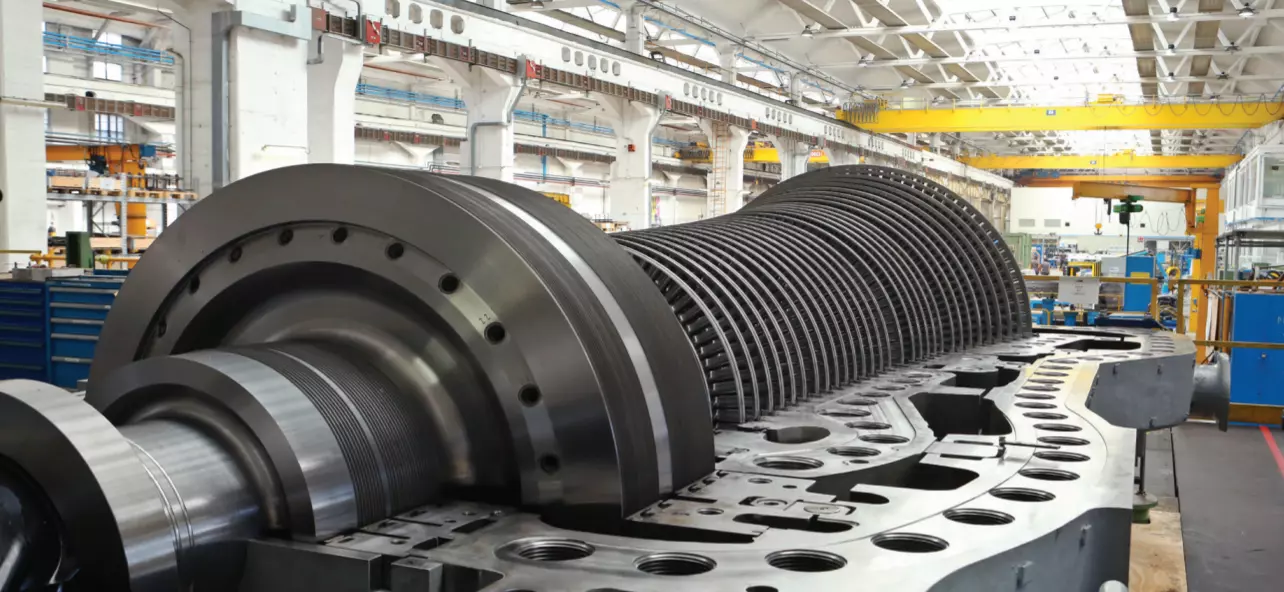
Back pressure steam turbine represents a fundamental element in power plants utilizing the Rankine cycle for electricity generation. This section provides an overview of the role of back pressure turbines in power systems, outlining their significance in converting thermal energy from steam into mechanical work.
- Thermodynamics of Back Pressure Steam Turbines:
A solid comprehension of thermodynamic principles underpinning the operation of back pressure steam turbines is crucial. This section delves into key concepts such as enthalpy, entropy, and the Rankine cycle, elucidating the interplay of pressure and temperature on turbine performance and efficiency.
- Design Considerations:
Designing efficient back pressure steam turbines requires a meticulous balance between aerodynamics, materials science, and mechanical engineering. This section explores the intricacies of blade profiles, steam path geometry, and rotor dynamics, emphasizing the critical role of these design elements in achieving optimal turbine efficiency.
- Operational Aspects:
Efficient operation of back pressure steam turbines demands careful consideration of various operational parameters. Topics covered include control mechanisms, governing systems, and the impact of variable steam conditions on turbine performance. Additionally, the role of extraction points and their influence on overall plant efficiency is discussed.
- Performance Optimization Strategies:
Optimizing the performance of back pressure steam turbines is essential for maximizing power plant efficiency. This section covers advanced strategies, including modifications to steam path geometry, the implementation of advanced control systems, and the integration of variable-speed drives. The impact of these strategies on overall efficiency and flexibility in responding to varying loads is examined.
- Advanced Technologies and Innovations:
The evolving landscape of power generation introduces new technologies and innovations in back pressure steam turbines. Concepts such as advanced materials, innovative blade designs, and the incorporation of digital control systems are explored. The potential impact of these advancements on turbine efficiency and reliability is discussed.
- Case Studies and Practical Applications:
Real-world case studies of successful back pressure steam turbine installations are presented, offering insights into challenges faced, solutions implemented, and lessons learned. These case studies serve as practical references for engineers and operators seeking to optimize the performance of existing installations or plan new projects.
- Future Trends and Challenges:
The text concludes with a discussion on emerging trends, challenges, and potential future developments in back pressure steam turbine technology. Topics such as the integration of advanced sensors for condition monitoring, the impact of Industry 4.0 on turbine operations, and the role of back pressure turbines in decentralized energy systems are explored.
In summary, this text provides a comprehensive exploration of back pressure steam turbines, offering a blend of theoretical foundations, practical insights, and a forward-looking perspective. Aimed at engineers, researchers, and students, this resource aims to deepen understanding of the complex dynamics involved in harnessing steam power through back pressure turbines for efficient electricity generation.
This comprehensive text delves into the intricacies of back pressure steam turbines, crucial components in power generation systems where the Rankine cycle is employed. Focusing on design principles, operational considerations, and strategies for optimizing performance, the following exposition serves as a valuable resource for engineers, researchers, and enthusiasts seeking an in-depth understanding of the nuanced dynamics associated with back pressure steam turbines.
- Introduction:
Back pressure steam turbines represent a fundamental element in power plants utilizing the Rankine cycle for electricity generation. This section provides an overview of the role of back pressure turbines in power systems, outlining their significance in converting thermal energy from steam into mechanical work.
- Thermodynamics of Back Pressure Steam Turbines:
A solid comprehension of thermodynamic principles underpinning the operation of back pressure steam turbines is crucial. This section delves into key concepts such as enthalpy, entropy, and the Rankine cycle, elucidating the interplay of pressure and temperature on turbine performance and efficiency.
- Design Considerations:
Designing efficient back pressure steam turbines requires a meticulous balance between aerodynamics, materials science, and mechanical engineering. This section explores the intricacies of blade profiles, steam path geometry, and rotor dynamics, emphasizing the critical role of these design elements in achieving optimal turbine efficiency.
