steam to external processes. Automation plays a crucial role in optimizing the operation of the extraction system, as it can adjust the extraction process in response to fluctuating external demands or changes in operational conditions.
One of the key challenges in designing the extraction system is managing the pressure drop that occurs when steam is extracted from the turbine. Extracting steam at different stages means that the remaining steam in the turbine must go through additional stages of expansion to reach the desired exhaust pressure, which can impact the overall energy efficiency of the turbine. The extraction system must, therefore, be designed to minimize these losses by ensuring that steam extraction is balanced and does not result in excessive pressure drops that would reduce the turbine’s output.
Additionally, the extraction system must account for varying demand in the external processes. For example, heating systems may require different amounts of steam depending on the time of day, weather conditions, or operational needs. The ability of the extraction system to adjust the volume of steam being extracted based on these dynamic needs is a key characteristic of modern cogeneration systems. Some turbines are equipped with adjustable extraction valves that allow for more precise control over the amount of steam being extracted, while others use variable speed drive mechanisms to manage the flow of steam at different pressure points.
In terms of the physical components, the extraction system includes extraction valves, piping, and sometimes heat exchangers or pressure regulators to ensure that the extracted steam is appropriately conditioned before being used. The extraction valves control the flow of steam from the turbine to the external system, adjusting in response to signals from the control system to either increase or decrease the flow based on demand. The piping network must be designed to minimize energy losses, maintain pressure integrity, and ensure that steam reaches the external process in the required state. In some cases, the extracted steam is passed through heat exchangers to adjust its temperature or pressure before use, depending on the needs of the connected system.
One of the considerations in extraction system design is the potential for moisture or contaminants in the extracted steam. In a steam turbine, moisture can form as steam expands through the turbine stages, particularly in the later stages where the pressure is low and condensation may occur. If extracted steam contains moisture, it may reduce the efficiency of the external system it is feeding into, especially if it is being used for heating purposes where dry steam is required. The extraction system must, therefore, be designed to minimize the moisture content in the extracted steam. This is typically achieved by placing extraction points strategically in the turbine stages, where the steam is still superheated and in vapor form, before condensation occurs.
Regular maintenance of the extraction system is crucial to ensure that the turbine operates efficiently and that the extracted steam remains in the desired condition. Over time, components such as extraction valves, piping, and heat exchangers can suffer from wear and corrosion, particularly in high-pressure or high-temperature environments. Regular inspections and maintenance ensure that these components continue to operate at peak efficiency. Additionally, any changes in the external system, such as fluctuations in heating demand or changes in the operational parameters of the industrial process, may require adjustments to the extraction system to maintain optimal performance.
The interaction between the extraction system and the turbine’s other components, such as the condenser and the generator, also plays a crucial role in maintaining overall system efficiency. The balance between the steam that is extracted for external use and the steam that continues through the turbine for power generation must be carefully managed. This balance ensures that the turbine operates at maximum capacity while also meeting the heating or industrial steam needs of the external systems. Mismanagement of this balance can result in suboptimal turbine performance, reducing efficiency and increasing operational costs.
In conclusion, the extraction system of a steam turbine is essential for its role in cogeneration, ensuring that steam can be extracted at various points to meet the needs of external systems while maintaining the turbine’s overall performance. The design and operation of the extraction system require careful consideration of steam flow, pressure, and temperature conditions, along with the use of advanced control and monitoring systems to optimize the extraction process. By maintaining a balance between power generation and external steam demands, the extraction system contributes significantly to the overall efficiency and flexibility of the turbine, enabling it to serve as a key component in combined heat and power (CHP) systems or other industrial applications.
Continuing with the extraction system, it’s essential to highlight how the integration of the extraction process within the larger steam turbine system can vary based on operational needs and design preferences. The extraction system’s flexibility allows the turbine to be used in various scenarios, from baseload power generation to situations where more dynamic adjustments are needed for industrial processes or district heating.
One of the most crucial aspects of the extraction system is its ability to handle different extraction pressures. The extraction points, or extraction valves, must be able to operate across a range of pressures, ensuring that the steam is delivered at the right temperature and pressure for its intended use. The pressure at which steam is extracted from the turbine directly influences both its energy content and its suitability for different applications. For example, steam extracted at a lower pressure might be ideal for district heating, where lower temperatures are required. In contrast, steam extracted at higher pressures could be more appropriate for industrial processes that need high-temperature steam.
The pressure regulation of the extracted steam is managed through carefully engineered control systems, which adjust the extraction process according to demand. These systems can be automated to respond to changes in steam demand in real time, such as those that occur during peak heating times or in industrial systems that fluctuate throughout the day. The ability to modulate steam extraction in response to these demands helps optimize the overall efficiency of the turbine, ensuring that it produces the maximum possible amount of power while still meeting the external steam requirements.
The design of the extraction system also considers the possible impact of steam extraction on the turbine’s exhaust conditions. In systems where high-pressure steam is extracted at multiple points, the steam that exits the turbine can have a lower energy content by the time it reaches the exhaust stage. This needs to be factored into the design, as it can affect the efficiency of the overall turbine system. In some cases, additional stages such as reheating might be necessary to recover some of the lost thermal energy before it exits the turbine. Reheating involves passing the extracted steam back through a heater before returning it to the turbine for further expansion, which helps maximize the turbine’s overall thermal efficiency.
When discussing extraction systems, another factor to consider is the longevity and reliability of the components that make up the system. Over time, components such as valves, piping, and seals may experience wear and tear due to the high pressure and temperature conditions they are subjected to. This makes regular maintenance and monitoring an essential part of the turbine’s operation. Advanced monitoring technologies, such as vibration sensors, pressure sensors, and real-time thermographic systems, allow operators to detect potential issues before they lead to costly failures. Preventive maintenance protocols, informed by data from these monitoring systems, help extend the lifespan of the extraction system and ensure that the turbine operates at optimal efficiency throughout its service life.
One potential challenge in extraction systems is the presence of non-condensable gases in the extracted steam, which can affect the quality of the steam and the efficiency of the external processes it serves. These gases, which may be present due to leaks or other inefficiencies within the turbine, can accumulate and create performance issues, including pressure imbalances or reduced heat transfer efficiency in heating applications. To mitigate this issue, extraction systems often incorporate air extraction or venting systems that remove non-condensable gases before the steam is sent to external applications. This ensures that the extracted steam retains its desired properties, minimizing the risk of inefficiency or damage to downstream equipment.
In terms of economic considerations, the extraction system plays a significant role in the cost-effectiveness of combined heat and power (CHP) systems. By extracting steam for external use, turbines can improve the overall economics of the plant, as they can provide both electricity and thermal energy. The steam extraction process is particularly beneficial in industrial settings, where heat is often required for processes like chemical manufacturing, food production, or even water desalination. Instead of generating separate heat sources, the turbine can provide both power and steam, reducing fuel consumption and lowering operational costs.
As energy efficiency and environmental sustainability become increasingly important, the extraction system’s design can also be tailored to reduce emissions and make the most of available resources. Efficient extraction systems help reduce the overall fuel consumption of a plant by ensuring that steam is used as efficiently as possible. This not only decreases operating costs but also helps lower greenhouse gas emissions, especially when the extracted steam replaces the need for additional fossil fuel-based heating. Furthermore, cogeneration systems that employ steam extraction help improve the overall efficiency of the plant, as the energy that would otherwise be wasted in a purely electrical generation process is repurposed for heating or industrial use.
In conclusion, the extraction system in a steam turbine is a multifaceted component that directly influences both the efficiency and flexibility of the turbine. By strategically extracting steam at various pressures and temperatures, the extraction system allows the turbine to meet a wide range of external demands while still maintaining power generation capabilities. Through advanced control systems, material design, and regular maintenance, the extraction system can be optimized to ensure that both the steam turbine and the connected external processes operate at maximum efficiency. As part of a cogeneration or combined heat and power system, the extraction system helps reduce fuel consumption, lower operational costs, and contribute to more sustainable energy production, making it a key feature in modern turbine-based energy solutions.
Continuing with the extraction system, it’s important to discuss how evolving technologies and design innovations are shaping the way extraction systems operate in modern steam turbines. The ongoing pursuit of higher energy efficiency, cost-effectiveness, and reduced environmental impact has led to the development of more advanced features and design strategies within the extraction system.
One area where innovation is making a significant impact is in the integration of advanced sensors and real-time data analytics. In the past, extraction systems relied primarily on manual inspection and fixed control points to manage steam flow and pressure. However, today’s turbines often employ an array of sensors embedded in the system to monitor key parameters such as steam temperature, pressure, flow rates, and even moisture content. These sensors feed data into sophisticated control systems that can adjust extraction parameters dynamically, ensuring that the turbine operates at peak efficiency in real-time.
This shift to more intelligent systems has improved the responsiveness and adaptability of extraction systems. For example, during periods of fluctuating energy demand or changes in external heating requirements, the system can automatically modulate steam extraction to meet those changes. These systems can fine-tune extraction amounts across different stages of the turbine, allowing operators to extract steam precisely as needed without overburdening the turbine or losing power generation capacity.
Furthermore, the ability to monitor steam conditions also facilitates more precise control over the quality of extracted steam. In applications such as district heating, where consistent temperature and pressure are crucial, the ability to maintain optimal steam quality is vital. If the extracted steam has too much moisture or is at an incorrect pressure, it can result in inefficiencies or damage to the systems that rely on it. By using real-time data to adjust steam extraction as needed, modern systems can help ensure that steam maintains its intended quality, minimizing potential problems and increasing system reliability.
In addition to sensor-driven improvements, the use of digital twins and predictive maintenance models is becoming more common in advanced extraction systems. A digital twin is a virtual replica of the physical turbine and its associated components, including the extraction system. This digital model is continuously updated with real-time data from the turbine, enabling operators to simulate various operational scenarios and assess the impact of different parameters on the system’s performance.
Predictive maintenance powered by digital twin technology allows operators to anticipate potential issues before they arise, reducing the risk of costly downtime or system failures. By analyzing patterns in the data, predictive models can identify wear and tear on components, such as valves or extraction pipes, and suggest the best time for maintenance or replacement. This proactive approach to maintenance helps extend the life of the extraction system and ensures that it continues to operate efficiently over time.
Another key innovation in the extraction system design is the incorporation of multi-stage extraction points, which provide even greater flexibility in how steam is diverted from the turbine. Multi-stage extraction allows the system to extract steam at different pressures at various points throughout the turbine, improving the overall thermal efficiency of the plant. By utilizing several extraction points, the turbine can operate in a more adaptable and responsive manner, catering to multiple external systems that may have varying steam requirements.
For example, a plant may need steam at one pressure for district heating and another pressure for an industrial process. A multi-stage extraction system can efficiently provide both of these streams of steam without sacrificing the turbine’s power generation capacity. The ability to extract steam at multiple pressures also allows for more optimized steam expansion within the turbine, minimizing losses and improving the overall efficiency of the entire cycle.
The design of the piping network and valves used in the extraction system has also evolved to enhance the system’s overall performance. Modern systems use advanced materials for the piping, such as corrosion-resistant alloys or composites, to ensure that the extraction system can withstand the high-pressure and high-temperature conditions it operates under. These materials help prevent leaks, ensure the system’s durability, and extend the life of components exposed to the steam. Additionally, the design of valves has improved to allow for smoother flow control and quicker response times, ensuring that steam is extracted in a controlled and efficient manner.
Moreover, the incorporation of heat recovery systems within the extraction process is another crucial factor in improving overall system efficiency. Heat recovery steam generators (HRSG) can capture waste heat from the exhaust or the extracted steam itself and reuse it to preheat the incoming feedwater. This helps reduce the amount of fuel needed to generate the same amount of power, increasing the overall thermal efficiency of the system. In combined heat and power (CHP) systems, this heat recovery can be directed back into the district heating network or to other industrial processes that require thermal energy.
For example, HRSGs can recover heat from the exhaust gases of a gas turbine and use that heat to generate steam, which can then be used for additional extraction points in a steam turbine. By utilizing multiple sources of energy within a plant, including both mechanical and thermal, extraction systems can be an integral part of creating more sustainable and cost-effective operations. The integration of heat recovery not only helps save on operational costs but also reduces the environmental footprint of the plant by utilizing existing energy more efficiently.
When examining the economic impacts of modern extraction systems, one key advantage is the potential for improved fuel efficiency and lower operational costs. By efficiently extracting steam for external use, the turbine can reduce the need for separate heating sources, such as boilers or dedicated heat plants, that would otherwise burn additional fuel. The extraction system thus plays a significant role in lowering the plant’s overall fuel consumption and improving its cost-effectiveness. The added flexibility also means that turbines can be used in a variety of energy markets, responding dynamically to changes in demand and maximizing profit potential.
The economic benefits also extend to the operating costs of the turbine itself. By improving the overall efficiency of steam extraction, the turbine can generate more usable energy with less fuel, reducing the cost per unit of energy produced. This can have a direct impact on the profitability of the plant, especially in competitive energy markets where cost efficiency is paramount. Additionally, the use of real-time monitoring, predictive maintenance, and digital twin technology can reduce maintenance costs by extending the life of components and minimizing unscheduled downtime, which are key contributors to operational expenses.
Sustainability is another critical consideration in modern extraction systems. As the global energy landscape increasingly shifts toward cleaner and more sustainable sources of energy, the role of cogeneration systems with extraction turbines has become more prominent. By utilizing both electricity and heat from the same fuel source, these systems help optimize the use of energy and reduce overall carbon emissions. Steam turbines equipped with advanced extraction systems can significantly lower a plant’s environmental impact by reducing the need for additional energy generation from fossil fuels. Furthermore, by supplying heat to external applications such as district heating or industrial processes, extraction systems contribute to decarbonizing sectors that rely heavily on thermal energy.
In conclusion, the extraction system in a steam turbine is a vital component that enables turbines to operate in a flexible, efficient, and cost-effective manner. The continued evolution of extraction system technologies, such as advanced sensor integration, multi-stage extraction, predictive maintenance, and heat recovery, allows for increasingly efficient operation, better performance, and lower operating costs. These advancements not only help maximize the performance of turbines but also contribute to sustainability and economic benefits in modern energy systems. As industries and power plants look to optimize their energy use, the extraction system will remain a central feature of many combined heat and power applications, providing both electrical and thermal energy in an efficient and sustainable way.
Continuing with the extraction system’s role and the advancements shaping its future, it is essential to further explore the challenges and considerations that arise in the design, operation, and maintenance of modern steam turbine extraction systems. These challenges are shaped by the increasing complexity of modern power plants and the evolving demands for greater efficiency, reliability, and environmental sustainability.
One of the significant challenges in managing the extraction system is maintaining the balance between power generation and external steam demand. Steam extraction must be carefully calibrated to ensure that the turbine continues to operate at optimal efficiency while meeting the needs of external systems. If too much steam is extracted, it can lead to a reduction in the turbine’s power generation capability. Conversely, insufficient steam extraction may result in external systems not receiving the required thermal energy. This delicate balancing act requires advanced control mechanisms, such as automated pressure regulation and real-time steam demand monitoring, to ensure that the extraction system adapts dynamically to changing conditions.
For instance, in a district heating application, the heat demand may vary throughout the day due to changes in ambient temperature or consumer demand patterns. Similarly, industrial processes that rely on steam may require varying amounts of thermal energy depending on production schedules. The extraction system must be able to adjust the steam extraction levels based on these fluctuating demands without compromising the turbine’s efficiency. Advanced digital control systems, integrated with real-time data from external processes, allow for precise regulation of extraction points and the efficient allocation of steam, ensuring that both power generation and thermal energy needs are met.
Another challenge is managing steam quality. Steam that is too wet or has a high moisture content can be detrimental to the extraction system and the external processes it serves. Wet steam can damage equipment, such as heat exchangers or industrial machinery, leading to increased maintenance costs and downtime. To prevent this, the extraction system must be designed to avoid steam condensation before it is extracted, ensuring that the steam remains dry and at the desired temperature and pressure. In some cases, separators or moisture-removal systems are incorporated into the extraction system to ensure the extracted steam meets the required quality standards.
A related concern is the potential for pressure fluctuations in the extraction system, which can occur due to variations in the steam flow rate or external demand. These fluctuations can affect the efficiency of the turbine and result in increased wear on components such as valves, pipes, and seals. To address this, many modern extraction systems include pressure regulation mechanisms, such as control valves or pressure relief valves, that help stabilize the pressure in the system and minimize the impact of pressure fluctuations. These mechanisms are crucial for maintaining the integrity of the extraction system and ensuring that steam is delivered at the appropriate pressure and temperature.
Operational flexibility, which is one of the strengths of modern extraction systems, can also be a double-edged sword. While the ability to extract steam at multiple pressures and in varying amounts provides greater flexibility in meeting the needs of external systems, it also introduces additional complexity in terms of system design and operation. The turbine must be able to handle the varying steam flow rates and pressures without compromising its overall performance. To manage this complexity, many advanced turbines incorporate features such as variable-speed drives, which adjust the turbine’s rotational speed to match the steam flow, and variable-valve mechanisms, which control the amount of steam extracted at each point.
These flexible systems also need to be carefully managed to avoid overloading the turbine or extraction system. For example, if extraction levels are increased too quickly or too much steam is diverted, it can lead to issues such as low pressure in the turbine stages, reduced power generation, or overheating of critical components. Operators must carefully monitor steam conditions, both within the turbine and in the external systems, to ensure that steam extraction remains within safe and efficient operating limits.
Maintenance is another critical area that demands attention in the context of extraction systems. With increased operational complexity and the use of advanced technologies, the maintenance of extraction systems has become more specialized and data-driven. Predictive maintenance strategies, powered by real-time data and advanced analytics, are helping operators identify potential failures before they occur. By continuously monitoring parameters such as vibration, temperature, and pressure, operators can detect anomalies that could signal the need for maintenance or repairs.
For example, a change in vibration patterns in an extraction valve or an unusual temperature rise in the piping system could indicate wear or damage, allowing operators to address the issue before it leads to a failure. In addition to minimizing downtime, predictive maintenance helps reduce maintenance costs by optimizing the scheduling of repairs and parts replacement based on actual wear patterns, rather than relying on fixed maintenance intervals. This approach maximizes the lifespan of components and ensures that the turbine’s extraction system remains in peak operating condition.
Modern extraction systems also benefit from improved materials and construction techniques. The materials used in the construction of valves, piping, and other components must be able to withstand high temperatures, high pressures, and exposure to steam, all of which can cause degradation over time. Advances in metallurgy and materials science have led to the development of corrosion-resistant alloys, high-performance seals, and advanced coatings that improve the durability and longevity of extraction system components. These innovations help extend the life of the system and reduce the frequency of costly repairs or replacements.
As energy efficiency standards become more stringent and sustainability concerns grow, the role of the extraction system in reducing environmental impact is becoming increasingly important. Extraction systems are a key part of combined heat and power (CHP) systems, which are considered more efficient than separate electricity and heat generation because they maximize the use of energy that would otherwise be wasted. By utilizing waste heat for heating or industrial processes, CHP systems help reduce greenhouse gas emissions and the consumption of fossil fuels, contributing to more sustainable energy production.
For instance, in a district heating system, the extracted steam can be used to heat buildings or provide hot water, displacing the need for separate heating sources, such as boilers or electric heaters. Similarly, in industrial processes, steam extracted from the turbine can replace other fuel-intensive heating methods, helping reduce the overall carbon footprint of the operation. In this way, the extraction system not only improves energy efficiency within the turbine but also contributes to broader environmental sustainability goals.
Looking forward, the continued development of digital technologies, artificial intelligence, and machine learning will likely enhance the capabilities of extraction systems even further. These technologies can improve the accuracy and responsiveness of control systems, allowing for more refined adjustments in real-time. Artificial intelligence, for example, could predict changes in steam demand based on external factors such as weather, economic activity, or production schedules, and adjust extraction parameters accordingly. As these technologies continue to evolve, they will allow extraction systems to operate more efficiently, with greater precision, and at lower cost, further contributing to the optimization of steam turbine performance.
In conclusion, the extraction system is a fundamental component of steam turbine operations, playing a crucial role in maintaining efficiency, flexibility, and cost-effectiveness. The advancements in sensor technology, predictive maintenance, material science, and digital control systems are reshaping how extraction systems are designed, operated, and maintained. While challenges remain in managing steam quality, pressure fluctuations, and system complexity, the continued development of advanced systems and technologies is helping to address these issues. The future of extraction systems will likely be marked by even greater integration of automation, artificial intelligence, and sustainability principles, making them an increasingly efficient and vital part of the global energy landscape.
Condenser
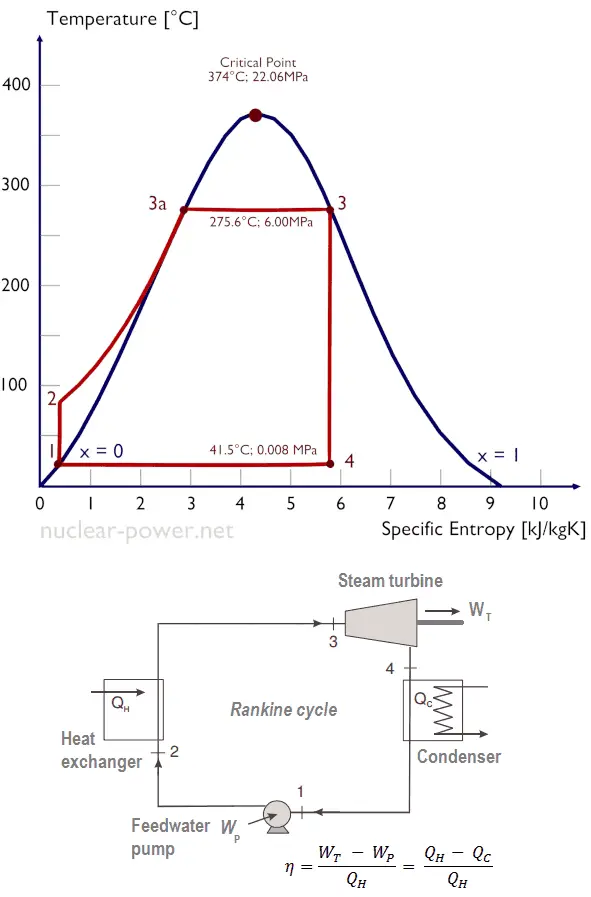
The condenser is a critical component in many thermodynamic cycles, particularly in steam turbines used in power plants. Its primary role is to cool and condense the exhaust steam from the turbine into liquid water, allowing the cycle to continue and maximizing efficiency. The design and operation of a condenser are essential for optimizing energy conversion in a steam turbine system, as it directly influences both the thermal efficiency and the overall performance of the power plant.
At the core of a steam turbine system, the condenser receives steam at low pressure from the turbine’s exhaust. This steam, which is typically at high temperature, needs to be cooled before it can be pumped back into the boiler to complete the water-steam cycle. The condenser achieves this by transferring heat from the steam to a cooling medium, typically water, that flows through tubes or other surfaces within the condenser. As the steam loses heat, it undergoes a phase change, transitioning from a vapor to a liquid state, which is essential for the efficiency of the system.
Condensers generally operate under vacuum conditions, meaning that the pressure inside the condenser is kept lower than the atmospheric pressure. This low pressure helps facilitate the condensation process by creating a pressure differential between the turbine exhaust and the condenser, allowing the steam to condense more effectively. The lower pressure in the condenser also contributes to the production of a more efficient vacuum that helps extract more energy from the steam as it passes through the turbine.
The process of condensation in the condenser is heavily influenced by the temperature of the cooling medium, which typically comes from a nearby water source, such as a river, lake, or cooling tower. This cooling medium circulates through a series of heat exchanger tubes, where it absorbs the heat from the exhaust steam. The cooled steam then condenses on the outside of these tubes, converting into liquid water, which is then collected at the bottom of the condenser. The resulting condensate is then pumped back into the boiler to be reheated and reused in the steam cycle.
In order to optimize the heat transfer process, condensers are designed with highly efficient heat exchange surfaces. These surfaces can take various forms, such as horizontal or vertical tubes, plates, or other specialized materials designed to maximize surface area and facilitate heat exchange. The larger the surface area for heat exchange, the more effectively the condenser can cool the steam and condense it. This is why many condensers are designed with intricate networks of tubes or finned surfaces to maximize heat transfer and improve system performance.
One of the primary performance metrics for a condenser is its heat transfer rate, which is determined by the rate at which heat is extracted from the steam. A higher heat transfer rate typically leads to better cooling and higher condenser efficiency, which in turn contributes to the overall thermal efficiency of the steam turbine cycle. In many cases, condensers are designed to operate at specific temperature differentials between the steam and the cooling medium to optimize this heat transfer process. This means that the selection of the cooling medium and its temperature are important factors in determining the condenser’s performance.
In addition to maximizing heat transfer efficiency, condensers must also handle the challenges of scaling, fouling, and corrosion. These issues arise from the presence of impurities in the water supply, which can lead to deposits forming on heat transfer surfaces, reducing their effectiveness. Over time, these deposits can accumulate and cause significant reductions in the heat transfer rate. To prevent these issues, condensers are designed with regular cleaning and maintenance schedules to remove fouling and ensure that the system operates at optimal efficiency.
In some cases, condensers may use advanced materials or coatings that resist corrosion or fouling, such as alloys or non-stick coatings, to improve longevity and performance. In addition, water treatment systems may be employed to remove contaminants from the cooling water before it enters the condenser, further reducing the risk of fouling and corrosion.
The condenser also plays a critical role in maintaining the efficiency of the turbine. The pressure at the condenser’s exhaust side directly influences the performance of the turbine. By keeping the pressure low, the turbine can operate with a greater pressure differential between the inlet and exhaust sides, leading to higher efficiency in terms of power output. If the condenser is not functioning properly and the pressure inside it rises, it can reduce the efficiency of the turbine by limiting the pressure differential and increasing the backpressure on the turbine’s exhaust.
In addition to the role of the condenser in steam turbine performance, its design also affects the overall environmental impact of the power plant. In particular, the water used as a cooling medium in the condenser may have a significant environmental footprint if not properly managed. Power plants that rely on once-through cooling, where water is drawn from a nearby source and then discharged back into the environment after passing through the condenser, must be careful to avoid thermal pollution. The discharged water is often warmer than the source water, which can disrupt local ecosystems and negatively affect aquatic life. To mitigate this, many power plants utilize cooling towers, which allow the cooling medium to be reused in a closed-loop system, reducing thermal pollution.
The environmental considerations also extend to the use of water treatment chemicals and the handling of wastewater. Some condensers may produce large amounts of wastewater or require chemicals to prevent scaling, which must be carefully managed to avoid contamination of local water sources. Regulations surrounding water quality and discharge limits are becoming stricter in many parts of the world, so power plants must implement best practices and technologies to comply with these regulations and minimize their environmental impact.
As the demand for cleaner energy production grows, some modern power plants are exploring alternative cooling methods to further reduce their environmental footprint. Air-cooled condensers, for example, use ambient air instead of water to cool the exhaust steam, eliminating the need for a large water supply and reducing the environmental impact on local water resources. While air-cooled condensers are less efficient in terms of heat transfer compared to water-cooled systems, they are increasingly being used in regions where water availability is limited or where environmental concerns are a priority.
The development of new condenser technologies, such as hybrid systems that combine water and air cooling, offers an opportunity for power plants to balance efficiency with environmental sustainability. By integrating multiple cooling methods, these systems can reduce water consumption while maintaining high performance, making them ideal for areas facing water scarcity or other environmental challenges.
In conclusion, the condenser is an essential component in steam turbine systems, playing a key role in improving energy efficiency by cooling and condensing exhaust steam. Its ability to maintain low pressure and facilitate effective heat transfer directly impacts the performance of the turbine and the overall thermal efficiency of the plant. However, condensers must also be designed to handle challenges such as fouling, corrosion, and scaling, which can hinder their performance. As energy efficiency standards increase and environmental regulations tighten, condensers are evolving through the adoption of advanced materials, water treatment technologies, and alternative cooling methods. These innovations help optimize the performance of steam turbines, reduce environmental impact, and ensure the long-term sustainability of power plants worldwide.
The condenser’s design and operation are continually evolving to meet the increasing demands for both efficiency and environmental sustainability. As power plants are pushed to achieve higher levels of performance, innovation in condenser technology is key to improving overall system reliability, energy savings, and the reduction of emissions.
One of the latest trends in condenser development is the focus on reducing energy consumption in the cooling process. Traditional water-cooled condensers, while effective in transferring heat, rely heavily on large volumes of water, which can lead to inefficiencies in regions with limited water availability. To address this, some power plants are shifting towards closed-loop cooling systems, which recycle cooling water instead of discharging it into the environment. These systems reduce the need for fresh water while also improving heat transfer efficiency. For example, cooling towers that allow water to evaporate, thus expelling heat, can be more sustainable than once-through cooling systems. These innovations can significantly enhance water conservation, especially in areas facing water stress or drought conditions.