- Operational Aspects:
Efficient operation of back pressure steam turbines demands careful consideration of various operational parameters. Topics covered include control mechanisms, governing systems, and the impact of variable steam conditions on turbine performance. Additionally, the role of extraction points and their influence on overall plant efficiency is discussed.
- Performance Optimization Strategies:
Optimizing the performance of back pressure steam turbines is essential for maximizing power plant efficiency. This section covers advanced strategies, including modifications to steam path geometry, the implementation of advanced control systems, and the integration of variable-speed drives. The impact of these strategies on overall efficiency and flexibility in responding to varying loads is examined.
- Advanced Technologies and Innovations:
The evolving landscape of power generation introduces new technologies and innovations in back pressure steam turbines. Concepts such as advanced materials, innovative blade designs, and the incorporation of digital control systems are explored. The potential impact of these advancements on turbine efficiency and reliability is discussed.
- Case Studies and Practical Applications:
Real-world case studies of successful back pressure steam turbine installations are presented, offering insights into challenges faced, solutions implemented, and lessons learned. These case studies serve as practical references for engineers and operators seeking to optimize the performance of existing installations or plan new projects.
- Future Trends and Challenges:
The text concludes with a discussion on emerging trends, challenges, and potential future developments in back pressure steam turbine technology. Topics such as the integration of advanced sensors for condition monitoring, the impact of Industry 4.0 on turbine operations, and the role of back pressure turbines in decentralized energy systems are explored.
In summary, this text provides a comprehensive exploration of back pressure steam turbines, offering a blend of theoretical foundations, practical insights, and a forward-looking perspective. Aimed at engineers, researchers, and students, this resource aims to deepen understanding of the complex dynamics involved in harnessing steam power through back pressure turbines for efficient electricity generation.
The Back-Pressure Steam Turbine: A Balancing Act of Power and Process
While its condensing cousin steals the spotlight in electricity generation, the back-pressure steam turbine (BPST) hums quietly in the background, playing a crucial role in powering industrial processes. Unlike the condensing turbine, whose primary objective is electricity production, the BPST dances to a different tune, prioritizing mechanical power extraction while cleverly utilizing the leftover thermal energy for industrial needs. Let’s peel back the layers of this fascinating machine and witness its intricate balance of power and process.
1. Steam’s Tailor-Made Journey: Adapting to Process Demands
Unlike the standardized high-pressure steam fed to condensing turbines, the BPST receives steam customized for specific industrial needs. The boiler, its fiery forge, tailors the steam’s pressure and temperature to suit the downstream process requirements. Whether it’s driving pumps in a petrochemical plant or powering compressors in a paper mill, the BPST receives steam tuned to the specific energy demands of the application.
2. Power Unveiled: A Waltz of Blades and Rotational Might
Just like its condensing counterpart, the BPST’s heart lies in the intricate ballet of steam and blades. Arranged in stages within the turbine casing, the blades pirouette with the incoming steam, extracting its kinetic energy through meticulously designed geometries. However, unlike the condensing turbine, the BPST’s blade design and stage configuration prioritize maximizing shaft power output while maintaining a specific exhaust pressure. This tailored approach ensures the extracted energy seamlessly integrates into the downstream process, driving equipment and powering industrial needs.
3. The Exhaust’s Encore: Thermal Energy’s Second Act
But the BPST’s brilliance goes beyond mere shaft power. Recognizing the remaining thermal potential in the exhaust steam, the turbine strategically maintains a higher-than-condensing pressure at the outlet. This residual heat becomes the star of the second act, providing valuable thermal energy for industrial applications. From preheating boiler feedwater to supplying process steam for heating or distillation, the BPST’s exhaust finds a new purpose, maximizing overall energy utilization.
4. Efficiency’s Double Play: Balancing Power and Process
The BPST’s true mastery lies in its delicate balancing act. It optimizes mechanical power extraction for the immediate process needs while simultaneously preserving precious thermal energy for further utilization. This intricate dance between immediate power and future thermal benefit distinguishes the BPST from its condensing counterpart, making it the unsung hero of industrial energy efficiency.
5. A Spectrum of Applications: Where the BPST Shines
The BPST’s versatility finds application across a vast spectrum of industries. From the behemoths of oil and gas processing to the intricate operations of paper and textile mills, the BPST seamlessly integrates into diverse production chains. Its ability to tailor power output and provide valuable thermal energy makes it a crucial cog in the machinery of modern industrial processes.
Beyond the Technical Spotlight: Challenges and Advancements
Despite its efficiency prowess, the BPST faces its own challenges. Optimizing blade design and stage configurations for specific processes requires careful engineering considerations. Additionally, ensuring stable operation while handling exhaust steam at varying pressures necessitates advanced control systems. However, continuous advancements in materials science, thermodynamics modeling, and control technology are constantly pushing the boundaries of BPST efficiency and operational flexibility.
The back-pressure steam turbine might not bask in the same limelight as its electricity-generating cousin, but its intricate dance of power and process plays a vital role in powering the wheels of modern industry. Its ability to seamlessly integrate into diverse applications, while maximizing energy utilization, makes it a true testament to engineering ingenuity and a champion of industrial efficiency. So, the next time you encounter a humming factory or a bustling refinery, remember the silent maestro pulling the strings – the back-pressure steam turbine, a master of balance in the grand symphony of industrial progress.
Back Pressure Steam Turbine Characteristics
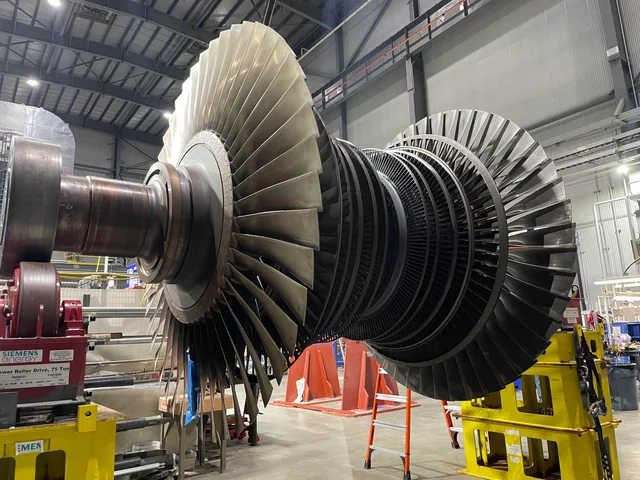
Governor Valve:
The governor valve is a crucial component in the control system of steam turbines, designed to regulate the flow of steam entering the turbine. Its primary function is to maintain a constant rotational speed of the turbine, ensuring a stable and predictable output of mechanical and electrical power.