On the other hand, air-cooled condensers, which use ambient air to cool the steam, are gaining traction as an alternative cooling solution, particularly in arid climates or regions with limited access to water. Though air-cooled condensers tend to be less efficient than water-cooled counterparts in terms of heat transfer, advances in heat exchanger technology and fan efficiency are enabling these systems to perform better than in the past. By reducing or even eliminating the need for a constant water supply, air-cooled condensers offer a more environmentally friendly solution that can help reduce water consumption and its impact on local ecosystems. However, these systems still present challenges in terms of efficiency, especially in high-temperature climates where the temperature of the air is closer to that of the steam, limiting the condenser’s ability to efficiently cool the steam.
Furthermore, hybrid cooling systems are emerging as a promising solution to balance the benefits of both water and air cooling. These systems use a combination of water and air to cool the steam, ensuring more efficient heat transfer while also minimizing water usage. By switching between water and air cooling depending on local conditions, such as temperature and water availability, hybrid systems offer a flexible, adaptive approach that can provide the best of both worlds. For instance, during times of high heat or water scarcity, the system can rely more heavily on air cooling, and when cooling water is more abundant, it can utilize water-based cooling more efficiently. These hybrid systems can help reduce the overall environmental footprint of power plants and allow for more sustainable and reliable energy production.
Another important trend in condenser technology is the integration of digital monitoring and predictive analytics. Modern condensers are increasingly equipped with sensors and monitoring equipment that allow operators to track key performance indicators such as temperature, pressure, flow rate, and cooling efficiency in real-time. Advanced data analytics can be used to predict potential failures or performance degradation, enabling operators to perform maintenance proactively. Predictive maintenance allows power plants to avoid unexpected downtime, which can result in significant losses in energy production. By identifying issues such as fouling or scaling before they become serious problems, plants can ensure that the condenser operates at peak efficiency and avoid costly repairs.
Additionally, some modern condensers are incorporating adaptive control systems that optimize the cooling process based on real-time data. These systems can adjust parameters such as flow rates or fan speeds in response to changes in ambient conditions or cooling demands, ensuring that the condenser is always operating efficiently and effectively. By utilizing artificial intelligence and machine learning algorithms, these adaptive control systems can also improve decision-making and enhance the performance of the entire steam turbine system.
Materials science continues to play a critical role in improving condenser performance. New materials that resist corrosion, scaling, and fouling are helping to extend the lifespan of condenser components and improve the efficiency of heat transfer. For example, the use of corrosion-resistant alloys or non-stick coatings in heat exchanger tubes can help reduce the accumulation of mineral deposits, which can impair heat transfer and increase maintenance costs. These materials also reduce the need for frequent cleaning or replacement of condenser parts, leading to cost savings and improved system uptime.
As the global energy sector strives for greater sustainability, there is an increasing emphasis on reducing the environmental footprint of power plants, and condensers are a crucial element in this goal. Beyond reducing water usage and minimizing thermal pollution, condensers are also being integrated into systems that promote energy efficiency. For example, in combined heat and power (CHP) systems, the heat recovered from the condenser can be utilized for district heating or industrial processes, providing thermal energy in addition to electricity. This dual-purpose approach helps to maximize the use of energy that would otherwise be wasted and contributes to overall energy savings.
The future of condenser technology also points toward more advanced methods of managing heat recovery. In some cases, condensers are being paired with organic Rankine cycle (ORC) systems or other waste heat recovery technologies that capture residual heat from the steam and convert it into additional electricity. This combination of technologies can increase the overall energy output of the power plant while reducing the environmental impact by utilizing more of the thermal energy produced during the steam generation process.
Finally, there is a growing interest in improving the overall efficiency of the thermodynamic cycle in which the condenser operates. Enhancements to the steam cycle, such as improvements to turbine efficiency, better heat recovery systems, and integrated energy storage solutions, can all help optimize the role of the condenser. The synergy between these technologies, along with advancements in condenser design, will likely lead to the development of more efficient, reliable, and environmentally friendly steam turbine systems in the future.
In conclusion, the condenser is a vital component in steam turbine systems, facilitating the condensation of exhaust steam and contributing to the efficiency and sustainability of power plants. While traditional water-cooled condensers remain widely used, newer approaches such as air-cooled, hybrid, and digitalized condensers are addressing the challenges of water conservation, energy efficiency, and environmental impact. The continued development of materials, predictive maintenance technologies, and adaptive control systems will further enhance the performance and reliability of condensers. As power plants adapt to the evolving demands of energy production, condensers will continue to play an essential role in shaping the future of energy systems, driving both efficiency and sustainability.
As the energy landscape continues to evolve, the role of condensers in power plants becomes even more crucial in achieving sustainability, efficiency, and operational resilience. There are several key areas where the development and optimization of condenser technologies are pushing the boundaries of energy production, helping to meet the growing global demand for cleaner energy.
One of the most significant advancements in condenser technology is the integration of waste heat recovery systems. These systems aim to capture heat that would otherwise be wasted in the cooling process and convert it into usable energy. For example, some power plants are using the heat from the condenser to pre-heat water entering the boiler, thereby reducing the energy required to bring the water to steam. This form of heat integration significantly improves the overall thermal efficiency of the plant. Additionally, excess heat from the condenser can be redirected to district heating systems, where it is used for residential and industrial heating. This approach not only maximizes the efficiency of the energy generated but also provides an alternative energy source for communities, reducing the reliance on fossil fuels for heating.
Another promising development in condenser technology is the use of advanced thermal storage systems. In regions where energy demand fluctuates, particularly during peak periods, condensers paired with thermal storage systems can store excess heat during low-demand times and release it during high-demand periods. This can help balance the load on the power grid and ensure a more stable and reliable energy supply. By coupling thermal storage with condensers, power plants can achieve more efficient use of generated energy, contributing to grid stability while minimizing waste.
In addition to waste heat recovery, the efficiency of condensers can be further enhanced through innovations in heat exchanger design. Advances in materials and manufacturing techniques are enabling the production of heat exchangers with improved surface area and thermal conductivity. For instance, enhanced tube designs, such as finned or spiral-wound tubes, can provide better heat transfer by increasing the surface area available for heat exchange. The use of advanced composite materials, such as carbon fiber or ceramics, can also improve the heat transfer rate while reducing the weight and corrosion susceptibility of the condenser. These design improvements help maximize the overall performance of the condenser while minimizing maintenance and operational costs.
The role of condensers in optimizing energy systems extends beyond traditional steam turbines. In combined cycle power plants, for instance, condensers are critical in enhancing the efficiency of both the gas turbine and steam turbine cycles. By effectively managing the heat recovery process between the gas turbine’s exhaust and the steam turbine, condensers can contribute to a significant increase in overall plant efficiency. This is particularly relevant in power plants that seek to maximize the use of available resources, reducing fuel consumption and the environmental impact of energy production.
Moreover, the integration of condensers into renewable energy systems, such as concentrated solar power (CSP) plants and geothermal energy plants, is gaining momentum. In CSP plants, condensers play an essential role in cooling the steam produced by solar collectors. By utilizing the heat captured from the sun, these systems can generate steam and power turbines to produce electricity. Condensers are crucial for maintaining the thermal efficiency of these systems by cooling the exhaust steam and ensuring the continuous operation of the cycle. In geothermal plants, condensers are similarly used to cool the steam extracted from geothermal wells, ensuring that the geothermal energy is effectively harnessed and utilized in electricity generation.
The growing need for efficient, low-carbon energy production has also led to the development of carbon capture and storage (CCS) technologies, where condensers play an indirect but essential role. In CCS systems, carbon dioxide is captured from the exhaust gases of power plants and stored underground to prevent its release into the atmosphere. Condensers can be integrated into these systems to manage the cooling process of the gases before they undergo capture. The use of low-energy, efficient condensers can make the carbon capture process more cost-effective by reducing the additional energy burden placed on the plant while ensuring that the cooling system operates smoothly.
With the increasing focus on energy decarbonization, the future of condenser technology is also linked to the broader transition to renewable energy sources. As renewable energy technologies such as wind and solar become more prevalent, power plants will need to adapt their systems to accommodate intermittent energy generation. Condensers, along with advanced grid management systems, will play an important role in ensuring that power plants can efficiently integrate renewable energy sources into the grid. By improving the flexibility and responsiveness of condensers to varying energy demands, plants will be able to maintain a stable power supply, even during periods of fluctuating renewable energy output.
Further, the continued growth of decentralized energy systems, such as microgrids and combined heat and power (CHP) plants, presents new challenges and opportunities for condenser technology. As more industrial and residential facilities adopt their own power generation systems, condensers will be integrated into smaller-scale energy systems. These decentralized systems will require condensers that are more compact, efficient, and adaptable to varying energy demands. Innovations in condenser design, such as modular or mobile units, will be crucial in providing flexible and scalable solutions for these smaller energy systems.
Moreover, global environmental concerns and stricter regulatory standards are driving the development of more environmentally friendly condenser solutions. There is an increasing emphasis on reducing the overall environmental impact of power plants, particularly in terms of water usage, chemical treatment, and thermal discharge. As such, condensers will need to evolve to comply with stringent water usage and discharge regulations, with a focus on minimizing the thermal pollution in aquatic environments. Innovations such as dry cooling systems, which reduce water consumption by using air instead of water for cooling, are likely to become more common, particularly in regions where water resources are scarce.
Finally, the push toward digitalization and automation in the energy sector is bringing new opportunities for optimizing condenser operations. Advanced monitoring and control systems can continuously track performance metrics, such as steam temperature, pressure, and flow rates, and adjust operations accordingly. These systems can identify inefficiencies in real time and provide operators with actionable insights to improve condenser performance. Furthermore, the integration of artificial intelligence and machine learning in turbine and condenser systems can enhance predictive maintenance capabilities, allowing for more accurate forecasts of equipment lifespan and performance degradation. This proactive approach to maintenance reduces the likelihood of unexpected failures, improves operational efficiency, and extends the life of critical components.
In conclusion, as power generation technologies advance, condensers will continue to evolve to meet the demands of efficiency, sustainability, and adaptability. From waste heat recovery and innovative materials to digital monitoring systems and hybrid cooling methods, the future of condenser technology is poised to support the transformation of the energy industry. As the world transitions to cleaner, more sustainable energy systems, condensers will remain integral to optimizing the performance of power plants and reducing their environmental footprint, ensuring that energy production remains both efficient and environmentally responsible.
The ongoing advancements in condenser technology are not just shaping the future of energy production, but are also contributing to the broader shift toward a more resilient, flexible, and sustainable energy infrastructure. These innovations are vital in addressing global energy challenges, including the need for cleaner energy, water conservation, and the optimization of resources in a rapidly evolving market.
One of the key drivers of change in condenser technology is the growing demand for energy storage solutions. With the increased use of renewable energy sources such as solar and wind, which are inherently intermittent, energy storage is becoming an essential part of the grid infrastructure. Condensers are being integrated into new systems that can store excess heat for later use, providing a means to harness and retain energy that would otherwise be wasted. This stored energy can be released when demand peaks, helping to smooth out fluctuations in supply and ensuring that power plants can operate efficiently even when renewable energy sources are not available. Such energy storage systems can also help reduce the reliance on fossil fuels for peak-load generation, contributing to the overall decarbonization of the energy sector.
Moreover, as the push for net-zero emissions intensifies, the role of condensers in supporting carbon capture technologies is becoming increasingly significant. By capturing and compressing CO2 emissions, power plants can reduce their contribution to climate change. Condensers are integral to this process, ensuring that the heat generated by the carbon capture system is properly managed, thereby improving the overall efficiency of the system. Advanced cooling systems within condensers are being designed to better handle the extra thermal load created by the capture process, which can significantly improve the efficiency and cost-effectiveness of carbon capture and storage (CCS) technologies. Furthermore, this integration can help power plants meet stricter environmental regulations, while providing a pathway for reducing emissions from existing infrastructure.
In addition to their role in CCS, condensers are becoming an essential part of the transition to greener power plants. In particular, there is growing interest in integrating them with geothermal and other low-carbon technologies. For example, in geothermal plants, condensers are used to manage the steam extracted from deep underground sources, cooling it to enable further energy extraction. These plants are a sustainable alternative to conventional fossil fuel-based power plants and have the potential to provide baseload power, reducing reliance on non-renewable resources. Condenser systems play a critical role in maintaining the thermal efficiency and operational stability of geothermal plants, ensuring that energy is harnessed effectively and continuously.
Similarly, hybrid power plants that combine multiple renewable energy sources, such as solar, wind, and geothermal, are emerging as a promising solution for ensuring reliable power generation. Condensers in these systems help manage the different temperature and pressure profiles from each energy source, allowing for more efficient energy conversion and minimizing the loss of heat. By optimizing condenser performance in hybrid systems, power plants can maximize the utilization of available renewable resources, reduce their overall carbon footprint, and offer more consistent energy output despite fluctuations in individual renewable sources.
As energy systems become increasingly complex and interconnected, the importance of advanced monitoring and predictive maintenance technologies for condensers is growing. The integration of IoT (Internet of Things) devices, sensors, and machine learning algorithms allows for real-time monitoring of condenser performance, enabling operators to identify inefficiencies and potential failures before they occur. This proactive approach to maintenance ensures that condensers continue to operate at peak performance, reducing unplanned downtime and extending the life of key components. Additionally, data-driven insights can help optimize energy consumption, enabling power plants to make better-informed decisions about when to operate at full capacity or when to reduce output, based on factors such as demand, weather conditions, and energy prices.
One of the emerging areas in condenser innovation is the use of nanotechnology to enhance heat transfer efficiency. Nanomaterials, with their unique properties at the microscopic scale, can significantly improve the thermal conductivity of materials used in heat exchangers, leading to better heat transfer rates and reduced energy loss. This can be particularly beneficial in air-cooled condensers, which traditionally struggle with lower efficiency compared to water-cooled systems. By utilizing nanomaterials, condensers can achieve higher levels of efficiency, even in challenging environmental conditions. This could make air-cooled systems more competitive with water-based systems, particularly in areas where water scarcity is a concern.
Similarly, the development of advanced coatings and surface treatments is enhancing the durability and performance of condenser components. These coatings can help prevent scaling, fouling, and corrosion, which are common issues in condenser systems, particularly those that use water as a cooling medium. By reducing the need for frequent cleaning and maintenance, these coatings can lower operating costs, increase system longevity, and improve overall efficiency. Additionally, self-cleaning surfaces are being explored, which could further reduce maintenance requirements and enhance the reliability of condensers over time.
The growing integration of renewable energy sources and the push for decarbonization are driving the demand for more efficient, cost-effective condenser technologies. As the industry continues to evolve, the importance of condensers in enabling the transition to cleaner, more sustainable energy systems will only increase. With innovations in materials science, digital technologies, heat recovery, and hybrid energy systems, condensers will continue to play a crucial role in optimizing power plant performance, enhancing energy efficiency, and reducing environmental impact.