In a back pressure steam turbine system, the governor valve responds to changes in load or demand for electricity. If there is an increase in demand, the governor valve opens to allow more steam into the turbine, facilitating higher power output. Conversely, if the demand decreases, the governor valve adjusts to reduce steam flow, maintaining a consistent turbine speed.
The governor valve operates based on a feedback loop that includes speed sensors, controllers, and actuators. As the turbine speed deviates from the setpoint, the governor valve adjusts to bring the speed back to the desired level. This dynamic control mechanism is essential for grid stability, ensuring that the turbine responds promptly to variations in electrical demand.
Advanced governor systems utilize digital controls and sophisticated algorithms to optimize turbine performance under varying conditions. The effectiveness of the governor valve directly impacts the overall efficiency and reliability of the back pressure steam turbine, making it a critical element in power generation systems.
Thermodynamics:
Thermodynamics is a branch of physics that deals with the principles governing the relationships between heat, work, temperature, and energy. In the context of back pressure steam turbines, a deep understanding of thermodynamics is essential for analyzing and optimizing the performance of these energy conversion systems.
The first law of thermodynamics, also known as the law of energy conservation, states that energy cannot be created or destroyed but can only change forms. In a back pressure steam turbine, this law is fundamental as it governs the conversion of thermal energy from high-pressure steam into mechanical work and subsequently into electrical power.
The second law of thermodynamics introduces the concept of entropy, which measures the degree of disorder or randomness in a system. In steam turbines, maintaining low entropy during the expansion of steam is crucial for maximizing the efficiency of the energy conversion process.
The isentropic process, where the entropy remains constant, is often used as an idealized model for steam expansion in turbines. However, real-world processes involve irreversibilities, and understanding these thermodynamic inefficiencies is crucial for practical turbine design and operation.
Thermodynamics also plays a role in the selection of working fluids, the design of heat exchangers, and the overall efficiency analysis of power cycles. Engineers use thermodynamic principles to optimize the performance of back pressure steam turbines, ensuring that they operate within specified parameters for maximum energy conversion efficiency and reliability.
Isentropic Efficiency:
Isentropic efficiency is a key parameter in the performance assessment of steam turbines, providing a measure of how efficiently a turbine converts the enthalpy of steam into mechanical work under idealized, reversible conditions.
Defined as the ratio of actual work output to the maximum work output possible in an isentropic (constant entropy) process, isentropic efficiency is expressed mathematically as the ratio of the change in enthalpy (h) to the isentropic change in enthalpy (h_s):
Isentropic Efficiency(isentropic)=ℎ1−ℎ2ℎ1−ℎ2Isentropic Efficiency(ηisentropic)=h1−h2sh1−h2
where:
- h1 is the enthalpy of the steam at the turbine inlet,
- h2 is the actual enthalpy of the steam at the turbine exit, and
- h2s is the enthalpy of the steam at the turbine exit under isentropic conditions.
In practical terms, isentropic efficiency provides insights into how well a steam turbine is performing compared to an idealized, frictionless, and reversible process. Actual processes involve inherent irreversibilities, such as friction, heat losses, and turbulence, which reduce the efficiency from the ideal isentropic case.
Engineers use isentropic efficiency as a critical parameter in the design and analysis of steam turbines, aiming to improve overall efficiency and energy conversion performance. Monitoring and optimizing isentropic efficiency help ensure that the turbine operates at its highest potential while considering real-world thermodynamic limitations.
Condenser:
A condenser is a vital component in steam power plants, including those utilizing back pressure steam turbines, designed to enhance the efficiency of the overall thermodynamic cycle by converting steam back into water.
After steam passes through the turbine blades, it enters the condenser, where it is exposed to a cooling medium, often water. The heat from the steam is transferred to the cooling medium, causing the steam to undergo a phase change from vapor to liquid. This condensed water is then pumped back into the steam cycle, completing the water-steam-water cycle.
The primary purpose of the condenser is to maintain a low-pressure environment at the turbine exhaust. This low pressure allows the steam to expand more fully in the turbine, extracting as much energy as possible. The efficiency of the condensation process is crucial for overall power plant efficiency.
Various types of condensers exist, such as surface condensers and direct-contact condensers, each with its advantages and disadvantages. Surface condensers use a series of tubes to facilitate heat exchange between steam and a cooling medium, while direct-contact condensers allow steam to come into direct contact with the cooling water.
The performance of the condenser significantly influences the efficiency of the steam cycle, making it a critical element in the design and operation of power plants using back pressure steam turbines.
Inlet Temperature:
In the context of back pressure steam turbines, the inlet temperature refers to the temperature of the steam entering the turbine. This parameter is a crucial factor influencing the efficiency and overall performance of the turbine.
The efficiency of a steam turbine is closely tied to the temperature difference between the steam at the inlet and the condenser pressure. Higher inlet temperatures generally lead to higher thermal efficiency, as the available temperature drop across the turbine is increased.
However, there are practical limits to the inlet temperature, primarily dictated by the materials used in turbine components. Higher temperatures can lead to increased stress and wear on turbine blades and other components. Advanced materials and cooling techniques are employed to push these temperature limits and improve overall efficiency.
The relationship between steam temperature and turbine efficiency is a key consideration in the design and operation of back pressure steam turbines. Engineers carefully balance the benefits of higher temperatures for increased efficiency with the challenges associated with material limitations and the potential for decreased turbine lifespan. Understanding and optimizing the inlet temperature contribute to achieving the desired performance and efficiency in power generation systems.
Blade Erosion:
Blade erosion is a significant concern in the operation of steam turbines, including back pressure steam turbines, and refers to the gradual wear and damage experienced by turbine blades over time. This erosion is primarily attributed to the impact of steam containing entrained solid particles, such as small water droplets or particulate matter.
As steam passes through the turbine stages, especially at high velocities, it can carry tiny solid particles. These particles impinge on the turbine blades, causing erosion and gradual material loss. Blade erosion is a complex phenomenon influenced by factors like steam quality, steam velocity, and the characteristics of the entrained particles.
The erosion process can result in changes to the blade’s surface geometry, leading to efficiency losses and potentially compromising the structural integrity of the turbine components. Engineers employ various strategies to mitigate blade erosion, including optimizing steam quality, employing advanced blade materials with enhanced erosion resistance, and implementing effective steam filtering systems.
Regular maintenance, inspections, and monitoring of erosion rates are essential to ensure the continued reliable and efficient operation of back pressure steam turbines. Understanding and addressing blade erosion contribute to extending the operational life and maintaining the performance of steam turbines in power generation and industrial applications.
Cogeneration Efficiency:
Cogeneration efficiency is a key metric in evaluating the performance of cogeneration or combined heat and power (CHP) systems, where both electrical and thermal energy are simultaneously produced from a single energy source, often involving back pressure steam turbines.
The efficiency of cogeneration is calculated by considering the total useful energy output (both electricity and thermal energy) compared to the energy input from the primary fuel source. The formula for cogeneration efficiency is:
Cogeneration Efficiency=Total Useful Energy OutputTotal Energy InputCogeneration Efficiency=Total Energy InputTotal Useful Energy Output
In the context of a back pressure steam turbine, this includes accounting for the electrical power generated and the thermal energy extracted from the steam for heating or industrial processes.
High cogeneration efficiency is desirable as it indicates effective utilization of the available energy, reducing waste and improving overall system sustainability. Cogeneration systems offer significant advantages in energy efficiency compared to separate production of electricity and thermal energy. Optimizing the cogeneration efficiency involves balancing the electrical and thermal loads, selecting appropriate equipment, and maximizing the use of both energy outputs to meet the demands of the end-users. This approach contributes to a more efficient and sustainable energy utilization strategy.
Steam Header:
A steam header is a vital component in steam power plants and industrial facilities utilizing back pressure steam turbines. It serves as a manifold that collects and distributes steam from multiple sources to various parts of the plant or industrial processes.
Steam produced in different sections of the plant, such as boilers or heat recovery steam generators, is directed to the steam header. The steam header acts as a distribution point, ensuring a steady and controlled supply of steam to different users or processes. It plays a critical role in balancing steam distribution and maintaining uniform pressure across the system.
The design of a steam header involves considerations of pressure, temperature, and flow rate, ensuring that steam is delivered efficiently and safely to the intended destinations. Valves and control systems may be integrated into the steam header to regulate steam flow and pressure, contributing to the overall stability and reliability of the steam supply.
Proper maintenance and monitoring of the steam header are essential to prevent issues such as steam leakage, uneven distribution, or pressure fluctuations. The effective operation of the steam header optimizes the performance of back pressure steam turbines and supports various industrial processes requiring a reliable source of steam.
Steam Quality:
Steam quality is a crucial parameter in the operation of steam turbines, including back pressure steam turbines. It refers to the dryness fraction or the percentage of vapor in a steam-water mixture. Steam quality is a key factor influencing the efficiency and reliability of the energy conversion process in steam turbines.
In an ideal scenario, steam would be perfectly dry, meaning it contains 100% vapor with no liquid water. However, in real-world conditions, steam often contains some water droplets or moisture. The presence of moisture can impact the performance of steam turbines, leading to issues such as erosion of turbine blades and reduced efficiency.
Steam quality is typically expressed as a percentage, with higher percentages indicating drier steam. Engineers carefully control and monitor steam quality in power plants by employing separators, moisture removal systems, and ensuring proper steam generation processes. Effective steam quality management helps minimize the potential for erosion and ensures that the energy in the steam is efficiently converted into mechanical work by the turbine.
Understanding and maintaining optimal steam quality are essential for the reliable and efficient operation of back pressure steam turbines, contributing to the longevity and performance of the turbine components.
Reheat Steam Turbine:
A reheat steam turbine is a specialized type of steam turbine commonly used in power plants to enhance the overall efficiency of the thermodynamic cycle. Unlike simple back pressure turbines, reheat turbines incorporate a reheat process, which involves reheating the steam before it undergoes additional expansion in the turbine.
In a reheat steam turbine system, high-pressure steam from the boiler is first expanded through the initial stages of the turbine, producing mechanical work. Instead of allowing the steam to continue its expansion through the remaining turbine stages, it is extracted and sent back to the boiler for reheating. The reheated steam is then directed into the subsequent stages of the turbine for further expansion and work production.
The inclusion of the reheat process allows the steam to expand over a larger temperature range, increasing the overall efficiency of the turbine. This is particularly beneficial in power plants where high thermal efficiency is a priority. Reheat turbines are commonly used in large-scale power generation facilities and are often associated with combined cycle power plants that integrate both gas and steam turbines.
The design and operation of reheat steam turbines require careful consideration of temperature and pressure conditions to optimize efficiency and power output while ensuring the reliability of turbine components.
Combined Cycle Power Plant:
A combined cycle power plant is a sophisticated energy generation facility that integrates both gas turbines and steam turbines to maximize overall efficiency in electricity production. This type of power plant is commonly employed to extract the most energy possible from fuel sources, making it an efficient and environmentally friendly option.
In a combined cycle power plant, the process begins with a gas turbine that burns fuel, typically natural gas. The gas turbine produces mechanical power by driving a generator, generating electricity. The hot exhaust gases from the gas turbine, which still contain significant thermal energy, are then directed to a heat recovery steam generator (HRSG).
The HRSG captures the residual heat from the gas turbine exhaust and uses it to produce high-pressure steam. This steam is then directed into a steam turbine, such as a back pressure turbine, where it undergoes additional expansion, generating additional mechanical power to drive a second generator.
The combination of the gas turbine and steam turbine in a single power plant creates a synergistic effect, significantly improving overall efficiency compared to separate power generation systems. Combined cycle power plants are known for their high efficiency, reduced environmental impact, and versatility in various applications, ranging from large-scale utility plants to industrial facilities.
Back Pressure Valve:
A back pressure valve is a crucial component in steam systems, particularly those utilizing back pressure steam turbines. Its primary function is to control and maintain the pressure of steam within the desired range at the turbine outlet, influencing both power generation and the extraction of thermal energy for other processes.
In a back pressure steam turbine system, steam is allowed to expand through the turbine, producing mechanical work and generating electricity. The back pressure valve, located at the turbine exhaust, regulates the pressure of the steam before it is released to the condenser or other downstream processes.
The operation of the back pressure valve is essential for controlling the overall system pressure, which directly impacts the efficiency and performance of the steam turbine. By adjusting the opening of the valve, operators can control the pressure at the turbine outlet and, consequently, the power output of the turbine. Properly configured back pressure valves contribute to stable turbine operation and optimal energy conversion.