As these technologies develop and mature, it is likely that future condensers will be more compact, more adaptable, and capable of operating in diverse environments with higher levels of efficiency. The role of condensers will become even more critical as power plants strive to meet the world’s growing energy demands while minimizing their environmental impact. Therefore, the future of condenser technology holds great promise, not only for the energy industry but also for global efforts to mitigate climate change and transition to a more sustainable energy future.
Control and Monitoring Systems
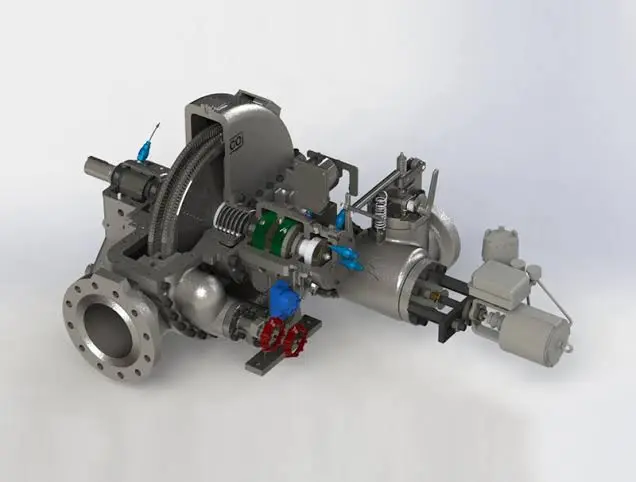
Control and monitoring systems are essential components in modern steam turbine and power plant operations, playing a pivotal role in optimizing performance, ensuring safety, and maintaining operational efficiency. These systems enable power plant operators to oversee and regulate the functioning of various plant components in real time, including steam turbines, condensers, boilers, and auxiliary systems. The evolution of control and monitoring technologies has significantly transformed how power plants are managed, with advancements in automation, data analytics, and artificial intelligence (AI) further enhancing their capabilities. The integration of sophisticated control and monitoring systems in power plants has enabled operators to achieve higher levels of reliability, efficiency, and flexibility, which are critical in the face of increasing energy demand and environmental sustainability goals.
One of the fundamental aspects of control and monitoring systems is the automation of plant processes. Traditional power plant operations often required manual intervention and routine checks, but with the advent of automation, most operations can now be controlled remotely and dynamically adjusted. Automation systems include Programmable Logic Controllers (PLCs) and Distributed Control Systems (DCS), which are used to monitor and control various aspects of the plant. These systems can collect data from a wide range of sensors and equipment, such as temperature, pressure, flow, and vibration, providing real-time feedback that allows for efficient process control. The automation of critical operations reduces the potential for human error, improves consistency in plant performance, and enables operators to respond to changes in load demand or system conditions more quickly.
In modern steam turbines and power plants, real-time monitoring is crucial for detecting and addressing issues before they become significant problems. Through advanced sensors and data acquisition systems, operators are provided with continuous information on the operational health of the plant. This data includes vital parameters like turbine speed, steam pressure, temperature, and vibration, as well as more complex metrics related to efficiency and emissions. By tracking these variables, control systems can identify abnormalities such as deviations from optimal operating conditions, signaling the need for corrective action. These monitoring systems not only prevent equipment damage but also help maintain optimal efficiency, reducing fuel consumption and wear on machinery.
One of the most important advancements in control and monitoring technology is the use of predictive analytics. Traditionally, power plant operators would react to equipment failure or performance issues as they occurred, but with the integration of predictive analytics, operators can forecast potential problems before they happen. Using machine learning algorithms and historical data, predictive analytics can identify patterns and trends in equipment performance, providing early warning signs of impending failures. This proactive approach to maintenance, known as predictive maintenance, can help minimize downtime and extend the lifespan of critical components. For example, predictive analytics can be used to monitor the health of turbine blades, identifying early signs of erosion or cracking, allowing for timely inspections or repairs before a failure occurs.
AI and machine learning are increasingly being used to enhance the performance of control and monitoring systems. These technologies can analyze large datasets more efficiently than traditional methods, identifying patterns and correlations that might be difficult for human operators to detect. In steam turbine control systems, AI can optimize the operation of the turbine by adjusting parameters in real time based on the changing conditions within the plant. This includes adjusting steam flow rates, pressure settings, and turbine speed to maintain optimal performance and maximize efficiency. By learning from past operations, AI algorithms can continually improve their predictions and optimize the control strategies, further enhancing the plant’s overall performance.
In addition to improving efficiency and reliability, modern control and monitoring systems are also designed to ensure the safety of plant personnel and the surrounding environment. Power plants involve high-pressure steam systems, rotating machinery, and complex electrical systems, all of which pose significant safety risks if not properly managed. Control systems are equipped with safety protocols that can detect unsafe conditions and automatically shut down or adjust operations to prevent accidents. For example, if a steam turbine detects an unusually high temperature or pressure, the control system can initiate a shutdown sequence to prevent overheating or damage. Similarly, emissions monitoring systems are employed to ensure that the plant complies with environmental regulations, with sensors continuously measuring pollutants such as carbon dioxide, nitrogen oxides, and sulfur dioxide. If emissions exceed permissible levels, the control system can take corrective actions, such as adjusting combustion rates or activating additional scrubbing systems to reduce pollutants.
Another critical aspect of control and monitoring systems is the integration with energy management systems (EMS). EMS allow for the optimization of energy usage across the entire power generation process, ensuring that electricity is produced at the lowest possible cost while maintaining grid stability. These systems can manage the distribution of power, balancing supply and demand in real time, and enabling plants to respond to fluctuations in grid demand. For example, when demand spikes, the EMS can increase power output by adjusting the operation of steam turbines or activating backup generators. Conversely, during periods of low demand, the EMS can reduce output or temporarily shut down non-essential systems, ensuring that energy production remains cost-effective.
As power plants increasingly integrate renewable energy sources such as solar and wind, control and monitoring systems must be adaptable to these new energy inputs. Renewable energy sources are variable by nature, with output depending on weather conditions and time of day. Control systems must be able to quickly respond to fluctuations in renewable generation, balancing it with traditional power sources to ensure a consistent and reliable supply of electricity to the grid. The integration of energy storage systems, such as batteries, with power plants is another growing trend, and control systems must be capable of managing the flow of energy between storage and generation sources. This requires advanced algorithms and real-time data analysis to optimize energy storage and discharge cycles, maximizing the value of renewable energy while minimizing reliance on fossil fuels.
Digital twins, which are virtual models of physical assets or processes, are also making their way into control and monitoring systems. Digital twins allow operators to simulate the behavior of turbines, boilers, and other key components in real-time. By using data from sensors and historical performance records, digital twins can provide a digital replica of the entire power plant, allowing operators to analyze different scenarios and test potential changes to the system without risking damage to the actual equipment. This can help improve decision-making, optimize maintenance schedules, and reduce downtime by simulating maintenance interventions before they are applied to the physical plant.
Furthermore, the future of control and monitoring systems is closely tied to the growing trend of plant digitalization. The integration of Internet of Things (IoT) devices, cloud computing, and advanced data analytics is revolutionizing how power plants are operated and maintained. By connecting all equipment and systems within a plant to a centralized digital platform, operators can gain a comprehensive view of plant performance from any location. Cloud-based solutions allow for the storage and analysis of vast amounts of data, providing insights that can lead to better decision-making, improved resource allocation, and reduced operational costs. Remote monitoring capabilities enable operators to track performance, troubleshoot issues, and perform diagnostic tasks from anywhere in the world, improving the flexibility and responsiveness of plant operations.
In conclusion, the development of advanced control and monitoring systems is a key factor in the modern power plant’s ability to optimize performance, improve efficiency, enhance safety, and reduce operational costs. From predictive maintenance and AI optimization to integration with renewable energy sources and energy management systems, these systems are driving the future of power generation. As the energy industry continues to evolve toward a cleaner, more sustainable future, control and monitoring technologies will remain essential for ensuring that power plants can meet growing demand while minimizing their environmental impact and operational risks. The increasing digitalization of the energy sector will further empower operators to make data-driven decisions that optimize plant performance and support the transition to a more sustainable energy future.
The continued development of control and monitoring systems in power plants is increasingly focused on enhancing the ability to predict, prevent, and mitigate operational disruptions. As power plants become more complex and interconnected, the demand for more sophisticated systems capable of managing a diverse range of inputs and outputs grows. This includes not only the need for advanced control algorithms but also the integration of advanced data acquisition systems that capture more granular information from a variety of sensors placed throughout the plant. These sensors monitor everything from mechanical vibrations and thermal stress on turbines to environmental parameters like humidity and air quality, providing a holistic view of plant performance.
In addition to predictive maintenance, the ability to optimize operational performance is another critical benefit of modern control systems. By analyzing historical and real-time data, these systems can generate recommendations for improving efficiency. This can involve adjusting the operation of turbines to better match the real-time availability of fuel, steam, or electricity, or making minor tweaks to system parameters such as temperature and pressure to improve overall efficiency. As the energy sector faces pressure to reduce fuel consumption and cut down on emissions, the ability to optimize operational performance becomes increasingly important for maintaining profitability while adhering to strict environmental standards.
One key area where control and monitoring systems play an essential role is in improving the overall efficiency of energy generation, particularly in combined-cycle power plants. These plants integrate both gas and steam turbines to extract the maximum possible energy from a given fuel source. The steam turbine’s performance is highly dependent on the operation of the gas turbine, and control systems must ensure that both turbines are operating in harmony. By continuously monitoring the interaction between the gas and steam turbines, control systems can adjust operational parameters to ensure that the overall plant efficiency is maximized. For instance, during periods of high demand, the control system may ramp up the gas turbine’s output, which in turn generates more exhaust heat for the steam turbine, thus improving the overall efficiency of the plant.
Moreover, the integration of renewable energy sources into the grid presents unique challenges that control and monitoring systems are well-equipped to handle. Renewable energy sources, such as solar and wind, are variable and unpredictable, and their output fluctuates based on weather patterns and time of day. Control systems help to integrate these renewable sources by dynamically adjusting the operation of steam turbines and other power generation assets to compensate for these fluctuations. For instance, during periods of high renewable energy production, the control system can reduce output from conventional sources, like steam turbines, to avoid overloading the grid. Conversely, when renewable energy output decreases, the control system can increase the output from steam turbines or other backup sources to meet demand. In this way, control and monitoring systems play a key role in maintaining grid stability while enabling the integration of cleaner, renewable energy sources.
Another area where modern control and monitoring systems have shown significant potential is in managing energy storage systems. As the role of batteries and other forms of energy storage expands, it is increasingly important to efficiently integrate storage with the generation of electricity. Control systems must ensure that stored energy is properly distributed across the grid, making sure that excess energy from peak generation times is captured and available for use during periods of high demand or when renewable generation is low. In addition, the control system needs to monitor the health of the storage system, tracking parameters like state of charge, temperature, and degradation, to ensure that it operates efficiently and lasts for as long as possible.
Further advancements in control and monitoring systems are focusing on the increased use of artificial intelligence (AI) and machine learning (ML) techniques. These technologies can analyze complex datasets, identify emerging trends, and recommend adjustments that human operators might overlook. By continuously analyzing data from various parts of the plant, AI algorithms can learn from past operational patterns and make real-time adjustments to improve plant efficiency. This process of continual learning can also enhance the system’s ability to predict future events, such as changes in grid demand, or identify potential failures before they happen, improving both the reliability and operational lifespan of plant equipment.
The adoption of digital technologies such as edge computing is another trend in the evolution of control and monitoring systems. Edge computing involves processing data locally at the source, rather than sending it to a central cloud server for analysis. This reduces latency and improves response times, which is particularly important in critical systems like power plants, where real-time decision-making is essential. By incorporating edge computing, control and monitoring systems can provide faster insights and enable more agile responses to changing conditions. For example, in the case of sudden shifts in grid demand, the control system can quickly adjust the output of steam turbines without waiting for data to be processed in the cloud, helping to maintain grid stability.
Moreover, the integration of blockchain technology into control and monitoring systems is a developing trend aimed at enhancing security, transparency, and data integrity in power plants. By utilizing decentralized ledgers, blockchain can ensure that data collected from plant sensors and monitoring systems is tamper-proof and securely stored. This can be especially important for compliance with regulatory requirements, where accurate records of operational parameters, emissions, and energy production are essential. Blockchain can also be used to track the flow of energy between different sources, ensuring that renewable energy credits and carbon offsets are properly accounted for.
Finally, as the push for sustainability continues, control and monitoring systems are being designed to not only optimize the performance of power plants but also to help reduce their environmental impact. These systems can track energy consumption in real time and adjust operations to reduce fuel usage and emissions. In addition, they help ensure that power plants meet regulatory requirements for emissions by monitoring pollutant levels and triggering corrective actions when necessary. This can include adjusting combustion rates, optimizing the use of scrubbing technologies, or reducing waste heat. In this way, control systems help plants comply with increasingly stringent environmental standards, while also improving their overall efficiency.
As power generation continues to evolve, control and monitoring systems will play an increasingly critical role in maintaining the operational efficiency, safety, and environmental sustainability of plants. Their ability to handle complex data, integrate renewable energy sources, predict maintenance needs, and optimize performance will be key factors in the success of future energy systems. The ongoing advancements in artificial intelligence, automation, and digital technologies promise to further enhance the capabilities of control and monitoring systems, enabling power plants to operate more efficiently, reduce costs, and minimize their environmental footprint. Ultimately, these systems will be central to ensuring that the energy sector can meet the growing demand for electricity while transitioning to a cleaner, more sustainable future.
As the energy sector moves towards greater integration of renewable energy sources and decarbonization, the role of control and monitoring systems becomes even more pivotal in ensuring the reliability and stability of power grids. In the context of steam turbines and other power generation systems, these technologies are becoming essential for managing not only conventional thermal power generation but also increasingly complex combinations of renewable sources and energy storage solutions. The flexibility of modern control systems allows power plants to adapt to the dynamic nature of energy production and consumption, a key factor in transitioning to a more sustainable energy landscape.
A particularly important function of control and monitoring systems is the ability to coordinate the operation of multiple generation sources in a flexible and responsive manner. In the case of hybrid power plants, which combine both renewable and conventional generation sources, control systems play a crucial role in managing the flow of energy between different sources and ensuring grid stability. These systems can automatically adjust the output of turbines, activate energy storage devices, and manage the connection to the grid in real-time to balance supply and demand. The ability to monitor the status of both renewable and conventional generation sources, as well as energy storage, is essential for maintaining a consistent and reliable power supply.