Additionally, back pressure valves play a role in managing the extraction of low-pressure steam for heating or industrial applications. This dual functionality enhances the versatility of back pressure steam turbines in applications where both electricity generation and thermal energy utilization are essential. Engineers carefully design and maintain back pressure valves to ensure efficient and reliable operation in steam power systems.
Steam Turbine Rotor:
The steam turbine rotor is a central and critical component in the operation of steam turbines, including back pressure steam turbines. It is the rotating element that receives the mechanical energy from the expanding steam and transfers it to the generator for the production of electrical power.
In a back pressure steam turbine, high-pressure steam is admitted into the turbine, causing the rotor to spin. The rotor is typically a shaft with multiple blades or buckets mounted on it. As the steam expands through the turbine blades, the rotor experiences a torque that results in rotational motion.
The design of the steam turbine rotor is a complex engineering task, considering factors such as material strength, aerodynamics, and dynamic balancing. The rotational speed of the rotor is a critical parameter, and it must be carefully controlled to maintain stable and efficient turbine operation.
To achieve the highest efficiency and reliability, modern steam turbine rotors are often designed with advanced materials and technologies. Additionally, precise manufacturing and assembly processes, along with continuous monitoring, are employed to ensure the integrity and performance of the steam turbine rotor throughout its operational life. The efficiency and overall success of the back pressure steam turbine system heavily depend on the design and maintenance of this essential rotating component.
Extraction Steam:
Extraction steam in the context of steam turbines refers to the controlled withdrawal of steam at intermediate stages of the turbine for specific industrial processes or heating applications. This process enhances the versatility and efficiency of back pressure steam turbines, allowing them to serve multiple purposes beyond electricity generation.
In a back pressure steam turbine system, high-pressure steam is admitted into the turbine to produce mechanical work and generate electricity. However, before the steam completes its expansion through all the turbine stages, a portion of it can be extracted for various purposes. This extracted steam, known as extraction steam, is redirected to other parts of the industrial process or utilized for district heating, enhancing the overall energy efficiency of the system.
Extraction steam is commonly used in industries where both electricity and thermal energy are required. The extraction process allows for the simultaneous production of electricity and the utilization of steam for heating applications or industrial processes. Careful control of extraction steam is essential to balance the energy requirements of different processes and maintain optimal efficiency in the overall system. Engineers design and optimize extraction systems based on specific operational needs, contributing to the flexibility and efficiency of back pressure steam turbines in various applications.
Condensate Pump:
A condensate pump is a critical component in steam power plants, especially those employing back pressure steam turbines. Its primary function is to move the condensed water, known as condensate, from the condenser back into the steam cycle, completing the water-steam-water cycle and ensuring efficient reuse of water within the system.
After steam passes through the turbine and undergoes condensation in the condenser, the resulting condensate is collected at the bottom of the condenser. The condensate pump then draws this water and pumps it back to the boiler or heat exchanger, where it is heated to become steam once again.
Efficient condensate removal is vital for maintaining a continuous and reliable steam cycle. The condensate pump helps prevent water accumulation in the condenser, ensuring a low-pressure environment for the incoming steam in subsequent cycles. Additionally, proper condensate return enhances the overall thermal efficiency of the power plant by reducing the need for additional makeup water.
Condensate pumps come in various designs, including centrifugal pumps and positive displacement pumps, and their selection is based on factors such as system pressure, flow requirements, and the distance the condensate needs to be pumped. Regular maintenance and monitoring of condensate pumps are essential to ensure their continued reliability and optimal performance in steam power systems.
Steam Accumulator:
A steam accumulator is a storage device in steam power systems designed to store excess steam during periods of low demand and release it when demand increases. This serves as a means of smoothing out fluctuations in steam supply and demand, contributing to the stability and efficiency of back pressure steam turbines.
During times of low demand or when the steam production exceeds the immediate requirements, the excess steam is directed into the steam accumulator. The accumulator stores this surplus steam at a high pressure. When the demand for steam rises, the stored steam can be released to supplement the current steam production, ensuring a continuous and stable supply.
Steam accumulators are particularly beneficial in situations where there are rapid and significant variations in steam demand, such as in industrial processes with intermittent heating requirements. By acting as a buffer, the steam accumulator helps prevent abrupt pressure changes in the steam distribution system and allows the back pressure steam turbine to operate more efficiently.
The design and sizing of steam accumulators involve considerations of pressure, temperature, and storage capacity. Properly implemented steam accumulators contribute to energy efficiency, reduced stress on the steam generation system, and improved responsiveness to varying steam demands.
Turbine Efficiency Curve:
The turbine efficiency curve is a graphical representation of how the efficiency of a steam turbine varies across different operating conditions. It provides a valuable tool for engineers and operators to analyze and optimize the performance of back pressure steam turbines under varying loads and steam conditions.
The x-axis of the curve typically represents the turbine’s operating range, often in terms of steam flow rate or power output. The y-axis represents the efficiency of the turbine at each corresponding point on the curve. The curve illustrates how the turbine efficiency changes as operating conditions, such as steam pressure or temperature, vary.
A well-designed turbine efficiency curve allows operators to identify the optimal operating point for the given conditions, maximizing the turbine’s efficiency and overall performance. It also aids in understanding the impact of load changes on efficiency and helps in making informed decisions about turbine operation.
Factors influencing the shape of the efficiency curve include the design of the turbine, the quality of steam, and the control mechanisms in place. Regular monitoring and analysis of the turbine efficiency curve enable operators to identify potential issues, implement improvements, and ensure that the back pressure steam turbine operates within its optimal efficiency range for prolonged reliability and cost-effective energy generation.
Critical Speed:
Critical speed, in the context of steam turbines, refers to the rotational speed at which a turbine rotor or shaft experiences resonance and significant vibrations. It is a crucial parameter in the design and operation of back pressure steam turbines to prevent mechanical failures and ensure the long-term reliability of the system.
When a rotor rotates at or near its critical speed, the natural frequencies of the rotor coincide with the excitation frequencies caused by imbalances or external forces. This resonance can lead to excessive vibrations, which may result in fatigue and failure of turbine components, such as blades or shafts.
Engineers carefully analyze the critical speed of a steam turbine during the design phase. Factors such as the rotor’s mass distribution, stiffness, and damping characteristics influence the critical speed. Balancing techniques, including precision manufacturing and assembly, are employed to avoid resonant conditions during operation.