Energy storage systems, such as batteries and pumped hydro storage, are becoming increasingly important in supporting the integration of intermittent renewable sources like wind and solar power. These energy storage systems allow excess electricity generated during periods of low demand or high renewable output to be stored and used when needed. Control systems are responsible for managing the charging and discharging of these energy storage systems in response to fluctuations in demand and generation. By optimizing the use of stored energy, control systems help ensure that power plants can maintain reliable generation while minimizing the need for backup fossil fuel plants.
The shift towards a decentralized energy grid, driven by the rise of distributed energy resources (DERs), also requires advanced control and monitoring systems to maintain system stability. Distributed energy resources include solar panels, small-scale wind turbines, and local energy storage systems that are often located at or near the point of consumption. Managing the integration of these distributed resources into the larger power grid can be challenging, as the power they generate is not always predictable and can fluctuate significantly. Control systems equipped with advanced forecasting and optimization capabilities can help manage these fluctuations by adjusting the operation of larger power plants, integrating energy storage, and ensuring that grid stability is maintained even in the face of variable generation.
With the increasing sophistication of control systems, there is also a growing focus on the cyber security of power plants. As digital systems become more integrated into plant operations, the risk of cyberattacks and data breaches grows. A successful cyberattack on a power plant’s control or monitoring system could have serious consequences, including power outages, equipment damage, and safety hazards. Therefore, security protocols are being designed to ensure that control systems are protected from cyber threats. These systems include encrypted communication channels, multi-factor authentication for system access, and regular security audits to ensure that the latest cybersecurity measures are in place.
The shift towards digitalization and the growing reliance on real-time data analysis also enables more efficient plant operations. Control and monitoring systems are increasingly utilizing advanced machine learning models to analyze vast amounts of operational data, predict potential performance issues, and recommend adjustments for optimization. For example, these systems can detect inefficiencies in turbine performance, suggest changes to operating conditions, and even predict potential failures before they occur. This predictive capability is particularly important in reducing unplanned downtime, extending the life of critical components, and lowering maintenance costs.
Additionally, the ongoing development of digital twins is revolutionizing the way power plants are managed. Digital twins are virtual replicas of physical assets or systems, created using data collected from sensors, equipment, and historical performance records. These virtual models allow operators to simulate various scenarios, evaluate the impact of potential changes to the plant, and test optimization strategies before they are implemented. By leveraging digital twins, control systems can test out different operating conditions in a risk-free virtual environment, leading to more informed decision-making and better overall performance.
Another emerging trend in the development of control and monitoring systems is the use of edge computing, which allows for the processing of data closer to the source of generation, rather than relying on centralized cloud-based platforms. This reduces latency and ensures faster response times, which is critical for real-time control of complex systems like steam turbines. Edge computing also enhances the ability to handle large volumes of data, improving the scalability of control and monitoring systems as plants become more digitized. The ability to process data locally at the source reduces reliance on network infrastructure, making control systems more resilient and flexible.
The environmental impact of energy generation is another key area where control and monitoring systems are making a difference. As power plants work to reduce emissions and improve their environmental performance, these systems help to monitor emissions in real-time and adjust operations to stay within regulatory limits. For example, in a steam turbine-based power plant, control systems can adjust the combustion process, fuel-to-air ratios, and exhaust gas treatment to minimize the release of pollutants such as nitrogen oxides (NOx), sulfur oxides (SOx), and carbon dioxide (CO2). By continuously monitoring these variables, control systems ensure that emissions remain within permissible levels and help plants meet ever-stricter environmental regulations.
Furthermore, modern control and monitoring systems are enabling the transition to more flexible and sustainable energy systems by supporting the operation of microgrids and virtual power plants. Microgrids are localized grids that can operate independently or in conjunction with the main power grid. Control systems are essential for managing the energy flow within microgrids, optimizing the use of local renewable energy generation, energy storage, and backup power sources. Virtual power plants (VPPs), on the other hand, aggregate the capacities of distributed energy resources to provide grid services. Control systems help to coordinate the operation of these decentralized resources, enabling them to act as a single unified entity that can support grid stability and provide services like demand response and frequency regulation.
In conclusion, the continued evolution of control and monitoring systems is critical to the future of steam turbine operations and the broader energy sector. These systems are enhancing the efficiency, flexibility, and sustainability of power plants by optimizing the performance of turbines, integrating renewable energy, managing energy storage, and ensuring grid stability. As technology advances, control and monitoring systems will become even more sophisticated, enabling operators to make data-driven decisions that improve performance, reduce operational costs, and minimize environmental impact. The ongoing development of AI, machine learning, digital twins, and edge computing will further revolutionize the way power plants are operated, making them more efficient, resilient, and adaptable to the demands of a rapidly changing energy landscape.
As the energy landscape continues to evolve with advancements in technology and increasing demands for cleaner, more sustainable energy, control and monitoring systems are poised to play an even more significant role in the operational success of steam turbines and other power generation technologies. These systems are helping to not only optimize efficiency and reduce operational costs but also support the integration of renewable energy sources, maintain grid stability, and reduce emissions. The convergence of digital technologies, such as artificial intelligence, edge computing, and machine learning, is enabling the development of more sophisticated control systems that enhance performance across all stages of power generation.
The integration of renewable energy into traditional power generation systems is one of the most important challenges that control and monitoring systems must address. Solar and wind energy are variable in nature, meaning their availability fluctuates depending on time of day, weather conditions, and geographic location. Control systems play a crucial role in managing the variability of these renewable sources by coordinating the response of steam turbines and other conventional generation sources. This is especially important in ensuring that grid demand is met without overloading the system or causing instability. During periods of high renewable generation, control systems can adjust the output of steam turbines to ensure that power generation is in sync with grid demand, minimizing excess generation and curtailment of renewable energy.
In addition, control and monitoring systems are key to managing hybrid systems that combine renewable energy sources with traditional turbines. These hybrid systems may involve steam turbines, gas turbines, batteries, or other energy storage technologies, working together to provide a reliable and flexible power supply. The control system must be capable of managing the interaction between these different generation sources, ensuring that each is operating at optimal efficiency and responding to grid conditions. For example, when renewable generation is high, control systems may reduce the operation of conventional steam turbines, increasing the output of energy storage systems instead to balance grid demand. During periods of low renewable generation, the system can ramp up the operation of steam turbines to ensure that there is enough power to meet demand.
The use of predictive analytics and real-time data monitoring within these systems is crucial for identifying and addressing potential issues before they lead to downtime or equipment failure. By analyzing data from various plant components, such as the turbine rotor, condenser, and extraction systems, control systems can identify performance trends and detect anomalies that might signal the need for maintenance or adjustments. This predictive capability reduces the likelihood of unplanned outages, which can be costly and disruptive. For instance, early detection of vibrations or thermal stresses in the turbine rotor can trigger preventative maintenance, avoiding more serious damage and extending the lifespan of critical equipment.
Another aspect of control and monitoring systems is their ability to improve the performance of energy storage systems, which are becoming increasingly important in supporting renewable energy integration. As energy storage technologies such as batteries, pumped hydro, and compressed air storage systems grow in importance, control systems play a key role in managing the charging and discharging cycles of these systems. By ensuring that energy is stored during times of excess generation and released during peak demand, control systems help optimize the use of renewable energy, minimize waste, and improve overall grid efficiency. This requires real-time monitoring and adjustment of the flow of electricity between generation sources, energy storage, and the grid.
The role of artificial intelligence and machine learning in enhancing the capabilities of control and monitoring systems cannot be overstated. These technologies allow for the continuous analysis of large volumes of operational data to optimize performance in real time. Machine learning algorithms can identify patterns in the data that human operators may miss, enabling more accurate predictions about system behavior and potential issues. For example, AI-driven control systems can predict when a turbine may need maintenance based on usage patterns and sensor data, allowing operators to address issues proactively rather than reactively. Furthermore, AI can support optimization by continuously adjusting the operating conditions of turbines, boilers, and other plant components to improve efficiency and reduce fuel consumption.
One particularly exciting development is the application of digital twins, which are virtual representations of physical assets or systems created using real-time data. In the context of steam turbines, digital twins can simulate the behavior of turbines, condensers, and other critical components, providing a powerful tool for optimizing performance. By testing different operating scenarios in a virtual environment, operators can identify the most efficient operating conditions without risking damage to actual equipment. This ability to simulate and optimize performance remotely reduces downtime and supports informed decision-making. Additionally, digital twins can be used to predict the future behavior of systems based on historical data, which can help identify potential failures or inefficiencies before they become major problems.
Edge computing, which involves processing data closer to the source of generation, is another innovation that is enhancing the functionality of control systems. With edge computing, data from sensors and monitoring devices can be analyzed locally in real-time, rather than being transmitted to a central server for processing. This reduces the time it takes for control systems to respond to changes in system conditions and improves operational flexibility. Edge computing is particularly valuable in large-scale power plants, where large amounts of data are generated by turbines, boilers, condensers, and other equipment. By processing this data at the edge, control systems can make faster, more accurate decisions, improving overall plant efficiency and reducing delays caused by network communication.
Cybersecurity is also becoming an increasingly important consideration as control systems become more interconnected and digitalized. As power plants rely more heavily on digital control and monitoring systems, the risk of cyberattacks increases. A successful cyberattack on a control system could have serious consequences, ranging from power outages to equipment damage and even safety hazards. Therefore, ensuring the cybersecurity of these systems is a priority. Control systems are increasingly being designed with robust security features, including encrypted communications, multi-factor authentication, and real-time threat detection, to protect against cyber threats.
Moreover, as part of a broader push for sustainability and environmental responsibility, control and monitoring systems are being designed to reduce the environmental impact of power generation. By optimizing the operation of turbines, reducing fuel consumption, and minimizing emissions, these systems contribute to the overall sustainability of power plants. For example, they can help steam turbines operate at optimal efficiency, reducing the amount of fuel consumed for each unit of electricity generated. Additionally, control systems can monitor emissions in real-time and adjust plant operations to ensure compliance with environmental regulations.
Looking ahead, the future of control and monitoring systems in power generation will likely involve continued advancements in artificial intelligence, data analytics, and automation. These technologies will enable more efficient, flexible, and resilient power generation systems, capable of meeting the growing demand for clean energy while minimizing operational costs. By enhancing the integration of renewable energy sources, improving the performance of energy storage systems, and optimizing the operation of steam turbines, control and monitoring systems will continue to play a central role in shaping the future of the energy sector.
In conclusion, control and monitoring systems are evolving to meet the challenges of modern power generation. With the increasing integration of renewable energy, the need for greater operational flexibility, and the growing importance of sustainability, these systems are critical for ensuring that power plants can operate efficiently and reliably. Through innovations in artificial intelligence, edge computing, predictive analytics, and digital twins, control systems are improving the efficiency, reliability, and performance of steam turbines and other power generation technologies. As the energy sector continues to evolve, these systems will be key to maintaining grid stability, reducing emissions, and ensuring the delivery of clean, reliable energy to meet the demands of the future.
Control and Monitoring Systems
Control and monitoring systems play a crucial role in the efficient and reliable operation of steam turbines and other power generation equipment. These systems are responsible for managing and optimizing various aspects of turbine operation, from startup and shutdown procedures to real-time adjustments for load changes, pressure, temperature, and other operational parameters. By continuously monitoring the performance of turbines and associated equipment, control and monitoring systems help to ensure that plants operate within safe, efficient, and optimal parameters. Their integration with various digital technologies has become increasingly important as power plants move towards greater automation, integration of renewable energy sources, and the adoption of advanced analytics for predictive maintenance and performance optimization.
One of the primary functions of control and monitoring systems is the regulation of turbine performance, ensuring that the turbine operates at optimal efficiency while meeting the required load and power output demands. These systems gather data from various sensors and instruments installed throughout the plant, which monitor key parameters such as steam pressure, temperature, turbine speed, vibration, and exhaust conditions. Based on this data, the control system adjusts the operation of the steam turbine and other components to maintain performance and respond to changing conditions. For example, if a turbine experiences a sudden fluctuation in load, the control system will automatically adjust steam flow, temperature, and pressure to maintain stable operation. In this way, the system contributes to maintaining a consistent, reliable power supply.
In addition to regulating turbine operation, control and monitoring systems also help prevent damage to turbines and other critical equipment by providing real-time data that allows operators to detect and address potential issues before they become major problems. By continuously monitoring various parameters and comparing them to predefined operational thresholds, these systems can identify anomalies or deviations that may indicate a malfunction or degradation in performance. For example, if a turbine begins to experience excessive vibration or overheating, the control system can trigger an alarm to alert operators, who can then take corrective action to prevent further damage or failure. This early detection capability is essential for reducing downtime, extending the life of equipment, and minimizing costly repairs or replacements.
As power generation becomes more complex, with the increasing integration of renewable energy sources and the push for greater efficiency and sustainability, control and monitoring systems are becoming more sophisticated. Modern power plants are often equipped with advanced digital technologies that allow for real-time data collection, analysis, and optimization of plant performance. These systems can track the performance of individual turbines, boilers, and other components, identify patterns in energy consumption, and make adjustments to improve overall efficiency. For example, in a combined-cycle power plant, where gas turbines are used in conjunction with steam turbines, control systems can optimize the sequencing and interaction between the two turbine types to maximize overall efficiency. Similarly, in plants that use renewable energy sources like solar or wind power, control systems help to manage the variable nature of these sources by adjusting the output of turbines or integrating energy storage solutions to ensure grid stability.
One of the key trends in modern control and monitoring systems is the use of predictive analytics and machine learning. These technologies enable control systems to not only monitor the current performance of turbines but also to predict future behavior based on historical data. Machine learning algorithms can analyze large volumes of data to identify patterns and trends, allowing operators to make more informed decisions about maintenance, optimization, and performance. For example, a predictive maintenance algorithm might analyze data on turbine vibration and temperature to predict when a component is likely to fail, allowing for timely maintenance and preventing unplanned downtime. These systems can also adjust operational parameters in real-time to optimize turbine performance, improving efficiency and reducing fuel consumption.
Another significant advancement in control and monitoring systems is the use of digital twins. A digital twin is a virtual representation of a physical asset, such as a steam turbine, that is created using real-time data and advanced simulation tools. By creating a digital twin of a turbine, operators can simulate various operating conditions and test different scenarios without affecting the actual turbine. This allows for more informed decision-making, better planning, and improved optimization of turbine performance. For example, operators can use a digital twin to test the effects of different steam flow rates or pressure conditions on turbine efficiency, helping to identify the most optimal operating parameters. Digital twins also enable predictive maintenance, as they can simulate the behavior of the turbine over time and predict potential failures or performance issues before they occur.