Regular monitoring and analysis of turbine vibrations are essential to detect any deviations from normal behavior that might indicate approaching critical speeds. Maintaining safe operating speeds and addressing any resonance issues contribute to the longevity and reliability of back pressure steam turbines in power generation applications.
Rotor Balancing:
Rotor balancing is a critical process in the design and maintenance of back pressure steam turbines, ensuring that the rotating components of the turbine operate smoothly and reliably. Balancing aims to minimize vibrations and reduce the potential for mechanical failures caused by uneven distribution of mass.
During operation, a rotating rotor can develop imbalances due to variations in the distribution of mass or changes in the operating conditions. These imbalances can lead to increased vibrations, which, if left unaddressed, may result in fatigue and premature wear of turbine components.
Rotor balancing involves the careful adjustment of the mass distribution to eliminate or minimize vibrations. This process may be carried out during the manufacturing of the turbine components or as part of routine maintenance. Balancing techniques include adding or removing mass strategically, using counterweights or trim balancing.
Modern rotor balancing often employs precision instruments and computerized systems to measure and analyze vibrations. Dynamic balancing, where adjustments are made while the rotor is in operation, allows for precise fine-tuning.
Effective rotor balancing is crucial for the overall performance and longevity of back pressure steam turbines. It contributes to smoother operation, reduced wear on components, and enhanced reliability, ensuring that the turbine operates within specified tolerances and delivers optimal efficiency in power generation.
Inlet Flow Path:
The inlet flow path in a back pressure steam turbine refers to the route steam takes as it enters the turbine from the steam source, such as a boiler or heat recovery system. This pathway is a critical element in the turbine’s design, influencing the efficiency and overall performance of the system.
The inlet flow path typically includes components like nozzles, diaphragms, and other control mechanisms that guide and control the flow of steam into the turbine. Each component plays a specific role in preparing the steam for expansion, ensuring uniform distribution, and optimizing the conditions for energy extraction.
Nozzles in the inlet flow path accelerate the steam, converting its pressure into velocity. This high-velocity steam is then directed onto the turbine blades, where it expands, producing mechanical work. Diaphragms help control the expansion process, allowing for multiple stages of the turbine to extract energy efficiently.
The design of the inlet flow path is crucial for achieving optimal energy conversion and minimizing losses. Engineers carefully consider factors such as steam pressure, temperature, and velocity in designing these components. Computational fluid dynamics and advanced modeling techniques aid in optimizing the geometry and performance of the inlet flow path.
Efficient inlet flow path design contributes to the overall effectiveness of back pressure steam turbines, ensuring that steam is utilized to its maximum potential for electricity generation and other industrial applications.
Main Key Features of a Back Pressure Steam Turbine
A back pressure steam turbine is a type of steam turbine used in power generation and industrial applications. Unlike condensing steam turbines, which exhaust steam at a lower pressure and temperature, back pressure turbines release steam at a higher pressure. This higher exhaust pressure enables the utilization of steam for various processes, such as heating or industrial applications, after it passes through the turbine.
In a back pressure turbine, steam is admitted at a high pressure, and it expands through the turbine blades, causing the rotor to turn and generate mechanical energy. The steam is then exhausted at an elevated pressure, allowing it to be used for heating purposes in industrial processes or district heating systems. This design is particularly suitable for applications where both electricity generation and thermal energy are required, making it a common choice in cogeneration or combined heat and power (CHP) systems.
The efficiency of a back pressure steam turbine is influenced by factors such as steam inlet pressure, turbine design, and the selection of operating parameters. Balancing the steam flow rate and optimizing the exhaust pressure are critical for achieving the desired performance and energy conversion efficiency in back pressure turbines.
Steam Turbine Efficiency:
Steam turbine efficiency is a crucial parameter that measures how effectively a steam turbine converts the thermal energy of steam into mechanical work. It is expressed as the ratio of the actual mechanical power output to the energy content of the steam entering the turbine. Higher efficiency indicates better utilization of the steam’s energy and, consequently, improved performance in power generation or industrial processes.
Efficiency in steam turbines is influenced by various factors, including turbine design, operating conditions, steam quality, and maintenance practices. Modern turbines are designed to achieve high efficiency levels, typically ranging from 40% to 50%, depending on the specific application and technology used.
Efficiency improvements can be achieved through advancements in turbine design, materials, and control systems. Proper maintenance, regular inspections, and optimization of operating parameters also contribute to maintaining or enhancing steam turbine efficiency over its operational life. Monitoring and improving efficiency are essential aspects of sustainable and economical energy generation, making it a key consideration in the design and operation of steam turbine systems.
Turbine Exhaust Pressure:
Turbine exhaust pressure refers to the pressure at which steam exits the steam turbine after performing mechanical work. In the context of back pressure steam turbines, this parameter is of particular importance because it determines the energy available for subsequent processes or applications.
In a back pressure turbine, the exhaust pressure is intentionally maintained at a higher level compared to condensing turbines. This higher exhaust pressure allows the steam to be used for various industrial processes or heating applications after leaving the turbine. The selection of the exhaust pressure is a critical design consideration as it directly influences the potential for extracting additional useful work from the steam.
Optimizing the turbine exhaust pressure involves a trade-off between maximizing electricity generation and utilizing the steam for other applications. Engineers carefully select the exhaust pressure based on the specific requirements of the overall system, aiming to achieve a balance that maximizes the overall efficiency and economic viability of the power generation or cogeneration plant. Control systems, such as governor mechanisms, play a crucial role in maintaining the desired turbine exhaust pressure under varying operating conditions.
Power Generation:
Power generation refers to the process of converting various forms of energy into electrical power. Steam turbines, including back pressure steam turbines, play a significant role in power generation by converting the thermal energy of steam into mechanical energy, which is then used to generate electricity.
In the context of back pressure steam turbines, power generation begins with the introduction of high-pressure steam into the turbine. The steam expands through the turbine blades, causing the rotor to turn and generate mechanical work. This rotational energy is then used to drive an electrical generator, converting the mechanical energy into electrical power.
The efficiency and performance of the back pressure steam turbine directly impact the amount of electricity generated. Engineers and operators carefully design and control the system to optimize power output while considering factors such as steam conditions, turbine design, and overall plant efficiency.