Control and monitoring systems also contribute to the overall safety and reliability of power plants by ensuring compliance with regulatory standards and industry best practices. For example, these systems are designed to monitor emissions, ensuring that power plants remain within the legal limits for pollutants such as carbon dioxide (CO2), nitrogen oxides (NOx), and sulfur dioxide (SO2). They can automatically adjust the combustion process or activate pollution control equipment to reduce emissions and ensure compliance with environmental regulations. Additionally, control systems can monitor equipment performance to ensure that turbines and other components are operating safely and efficiently, helping to prevent accidents, damage, or failures that could pose a risk to plant personnel or the surrounding environment.
As the energy sector continues to evolve, the role of control and monitoring systems in ensuring efficient, reliable, and sustainable power generation will only grow more important. The integration of renewable energy sources, the adoption of advanced digital technologies, and the ongoing push for greater efficiency and sustainability are driving the development of more sophisticated control systems that can optimize the operation of steam turbines and other power generation technologies. As these systems become more advanced, they will continue to provide valuable insights into plant performance, enabling operators to make better decisions, reduce operational costs, and improve the overall efficiency of power generation. Furthermore, the continued integration of machine learning, predictive analytics, and digital twins will enhance the ability of control and monitoring systems to optimize turbine performance, predict and prevent failures, and ultimately contribute to the ongoing transformation of the global energy landscape.
In conclusion, control and monitoring systems are vital to the successful operation of steam turbines and other power generation technologies. They enable power plants to operate efficiently, safely, and reliably by continuously monitoring performance, detecting potential issues, and making real-time adjustments to optimize operations. With advancements in digital technologies, such as predictive analytics, machine learning, and digital twins, control systems are becoming more powerful and capable of driving improvements in plant performance. As the energy sector moves towards greater integration of renewable energy, increased automation, and sustainability, control and monitoring systems will continue to play an essential role in ensuring that power plants meet the growing demand for clean, reliable, and efficient energy.
Control and monitoring systems continue to evolve as advancements in technology create more sophisticated tools for managing power generation systems. The integration of IoT (Internet of Things) sensors, cloud computing, and edge computing is enabling real-time, granular monitoring of turbines, boilers, condensers, and other components. These systems are not just reactive but are increasingly proactive, using predictive algorithms to anticipate system behavior and prevent failures before they occur. As these technologies become more advanced, power plants are not only able to improve the operational efficiency of their turbines but also reduce costs associated with unplanned downtime and inefficient operations.
In particular, edge computing is a growing trend that enhances control and monitoring capabilities. By processing data closer to the source of data generation (i.e., directly at the turbine or sensor level), edge computing reduces latency and allows for quicker responses to real-time changes in turbine operation. This is crucial in situations where swift adjustments are needed to prevent damage or to optimize performance. For instance, if a sudden fluctuation in turbine speed is detected, an edge-based system can immediately adjust parameters such as steam flow or pressure without the delays caused by transmitting data to a central server. This enhances both operational flexibility and responsiveness, which are essential in maintaining the reliability of the power generation system.
Moreover, the integration of control and monitoring systems with broader energy management platforms is allowing for better coordination across the entire power plant. These systems can now monitor not just individual turbines but also entire systems that include multiple generation sources, such as combined-cycle plants or hybrid systems that incorporate renewable energy. The coordination between steam turbines, gas turbines, solar panels, and energy storage systems (like batteries or pumped hydro) is made possible through advanced control systems. For example, when the output from a renewable energy source like wind or solar is high, the system may automatically reduce the output of steam turbines and shift to storage or other backup generation to ensure grid stability. Conversely, during periods of low renewable energy generation, steam turbines can be ramped up to ensure that power supply meets demand.
The increasing digitization of control and monitoring systems is also facilitating more effective data management and optimization of energy consumption. Big data analytics are being used to analyze vast amounts of operational data from turbines, compressors, condensers, and boilers. These insights help to identify performance trends, forecast energy demand, and optimize energy use. Advanced analytics can help identify inefficiencies that may not be immediately obvious, such as minor discrepancies in turbine efficiency that, over time, lead to significant performance losses. By addressing these inefficiencies early on, operators can improve the long-term performance of the plant and reduce fuel consumption, contributing to both cost savings and a reduction in environmental impact.
As we move into the future, cybersecurity will be a critical area of focus for control and monitoring systems. With the increased reliance on interconnected digital systems, power plants are more vulnerable to cyber threats than ever before. Any disruption to the control systems could have serious consequences, potentially leading to outages, equipment damage, or even safety hazards. Therefore, ensuring the security of these systems is paramount. This includes implementing robust encryption protocols, access controls, and continuous monitoring for potential vulnerabilities or cyberattacks. The implementation of cybersecurity measures in control and monitoring systems is essential not only for protecting plant assets but also for ensuring the safety and integrity of the grid and the broader energy infrastructure.
The use of digital twins in control systems is another exciting development. A digital twin is a virtual representation of a physical asset or system, and in the case of turbines, it is a real-time simulation of the turbine’s operation. Digital twins enable operators to perform virtual testing of turbine components, predict potential failures, and optimize performance without the risk of damaging physical equipment. By running simulations of various operating conditions, operators can identify the most efficient parameters for turbine operation and fine-tune control strategies for better performance. Additionally, digital twins can provide predictive insights, allowing operators to schedule maintenance before a problem becomes critical, thus minimizing downtime and avoiding costly repairs.
The shift toward more decentralized and distributed energy generation systems also calls for new approaches to control and monitoring. With the growing prevalence of microgrids, distributed energy resources, and virtual power plants, the need for flexible and scalable control systems becomes even more critical. These systems must be capable of managing multiple, often diverse, energy sources that may not be directly connected to a central grid. This involves balancing the interaction between renewable generation, energy storage, and traditional generation systems to meet local demand while ensuring grid stability. Advanced control and monitoring systems that can operate in real-time, aggregate data from diverse sources, and make decisions autonomously are essential for the success of these decentralized systems.
In addition to their role in operational optimization, control and monitoring systems are increasingly being leveraged for sustainability efforts. By improving the overall efficiency of steam turbines and other power generation equipment, these systems help reduce fuel consumption, lower emissions, and increase the overall sustainability of power plants. For example, by optimizing the combustion process or adjusting steam conditions, control systems can minimize fuel waste and reduce greenhouse gas emissions. Furthermore, by monitoring emissions in real-time and automatically adjusting operations, control systems help ensure that power plants remain compliant with environmental regulations and contribute to the transition toward cleaner energy production.
Another key trend is the growing role of automation in power plants. Control and monitoring systems are becoming increasingly autonomous, reducing the need for manual intervention. Advanced automation allows plants to operate with minimal human oversight, improving efficiency and safety while also reducing labor costs. Automation systems can take over routine tasks such as adjusting steam flows, managing pressure levels, and responding to changes in load, freeing up operators to focus on more complex or critical issues. This shift toward greater automation is also helping to improve safety, as systems can detect and respond to hazardous conditions more quickly than human operators.
Looking ahead, the integration of artificial intelligence (AI) and machine learning (ML) will continue to enhance the capabilities of control and monitoring systems. AI and ML algorithms can process and analyze vast amounts of operational data, enabling more precise predictions about turbine performance, identifying trends in energy consumption, and optimizing the response to changing grid conditions. By continuously learning from operational data, AI-driven systems can improve their decision-making over time, ensuring that turbines operate at peak efficiency under a variety of conditions. This could lead to more significant reductions in fuel consumption, greater operational flexibility, and improved overall performance.
In conclusion, the evolution of control and monitoring systems is reshaping the landscape of steam turbine operation and power generation as a whole. With the integration of advanced digital technologies, predictive analytics, and automation, these systems are enabling more efficient, reliable, and sustainable power generation. As the energy sector continues to shift towards greater reliance on renewable sources, energy storage, and decentralized generation, control and monitoring systems will play an increasingly critical role in ensuring that plants operate efficiently, safely, and in harmony with the broader energy grid. The future of power generation lies in the seamless integration of these advanced systems, which will continue to drive innovation, reduce operational costs, and contribute to the global push for cleaner energy.
As control and monitoring systems become more sophisticated, they are also integrating with the broader energy ecosystem. The convergence of digital technologies, such as the Internet of Things (IoT), artificial intelligence (AI), machine learning (ML), and big data analytics, is allowing for greater collaboration between different energy systems and plants. The integration of these technologies enables real-time monitoring, enhanced decision-making, and predictive capabilities, offering the potential for significant improvements in operational efficiency and reliability.
One key area where these systems are having a profound impact is in predictive maintenance. Traditionally, power plants have relied on scheduled maintenance and inspections to ensure the proper functioning of turbines and other critical equipment. However, predictive maintenance powered by AI and machine learning algorithms can analyze data from sensors embedded within turbines to detect early signs of wear, vibration, temperature anomalies, or potential failures. By continuously monitoring the condition of equipment, the system can predict when components are likely to fail, allowing operators to perform maintenance before a failure occurs. This proactive approach reduces unplanned downtime, minimizes repair costs, and extends the lifespan of critical assets.
Furthermore, predictive maintenance can help optimize parts inventory, reduce unnecessary maintenance interventions, and prevent overstocking of spare parts. With more accurate insights into when a part will need replacement or servicing, operators can streamline their maintenance schedules and avoid the costly and inefficient practice of over-servicing equipment. This helps to lower operational costs and improve the overall cost-effectiveness of the power generation process.
Additionally, digital twins play a growing role in control and monitoring systems. As virtual models of physical assets, digital twins allow operators to simulate the behavior of turbines and other components under various conditions. By monitoring the performance of actual turbines in real time, operators can compare their real-world performance to the predictions made by the digital twin model. This enables operators to quickly identify any discrepancies and make necessary adjustments to optimize performance. Moreover, digital twins are highly valuable for training operators, as they provide a safe environment for learning how turbines will behave in different scenarios. This virtual training helps operators gain experience without the risks associated with training on live equipment.
Control systems are also evolving to handle the increasing complexity of energy networks. As energy generation becomes more decentralized and reliant on renewable sources such as wind, solar, and biomass, control systems must adapt to accommodate fluctuating power outputs and varying grid conditions. Smart grids, which integrate advanced monitoring and control systems, are capable of optimizing the distribution of energy across multiple generation sources. These systems can automatically adjust energy output to match demand, ensuring that energy supply is consistent even as renewable energy sources experience intermittent fluctuations. For example, when wind speeds decrease or solar radiation is low, the system can increase output from traditional steam turbines to compensate for the loss of renewable generation.
The integration of energy storage systems, such as batteries and pumped hydro storage, into power plants is another trend that has enhanced the flexibility of control systems. Energy storage helps to stabilize the grid by storing excess energy when demand is low or generation from renewable sources is abundant and discharging it when demand is high or renewable generation is insufficient. Control systems are responsible for managing the flow of energy between the grid, turbines, and storage systems, ensuring that energy is used efficiently and that the grid remains stable. By dynamically balancing energy generation and storage, control systems play a crucial role in enabling the greater penetration of renewable energy while maintaining grid reliability.
In the realm of efficiency and emissions control, modern control and monitoring systems are crucial for optimizing steam turbine performance and reducing fuel consumption. These systems can monitor fuel combustion in real time and make adjustments to ensure that turbines operate at peak efficiency. For example, if the combustion process is not optimized, control systems can adjust parameters like air-fuel ratio, burner configuration, or combustion temperature to reduce fuel consumption and lower emissions. By maintaining efficient combustion and minimizing heat losses, these systems help to reduce operational costs and minimize the environmental footprint of the plant.
One of the primary advantages of these advanced systems is the ability to optimize the entire power generation process, not just individual components like turbines. By integrating data from boilers, condensers, turbines, energy storage, and even grid demand forecasts, control systems can improve the overall efficiency of the plant. For example, control systems can dynamically adjust steam flow to turbines based on real-time demand or emissions targets, reducing energy waste and improving both efficiency and cost-effectiveness. Additionally, these systems enable the integration of more flexible and efficient fuel sources, such as natural gas, biofuels, or hydrogen, into steam turbine operations, further enhancing the sustainability and reliability of power generation.
The future of control and monitoring systems in power plants lies in continued innovation, with advances in artificial intelligence, predictive analytics, and digital twins offering new opportunities for performance optimization, energy management, and sustainability. The continued advancement of these technologies promises a more automated, responsive, and intelligent approach to managing power generation systems. By integrating real-time data analysis, machine learning, and predictive capabilities, operators can make more informed decisions and ensure that turbines and other power generation assets operate efficiently and reliably. The role of control and monitoring systems will continue to grow as power plants evolve to meet the challenges of a changing energy landscape, including the need for greater flexibility, integration of renewable energy, and a more sustainable future.
Another area where control and monitoring systems are expected to make a significant impact is in grid optimization. As the demand for electricity increases and more distributed energy resources come online, power grids must become smarter to balance supply and demand more effectively. Advanced control systems are crucial for enabling real-time grid balancing by automatically adjusting energy generation and storage. These systems can monitor the state of the grid, adjust turbine outputs, and manage energy storage systems to ensure that supply matches demand, even during peak usage or when renewable generation is low. By enhancing grid stability, these systems can improve the resilience of energy systems and reduce the likelihood of outages.
In conclusion, the ongoing evolution of control and monitoring systems is reshaping how power plants operate and manage their assets. With the integration of advanced digital technologies, machine learning, predictive maintenance, and real-time monitoring, these systems provide a powerful tool for improving turbine performance, enhancing efficiency, reducing costs, and promoting sustainability. As the global energy landscape transitions toward more renewable and decentralized sources of power, the role of these systems in optimizing power generation, maintaining grid stability, and ensuring operational flexibility will become increasingly vital. Moving forward, these systems will continue to be at the forefront of innovation in the energy sector, driving the transition to cleaner, more efficient, and more reliable energy generation.
As we continue to see innovations in control and monitoring systems, the adoption of advanced technologies like blockchain for decentralized energy management, augmented reality (AR) for maintenance, and the increased role of artificial intelligence (AI) will further reshape the future of power generation. One of the exciting areas of development is the integration of blockchain technology in energy systems. Blockchain can enable secure and transparent transactions of energy within microgrids or between distributed energy resources (DERs) and the main grid. For instance, when power is generated from a renewable source, blockchain could be used to track and verify the energy production, ensuring that it is fairly distributed and accounted for across the network. This can be particularly valuable in peer-to-peer energy trading platforms where consumers can buy and sell energy from each other. Control systems, empowered by blockchain, will ensure that these transactions are recorded in real-time and the data is immutable, creating a highly transparent and secure method of managing distributed energy.
On the maintenance front, augmented reality (AR) is being explored as a tool to enhance the effectiveness and efficiency of turbine inspections and repairs. With AR, operators and technicians can overlay digital information onto physical turbines, enabling them to visualize performance data, maintenance logs, and even step-by-step repair instructions in real-time. This can reduce the time needed for troubleshooting, decrease the risk of human error, and improve overall plant safety. Technicians can access remote assistance through AR, allowing experts to guide them through complex repairs or adjustments without being physically present. By providing detailed, contextual information directly to the technician’s field of view, AR empowers operators to address issues more quickly and accurately.
Further pushing the boundaries of automation and optimization, AI and machine learning will increasingly be deployed in control and monitoring systems to enable adaptive, self-learning plants. Traditional power generation systems rely on pre-programmed rules and manual adjustments to optimize performance. However, AI-powered systems have the capability to learn from historical data and adjust operations in real-time, based on the plant’s current state. For example, machine learning algorithms can predict when a turbine might experience efficiency losses or when a part is likely to fail, allowing the system to automatically adjust turbine operations to avoid these issues before they become major problems. These systems can continuously refine their predictive models as more data is collected, resulting in ever-improving performance, efficiency, and reliability.
These AI-driven systems will also facilitate more precise energy dispatch, balancing power generation with fluctuating demand. During periods of high demand or low renewable energy generation, the control system can analyze grid conditions and adjust turbine output accordingly. Conversely, during periods of low demand or high renewable generation, turbines can be dialed down to minimize fuel consumption and reduce emissions. By doing so, the system can optimize fuel use and lower operational costs while maintaining grid stability.
Another emerging trend is the increasing use of digital simulation and modeling techniques to support decision-making. With the integration of real-time data from turbines and other power generation components, digital simulation tools can model the potential effects of different operating conditions before they are implemented. For instance, an operator could use a simulation tool to evaluate how different steam pressure levels or turbine configurations would affect overall plant performance and efficiency. These simulations help identify the most effective operating strategies and allow for better-informed decision-making. By testing different scenarios virtually, operators can avoid costly errors, reduce system downtime, and fine-tune performance.
Moreover, in the context of regulatory compliance and sustainability, these advanced control and monitoring systems can automatically collect and report emissions data, ensuring that the plant remains compliant with environmental regulations. They can also facilitate the use of carbon capture and storage (CCS) technologies by managing the integration of these systems with turbines and other plant components. As governments and regulatory bodies continue to place more stringent emissions targets on power plants, these systems will help operators monitor and reduce their carbon footprint more effectively. Control systems will be able to adjust turbine operations to align with emissions reduction goals, thus supporting the transition to greener, low-carbon energy.
As the energy landscape evolves, there is also a growing trend toward hybrid systems that combine various energy sources, such as renewable generation, natural gas, and energy storage. These hybrid systems require advanced control and monitoring solutions that can balance multiple inputs and outputs. For example, if wind or solar generation is low, the control system may automatically call upon natural gas turbines or energy storage to fill the gap, ensuring a continuous and stable power supply. These flexible, hybrid systems will enable power plants to integrate renewable energy more effectively while maintaining reliability, making them essential for the transition toward more sustainable energy production.
Another important development is the increasing importance of data integration across the energy value chain. In the past, turbines and other equipment were often operated in silos, with little integration between the various components of a power plant. Today, however, there is a growing trend toward integrating data from turbines, boilers, steam generators, and even grid operators into a unified system. This integration allows for better coordination and more informed decision-making, as operators can assess how each part of the plant is performing in relation to others. For example, a drop in turbine performance could trigger an investigation into the boiler’s efficiency, helping to pinpoint and resolve inefficiencies across the entire system.
Energy management systems (EMS) are also becoming a key part of the broader control and monitoring ecosystem. These systems oversee the operation of all energy assets, including turbines, batteries, and grid connections, to ensure that power generation is optimized in line with real-time demand. EMS platforms can respond dynamically to changes in the grid, making adjustments to turbine output, battery charging, or power exports based on changing conditions. In turn, this reduces energy waste, improves efficiency, and minimizes costs while ensuring that the plant remains flexible enough to respond to sudden fluctuations in grid demand or renewable generation.
In the future, as more power plants move toward energy decentralization and edge computing, we will see an increasing reliance on local control and monitoring systems that are capable of making decisions on-site without waiting for commands from a central control room. These edge-based systems, in combination with advanced AI algorithms, will allow plants to respond more quickly to local conditions, enhancing efficiency and grid resilience. Moreover, these decentralized systems can share insights with other plants or microgrids, fostering greater collaboration across the energy network and contributing to the creation of more resilient, sustainable, and flexible energy systems.
To sum up, the next generation of control and monitoring systems will continue to revolutionize steam turbine operation, power generation, and energy management as a whole. With the increasing integration of digital technologies, data analytics, and artificial intelligence, these systems will enable power plants to operate more efficiently, flexibly, and sustainably. The evolving landscape of energy production, with the rise of renewable energy, decentralized grids, and hybrid systems, will further highlight the importance of sophisticated control systems in optimizing performance, reducing emissions, and ensuring the reliability of the power supply. Ultimately, control and monitoring systems will play a central role in shaping the future of energy production and the transition to a cleaner, more sustainable energy grid.
Reheat Steam Turbines
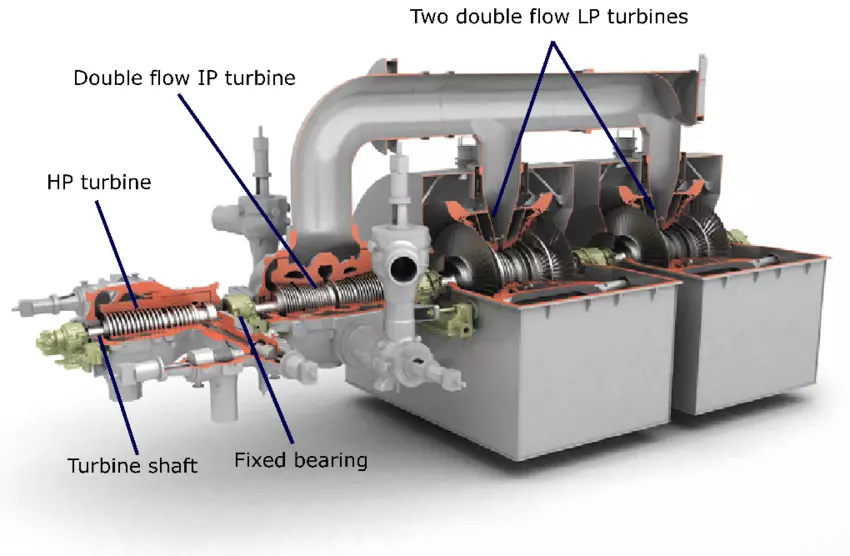
Reheat steam turbines are a type of steam turbine used in power generation, commonly found in thermal power plants where efficiency and performance are of critical importance. These turbines are designed to operate with a reheat cycle, a process where steam is passed through the turbine, then returned to the boiler to be reheated before it enters the turbine again. This reheat process improves the overall efficiency of the turbine and allows for better utilization of thermal energy.
In a conventional steam cycle, steam is produced in a boiler, expanded through the turbine, and then condensed in a condenser. However, by reheating the steam after its initial expansion, the turbine’s efficiency is significantly increased. The reheat process involves extracting the steam from the intermediate stage of the turbine, sending it back to the boiler, where it is heated again to a higher temperature. The steam is then reintroduced into the turbine at a later stage for further expansion, which helps increase the overall energy conversion efficiency by reducing the moisture content of the steam in the final stages of expansion. This moisture reduction helps to avoid damage to the turbine blades, which could occur if the blades were exposed to wet steam.
The benefits of reheat steam turbines lie primarily in their ability to achieve higher thermal efficiency and lower fuel consumption compared to traditional, single-stage turbines. By employing a reheat cycle, the thermal efficiency of the entire steam cycle can be increased, reducing the amount of fuel needed to produce the same amount of electricity. This translates to reduced operating costs and lower emissions, making reheat steam turbines a more environmentally friendly option for power generation.
The operation of a reheat steam turbine relies on careful coordination between the boiler, turbine, and condenser to maintain the desired pressure and temperature levels throughout the cycle. After the steam is reheated, it is expanded through the turbine’s intermediate or final stages, and the energy extracted from the steam is converted into mechanical power to drive the generator. The turbine operates in a manner that allows it to handle the high temperatures and pressures of the reheat steam, which requires high-quality materials and precise engineering to ensure reliable operation.
The reheat steam turbine’s ability to operate efficiently under a wide range of conditions makes it an attractive choice for many types of power plants, including coal-fired, natural gas, and nuclear power plants. In these plants, reheat turbines are often used to optimize the performance of the cycle, especially when operating in tandem with superheaters and reheaters in the boiler. The additional heat provided by the reheat process allows the plant to operate at higher efficiency, especially under varying load conditions, ensuring that more energy is extracted from the fuel and converted into usable electrical energy.
In terms of construction, reheat steam turbines are typically built with multiple stages. The steam enters the turbine at a high pressure and temperature, expands through the first stage, and is then extracted for reheating. After reheating, the steam enters a second stage of expansion in the turbine. This multiple-stage expansion process enables the turbine to extract more energy from the steam, improving its efficiency. Each stage of expansion in the turbine is designed to handle a specific pressure and temperature range, with high-grade materials being used to withstand the stresses and high temperatures associated with reheat cycles.
Reheat steam turbines also require advanced control systems to regulate the flow of steam, maintain the pressure and temperature at various points in the cycle, and ensure the safe and efficient operation of the turbine. These control systems are critical for ensuring that the reheat process is performed optimally, and that the system can adjust to changes in demand or operating conditions. Modern control systems are capable of performing real-time diagnostics, monitoring the condition of the turbine, and adjusting parameters like steam flow, pressure, and temperature to maintain maximum efficiency.
Despite the advantages in efficiency, reheat steam turbines also present some challenges. One of the main drawbacks is the complexity of the system, as it requires additional components such as reheaters and specialized piping to return steam to the boiler. This adds to the initial cost of the system, as well as to the complexity of operation and maintenance. The additional infrastructure needed for reheat cycles also increases the size of the plant and the space required for installation. Furthermore, while reheat steam turbines offer improved efficiency, their higher operating temperatures and pressures can result in greater wear and tear on the turbine components over time. This can lead to higher maintenance costs and the need for more frequent inspections to ensure that the turbine is operating optimally.
The application of reheat steam turbines in power generation plants plays a significant role in the quest for higher efficiency and lower environmental impact in the power industry. By improving the efficiency of the steam cycle and reducing fuel consumption, reheat turbines contribute to a more sustainable energy future. In particular, they help mitigate the environmental impact of fossil-fuel-based power generation by reducing the amount of carbon dioxide and other pollutants released into the atmosphere.
In recent years, advancements in material science and engineering have helped to address some of the challenges associated with reheat steam turbines. Newer materials that can withstand higher temperatures and pressures are allowing for greater efficiencies and longer operational lifespans. For example, the development of advanced alloys and coatings has improved the performance and durability of turbine blades, enabling them to withstand the harsh conditions in reheat cycles for longer periods. Additionally, improvements in control and monitoring systems have enabled more precise regulation of steam temperature, pressure, and flow, leading to better performance and reduced wear on the turbine components.
Reheat steam turbines are increasingly being considered as part of integrated combined-cycle power plants, where both gas and steam turbines are used together to maximize efficiency. In these systems, the gas turbine generates electricity by burning natural gas, and the exhaust heat from the gas turbine is used to produce steam, which then powers the steam turbine. The reheat steam turbine takes advantage of this additional heat to further improve efficiency, making combined-cycle systems some of the most efficient power plants in operation today. By combining the high efficiency of the reheat cycle with the added energy recovery from the gas turbine exhaust, these systems can achieve efficiencies that surpass those of conventional power plants.
In conclusion, reheat steam turbines represent a vital technology in modern power generation, offering significant improvements in thermal efficiency and fuel consumption. Through the reheat process, these turbines allow for more energy to be extracted from the steam, reducing operating costs and emissions. Though they require more complex systems and higher initial investment, their long-term benefits in efficiency and environmental impact make them a key solution for sustainable power generation. As technology continues to advance, reheat steam turbines will remain a crucial component of energy systems that prioritize efficiency, flexibility, and sustainability in power generation.
Reheat steam turbines have a crucial role to play in the evolving landscape of power generation, where the demand for higher efficiency and lower emissions is growing. As energy generation increasingly shifts toward renewable sources like wind and solar, the flexibility and efficiency of steam turbines, particularly reheat models, will become even more valuable. These turbines are not only essential in thermal power plants but are also gaining prominence in hybrid power generation systems that combine renewable energy sources with traditional steam-based systems. This hybridization allows for the optimization of the energy mix, ensuring a continuous and reliable power supply even when renewable energy sources are intermittent.
The ability of reheat steam turbines to operate efficiently in variable conditions is one of their key strengths. Modern reheat turbines are designed with greater adaptability, enabling them to perform well under different load conditions. This is especially important in a power grid where the integration of variable renewable energy sources often causes fluctuations in demand. Reheat steam turbines, with their advanced control systems, can adjust output smoothly, providing reliable backup power when renewable generation is insufficient, thus maintaining grid stability. In these settings, reheat turbines act as valuable assets that complement renewable energy sources, ensuring that the overall energy mix is optimized for both performance and sustainability.
Moreover, the trend toward more decentralized power generation systems is increasing the demand for advanced, efficient steam turbines like those with reheat cycles. Decentralized power plants, which are often smaller and more localized than traditional central power stations, benefit from the improved efficiency of reheat steam turbines. These plants can take advantage of the higher thermal efficiency of reheat turbines to generate more electricity from a given amount of fuel, thereby reducing their operational costs and environmental impact. The deployment of reheat turbines in smaller-scale, combined heat and power (CHP) systems also holds promise, particularly in industries that require both electricity and thermal energy, such as chemical processing and district heating.
The operational benefits of reheat steam turbines extend beyond just efficiency. These turbines are also integral to improving the overall reliability and lifespan of the power generation system. By reducing the moisture content in steam at the later stages of expansion, reheat turbines minimize the potential for corrosion and erosion of turbine blades, thus extending their operational life. This results in lower maintenance costs and fewer unplanned shutdowns, contributing to the overall economic efficiency of the plant.
In the context of energy transition, the use of reheat steam turbines is becoming increasingly relevant. As governments and industries continue to prioritize decarbonization, reheat turbines can help power plants operate more sustainably by maximizing the energy extracted from conventional fuels and reducing greenhouse gas emissions. In the long term, these turbines can play a role in supporting the transition to a lower-carbon future by improving the efficiency of power generation during the phase-out of fossil fuels and the ramp-up of cleaner energy sources.