Power generation using back pressure steam turbines is prevalent in various industries and power plants, especially in applications where simultaneous electricity generation and thermal energy utilization are required, making it a versatile solution in combined heat and power (CHP) or cogeneration systems.
Energy Conversion:
Energy conversion refers to the process of transforming one form of energy into another. In the case of back pressure steam turbines, the primary focus is on converting the thermal energy stored in high-pressure steam into mechanical work and, ultimately, electrical energy.
The energy conversion process in a back pressure steam turbine involves several stages. First, high-pressure steam is introduced into the turbine, where it expands through the turbine blades. This expansion causes the turbine rotor to rotate, converting the thermal energy of steam into mechanical energy.
The mechanical energy generated by the turbine is then utilized for various purposes, with the most common application being the rotation of an electrical generator. The generator, coupled with the turbine, converts the mechanical energy into electrical power. This process demonstrates the fundamental principle of energy conversion – changing the form of energy from steam’s high-pressure and high-temperature state to electricity.
Efficiency in energy conversion is a key consideration in the design and operation of back pressure steam turbines, ensuring that a significant portion of the thermal energy is effectively transformed into useful electrical power for industrial, commercial, or residential use.
Condensing Steam Turbine:
A condensing steam turbine is a type of steam turbine used in power generation where steam is allowed to expand and do mechanical work, and then it is condensed back into water. Unlike back pressure steam turbines, condensing turbines operate with lower exhaust pressures, often below atmospheric pressure.
In a condensing steam turbine, high-pressure steam is introduced into the turbine, where it expands through the blades, generating mechanical work. After passing through the turbine, the low-pressure steam is condensed back into water using a condenser. The condensation of steam allows the turbine to operate at lower pressures, improving the efficiency of the energy conversion process.
Condensing turbines are commonly used in power plants where the objective is to maximize electricity generation and overall efficiency. The lower exhaust pressure results in a larger pressure drop across the turbine, extracting more work from the steam. This design is suitable for large-scale power generation facilities that focus on optimizing electricity output without the need for simultaneous thermal energy utilization.
Steam Inlet Pressure:
Steam inlet pressure is a critical parameter in the operation of steam turbines, including back pressure steam turbines. It refers to the pressure at which high-temperature and high-pressure steam enters the turbine for the purpose of energy conversion.
In the context of back pressure turbines, maintaining an optimal steam inlet pressure is essential for achieving efficient and reliable performance. The inlet pressure affects the energy available for conversion and, consequently, the power output of the turbine. Engineers carefully design systems to ensure that steam entering the turbine is at the appropriate pressure to maximize the efficiency of the energy conversion process.
The selection of steam inlet pressure is influenced by factors such as the desired power output, turbine design, and the overall configuration of the power generation or industrial process. Controlling and regulating steam inlet pressure is typically managed by control systems and valves to adapt to varying operating conditions and demands, ensuring the turbine operates within specified parameters for optimal performance.
Turbine Blades:
Turbine blades are crucial components in steam turbines responsible for extracting mechanical energy from the expanding steam. In back pressure steam turbines, these blades play a key role in the conversion of thermal energy into rotational motion.
The design and material composition of turbine blades are critical factors influencing the efficiency and performance of the steam turbine. Blades are shaped to efficiently guide the steam flow, optimizing the extraction of energy as steam expands through the turbine. Materials with high strength and resistance to corrosion and erosion are chosen to withstand the demanding conditions of high-speed steam flows.
There are various types of turbine blades, including impulse and reaction blades, each designed to handle specific pressure and velocity profiles of steam. The arrangement and geometry of these blades contribute to the overall efficiency of the turbine in converting steam energy into mechanical work. Advances in blade technology, including aerodynamics and materials science, continue to improve the overall performance and longevity of steam turbines in power generation and industrial applications.
Thermal Power Plant:
A thermal power plant is a facility that generates electricity by converting heat energy into electrical power. Back pressure steam turbines often play a central role in the operation of thermal power plants, where the primary source of heat is typically the combustion of fossil fuels like coal, natural gas, or biomass.
In a thermal power plant, fuel is burned to produce high-temperature and high-pressure steam. This steam is then directed into a steam turbine, such as a back pressure turbine. As the steam expands through the turbine blades, it causes the rotor to turn, generating mechanical energy. The mechanical energy is subsequently used to drive an electrical generator, converting the thermal energy into electricity.
Thermal power plants are essential components of the global energy infrastructure, providing a reliable and large-scale source of electricity. The integration of back pressure steam turbines in such plants allows for the simultaneous production of electrical power and the utilization of steam for industrial processes or district heating, contributing to the overall efficiency and sustainability of the power generation system.
Steam Flow Rate:
Steam flow rate is a critical parameter in the operation of steam turbines, representing the quantity of steam that passes through the turbine per unit of time. In the context of back pressure steam turbines, the steam flow rate is a key factor influencing the power output and overall efficiency of the turbine.
The control and optimization of steam flow rate involve considerations of system design, turbine capacity, and the specific requirements of the power generation or industrial process. Maintaining a balance between steam flow rate and turbine capacity is essential for achieving optimal performance. Too high or too low a steam flow rate can impact the efficiency and reliability of the turbine.
Engineers and operators carefully design and control steam flow rates based on factors such as the desired power output, turbine design specifications, and the need to meet varying operational conditions. Accurate measurement and control of steam flow are essential for ensuring the stability and efficiency of back pressure steam turbines in diverse applications.
Power Output:
Power output in the context of back pressure steam turbines refers to the amount of mechanical or electrical power generated by the turbine as a result of converting the thermal energy of steam. It is a crucial performance metric that directly impacts the overall efficiency and effectiveness of the power generation or industrial process.
The power output is influenced by factors such as steam conditions (pressure and temperature), turbine design, steam flow rate, and the rotational speed of the turbine. Engineers aim to optimize these factors to achieve the desired level of power output while maintaining system reliability and efficiency.
Efficient power output is a key consideration in the design and operation of back pressure steam turbines, especially in applications where simultaneous electricity generation and thermal energy utilization are essential. It involves a careful balance between maximizing the conversion of steam energy into useful work and meeting the specific requirements of the intended use, whether it be electricity generation, industrial processes, or combined heat and power applications.
Steam Velocity:
Steam velocity is the speed at which steam travels through the steam turbine blades during the expansion process. In back pressure steam turbines, managing and optimizing steam velocity is crucial for efficient energy conversion and turbine performance.