In conclusion, reheat steam turbines remain a critical component in the energy sector, particularly in the context of higher efficiency and more sustainable energy production. The continued development of advanced turbine technology, including better materials and more efficient control systems, will only enhance the performance and reliability of these turbines. As power generation becomes more integrated with renewable energy sources, reheat steam turbines will continue to be key players in ensuring the stability, flexibility, and efficiency of the global energy grid.
The evolution of reheat steam turbines also aligns with the global shift towards improving the resilience and adaptability of energy systems. As energy production becomes more complex, with increased integration of renewable sources and more stringent environmental regulations, turbines must be able to operate in a way that maximizes performance under diverse conditions. The inclusion of reheat steam turbines in advanced power plants offers a significant advantage in this area, particularly in systems that must meet peak demand loads while maintaining efficiency and minimizing emissions.
One of the key advantages of reheat steam turbines is their ability to operate efficiently over a wide range of load conditions. Power plants often experience fluctuations in electricity demand due to changes in weather, time of day, or shifts in industrial activity. The ability of reheat steam turbines to handle such variations without compromising performance or efficiency makes them ideal for modern power generation systems. These turbines can adjust their output levels in response to changing grid conditions, allowing for a more flexible and responsive energy generation process. This operational flexibility is especially important in a grid that incorporates both renewable and conventional energy sources, as it helps to balance the supply and demand of electricity.
Additionally, reheat steam turbines offer the potential for increased combined heat and power (CHP) generation. In industries such as chemical processing, paper manufacturing, and district heating, CHP systems are used to generate both electricity and thermal energy for industrial processes or heating purposes. Reheat steam turbines are well-suited for CHP applications because they can extract more energy from the steam cycle. This enables higher efficiency in both electricity generation and thermal output, making them highly attractive for industrial operations seeking to maximize energy use while minimizing waste. By improving the efficiency of the steam cycle, reheat turbines contribute to the overall sustainability of these systems.
As the power generation sector transitions toward cleaner energy, reheat steam turbines are also playing a crucial role in reducing the carbon footprint of conventional power plants. By increasing the efficiency of steam-based generation, these turbines help reduce the amount of fuel required to produce electricity, which in turn lowers greenhouse gas emissions. Furthermore, the ability of reheat steam turbines to operate efficiently at lower fuel consumption levels directly contributes to lowering the operational costs of power plants, making them more competitive in an increasingly carbon-constrained world.
The materials used in the construction of reheat steam turbines are also evolving to meet the demands of modern, high-efficiency systems. Advances in metallurgy and materials science have led to the development of alloys and coatings that can withstand the high temperatures and pressures associated with reheat cycles. These materials are critical for ensuring the longevity and reliability of turbine components. For example, superalloys that are resistant to thermal fatigue, corrosion, and erosion are commonly used in the manufacture of turbine blades, allowing them to operate in the extreme conditions of reheat steam turbines without losing performance or durability over time.
Moreover, the integration of advanced monitoring and diagnostic technologies has made it easier to track the condition of reheat steam turbines and optimize their performance. Sensors embedded within the turbine and other critical components continuously monitor variables such as temperature, pressure, vibration, and steam flow. These sensors feed real-time data to control systems, which can analyze the performance of the turbine and make adjustments to optimize efficiency. For example, if the system detects that a certain component is experiencing wear or that the turbine is operating outside its optimal performance range, it can automatically make adjustments or send maintenance alerts to operators. This proactive approach to maintenance and optimization reduces downtime, minimizes unexpected failures, and extends the overall lifespan of the turbine.
Another exciting development in reheat steam turbine technology is the growing potential for digital twins. A digital twin is a virtual replica of a physical system, such as a turbine, that can be used to simulate and monitor its behavior in real-time. Through the use of sensors and data analytics, digital twins can provide detailed insights into the turbine’s performance, predict future issues, and suggest maintenance activities before problems arise. This technology is helping operators to improve efficiency, reduce operational costs, and enhance safety by providing a better understanding of the turbine’s behavior under different conditions. As digital twin technology continues to mature, it is expected to play a significant role in optimizing the operation of reheat steam turbines in the coming years.
Additionally, reheat steam turbines are increasingly being deployed in combined-cycle power plants, where both gas and steam turbines are used to generate electricity. In these plants, the gas turbine generates power by burning natural gas, while the exhaust heat from the gas turbine is used to produce steam for the steam turbine. The integration of reheat steam turbines in such systems enables even higher efficiency by extracting more energy from the steam. Combined-cycle plants with reheat steam turbines are some of the most efficient power plants in operation today, achieving efficiencies of over 60%. These plants can respond to fluctuations in electricity demand more effectively than conventional plants, providing a reliable source of power while minimizing emissions.
The use of reheat steam turbines is also becoming more prominent in the pursuit of cleaner energy technologies. For instance, in the development of carbon capture and storage (CCS) systems, reheat steam turbines play a key role by improving the overall efficiency of the power generation process, thus reducing the amount of CO2 emitted per unit of electricity produced. These systems are critical in the fight against climate change, as they help mitigate the environmental impact of fossil fuel-based power plants. As CCS technologies continue to evolve, reheat steam turbines are expected to be an essential part of these integrated solutions, enabling more efficient capture and storage of carbon dioxide.
As the power industry continues to evolve, the role of reheat steam turbines will become increasingly important. Their ability to operate at higher efficiencies, reduce emissions, and integrate into complex energy systems makes them a key technology in both conventional and emerging energy generation systems. With the continued advancements in turbine design, materials science, control systems, and digital technologies, reheat steam turbines will remain central to the global effort to produce cleaner, more efficient energy for the future.
In summary, reheat steam turbines are a vital technology in the modern power generation landscape. Their ability to increase the efficiency of the steam cycle, reduce fuel consumption, and improve the overall sustainability of power plants makes them a valuable asset in both traditional and emerging energy systems. As the energy sector continues to evolve, reheat steam turbines will continue to play an essential role in meeting the growing demand for clean, efficient, and reliable electricity.
As the power generation industry faces increasing pressure to reduce carbon emissions and improve energy efficiency, reheat steam turbines will continue to be a cornerstone of the energy mix. Their ability to harness the maximum potential of steam through the reheat cycle not only enhances performance but also aligns with the industry’s goals of minimizing environmental impact and reducing reliance on fossil fuels.
Looking toward the future, the development of reheat steam turbines is likely to be influenced by several key trends and innovations. One of the most significant of these is the ongoing push toward integrating renewable energy sources into the grid. The variable nature of renewable sources such as wind and solar power requires that traditional power plants, including those with reheat steam turbines, adapt to provide flexible, on-demand power generation. Reheat turbines, with their ability to efficiently handle a wide range of operational conditions, are ideally suited to meet the challenge of providing stable backup power when renewable energy generation is low. This capability will be crucial as countries strive to decarbonize their power sectors while maintaining grid stability and reliability.
In addition to integrating renewable energy, the demand for electrification in various sectors, such as transportation and heating, is growing. This electrification will place additional pressure on the grid, creating a need for power plants that can respond quickly and efficiently to fluctuations in demand. Reheat steam turbines, with their ability to adjust output levels based on changing demand, can help meet this increased load while maintaining optimal efficiency. This flexibility will make them a critical asset in grids that are becoming more dynamic due to the growing penetration of electric vehicles, heat pumps, and other electrified technologies.
As energy generation transitions to cleaner sources, reheat steam turbines may also play a role in hybrid plants that combine renewable energy with advanced thermal generation technologies. For example, concentrating solar power (CSP) plants that use mirrors or lenses to focus sunlight to generate heat can be integrated with reheat steam turbines. These plants can use the stored thermal energy from the sun to generate steam, which is then passed through the reheat cycle to maximize efficiency. Such hybrid solutions allow for continuous electricity generation even when the sun is not shining, providing a reliable source of clean energy that can be dispatched as needed.
Furthermore, the shift toward decarbonization is likely to drive ongoing research and development in materials science, turbine design, and digital technologies. New materials that can withstand even higher temperatures and pressures, such as advanced ceramics and superalloys, will enable reheat steam turbines to operate at even greater efficiencies. These materials, combined with improved coatings and turbine blade designs, will ensure that turbines remain durable and reliable under the extreme conditions of the reheat cycle.
Another key area of development is the incorporation of digital technologies, including artificial intelligence (AI) and machine learning, into the operation and maintenance of reheat steam turbines. AI algorithms can analyze vast amounts of data collected from sensors embedded in turbines, identifying patterns and predicting potential issues before they occur. For example, AI could detect early signs of turbine blade erosion or thermal stress, allowing operators to perform predictive maintenance and avoid costly downtime. As digital technologies become more integrated into power plant operations, the ability to optimize the performance of reheat steam turbines will become increasingly sophisticated, further enhancing their efficiency and reliability.
The integration of reheat steam turbines into district heating systems is another area where they are likely to see increased use. In many cities around the world, district heating networks provide heat to residential, commercial, and industrial buildings by using steam or hot water circulated through a network of pipes. Reheat steam turbines can be a valuable component in these systems, especially when combined with combined heat and power (CHP) technology. The efficiency of reheat turbines allows them to generate electricity while simultaneously providing heat to the district heating network. This dual functionality maximizes the use of energy, reducing waste and lowering operational costs for utilities.
As the global energy landscape evolves, reheat steam turbines will also be key players in the continued advancement of carbon capture, utilization, and storage (CCUS) technologies. Power plants equipped with reheat steam turbines will be better positioned to integrate carbon capture systems, as the increased efficiency of the steam cycle can offset the additional energy required for capturing and compressing CO2. By improving the overall efficiency of the power plant, reheat turbines make it easier to implement CCUS technologies without sacrificing performance. As governments implement stricter emissions regulations, the role of reheat steam turbines in helping to capture and store carbon will become increasingly important.
The push for greater efficiency and sustainability in the power generation sector will continue to drive innovation in reheat steam turbine technology. As the global energy industry faces the challenges of balancing growing demand with environmental concerns, reheat steam turbines will remain essential for meeting these needs. Their ability to improve the efficiency of thermal power plants, reduce fuel consumption, and lower emissions makes them a critical tool in the ongoing transition to cleaner, more efficient energy systems.
In conclusion, the future of reheat steam turbines looks promising, as they continue to evolve in response to the changing energy landscape. Their high efficiency, flexibility, and ability to integrate into advanced power generation systems make them a vital part of the energy mix. Whether through supporting the integration of renewable energy, enabling hybrid power systems, or contributing to the decarbonization of the power sector, reheat steam turbines will remain a cornerstone of sustainable and efficient energy production. As technology advances and new challenges emerge, these turbines will continue to play a crucial role in shaping the future of power generation.
EMS Power Machines
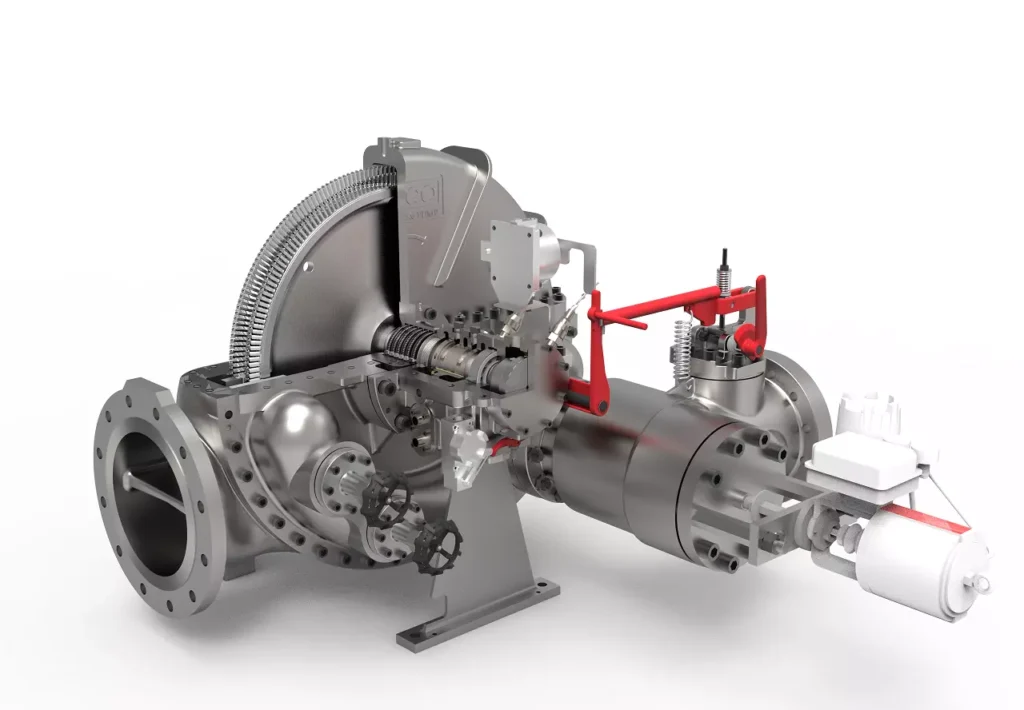
We design, manufacture and assembly Power Machines such as – diesel generators, electric motors, vibration motors, pumps, steam engines and steam turbines
EMS Power Machines is a global power engineering company, one of the five world leaders in the industry in terms of installed equipment. The companies included in the company have been operating in the energy market for more than 60 years.
EMS Power Machines manufactures steam turbines, gas turbines, hydroelectric turbines, generators, and other power equipment for thermal, nuclear, and hydroelectric power plants, as well as for various industries, transport, and marine energy.
EMS Power Machines is a major player in the global power industry, and its equipment is used in power plants all over the world. The company has a strong track record of innovation, and it is constantly developing new and improved technologies.
Here are some examples of Power Machines’ products and services:
- Steam turbines for thermal and nuclear power plants
- Gas turbines for combined cycle power plants and industrial applications
- Hydroelectric turbines for hydroelectric power plants
- Generators for all types of power plants
- Boilers for thermal power plants
- Condensers for thermal power plants
- Reheaters for thermal power plants
- Air preheaters for thermal power plants
- Feedwater pumps for thermal power plants
- Control systems for power plants
- Maintenance and repair services for power plants
EMS Power Machines is committed to providing its customers with high-quality products and services. The company has a strong reputation for reliability and innovation. Power Machines is a leading provider of power equipment and services, and it plays a vital role in the global power industry.
EMS Power Machines, which began in 1961 as a small factory of electric motors, has become a leading global supplier of electronic products for different segments. The search for excellence has resulted in the diversification of the business, adding to the electric motors products which provide from power generation to more efficient means of use.