The velocity of steam is influenced by factors such as steam pressure, temperature, and the design of the turbine blades. Engineers carefully design the blades to control steam flow, ensuring that the velocity is within optimal ranges for efficient energy extraction. Proper control of steam velocity helps prevent issues such as erosion or excessive wear on turbine components.
Maintaining an appropriate steam velocity is essential for maximizing the energy transfer from steam to the turbine blades. This ensures that a significant portion of the thermal energy is effectively converted into mechanical work, contributing to the overall efficiency of the back pressure steam turbine in power generation or industrial applications. Control systems are employed to manage steam velocity under different operating conditions, ensuring stable and reliable turbine performance.
Steam Expansion:
Steam expansion is a fundamental process in steam turbines, including back pressure steam turbines, where high-pressure steam undergoes controlled expansion to produce mechanical work. This expansion process is a key aspect of converting the thermal energy stored in steam into useful energy, typically in the form of rotation that drives a generator for electricity generation.
In a back pressure steam turbine, steam expansion occurs as the high-pressure steam is admitted into the turbine. As steam flows through the turbine blades, it expands, and its pressure and temperature decrease. This expansion leads to the rotation of the turbine rotor, generating mechanical energy.
The efficiency and performance of a back pressure steam turbine depend on the careful design and control of the steam expansion process. Engineers optimize factors such as blade design, steam inlet conditions, and turbine geometry to ensure efficient energy extraction during steam expansion. The goal is to harness as much energy as possible from the expanding steam while maintaining stable and reliable turbine operation.
Steam Pressure Control:
Steam pressure control is a critical aspect of operating back pressure steam turbines efficiently. It involves managing and regulating the pressure of steam entering the turbine to ensure optimal performance and safety.
Control systems are employed to maintain a consistent and appropriate steam pressure based on the operational requirements of the system. These systems often include valves and sensors that monitor and adjust steam flow to achieve the desired pressure levels. Proper control of steam pressure is essential for preventing issues such as turbine instability, excessive wear on components, or inefficient energy conversion.
In back pressure turbines, precise steam pressure control is particularly important because it directly affects the turbine’s power output and overall efficiency. Engineers design control mechanisms to respond to variations in demand and operating conditions, ensuring the turbine operates within specified pressure ranges for optimal performance in power generation or industrial applications.
Generator Drive:
A generator drive refers to the mechanism by which the mechanical energy produced by a steam turbine, such as a back pressure steam turbine, is used to drive an electrical generator. The generator converts this mechanical energy into electrical power.
In the context of back pressure steam turbines, the rotating shaft connected to the turbine rotor is coupled to the generator. As the turbine blades extract energy from expanding steam, the rotor turns, causing the generator to rotate. This rotation induces the generation of electrical power through electromagnetic processes within the generator.
Efficient generator drive systems are crucial for maximizing the conversion of mechanical energy into electrical power. The design and synchronization of the turbine and generator, along with control systems, play a significant role in achieving stable and reliable power generation. The output from the generator can be further controlled and managed to meet the demands of the electrical grid or specific industrial applications.
Turbine Governor:
A turbine governor is a control system used to regulate the speed and output of a steam turbine, including back pressure steam turbines. Its primary function is to maintain a consistent and stable rotational speed of the turbine, which, in turn, ensures the desired electrical power output.
The governor achieves this by adjusting the flow of steam entering the turbine based on changes in load or demand. If there is an increase in electrical demand, the governor opens the steam control valves to allow more steam into the turbine, increasing the power output. Conversely, if the demand decreases, the governor reduces steam flow to maintain a steady speed and output.
Turbine governors are crucial for grid stability and the reliable operation of power plants. They help prevent fluctuations in electrical frequency, ensuring a consistent and synchronized supply of electricity to the grid. Modern turbine governors use advanced control algorithms and feedback systems to respond quickly and accurately to changes in load, contributing to the overall stability and efficiency of the power generation system.
Turbine Performance:
Turbine performance in the context of back pressure steam turbines encompasses various factors that influence the efficiency, reliability, and overall effectiveness of the turbine in converting steam energy into mechanical work and electricity.
Key aspects of turbine performance include steam inlet conditions, steam flow rate, expansion efficiency, and the control of turbine parameters such as speed and pressure. Engineers focus on designing turbines to operate within optimal ranges for these factors, balancing the need for electricity generation with other potential uses of the steam, such as industrial processes or district heating.
Monitoring and assessing turbine performance involve the use of instrumentation and control systems that provide real-time data on operating conditions. Regular maintenance and adjustments based on performance data are essential to ensure the turbine operates efficiently and meets its design specifications over its operational life. Optimizing turbine performance contributes to the overall success of power generation systems, especially in applications where both electricity and thermal energy are valuable outputs.
Cogeneration:
Cogeneration, also known as combined heat and power (CHP), is a highly efficient energy generation strategy that involves the simultaneous production of electricity and useful thermal energy from a single energy source. Back pressure steam turbines are commonly employed in cogeneration systems to achieve this dual output.
In a cogeneration plant, high-pressure steam is directed into a back pressure steam turbine, generating mechanical work to drive an electrical generator. The electricity produced is then utilized for various purposes. Importantly, the steam, after passing through the turbine, retains a significant amount of thermal energy. This low-pressure steam is then used for heating or industrial processes, maximizing the overall efficiency of the energy conversion.
Cogeneration is advantageous in applications where there is a demand for both electricity and thermal energy, such as in industrial facilities, district heating systems, or commercial buildings. The integration of back pressure steam turbines in cogeneration enhances energy sustainability by efficiently utilizing the available energy and minimizing waste.
Steam Cycle:
The steam cycle refers to the continuous and cyclical process through which steam is generated, expanded in a steam turbine, and then condensed back into water to be reused. This cycle is fundamental to the operation of steam turbines, including back pressure steam turbines, in power generation and industrial applications.
The steam cycle typically begins with the combustion of a fuel source, such as coal, natural gas, or biomass, to produce high-temperature and high-pressure steam. This steam is then directed into the steam turbine, where it expands, producing mechanical work that is used to drive an electrical generator. After passing through the turbine, the low-pressure steam is condensed back into water in a condenser, and the cycle repeats.
Efficient operation of the steam cycle is essential for optimizing the performance of back pressure steam turbines. Engineers carefully design and control the various stages of the cycle to ensure maximum energy conversion and minimize losses. The steam cycle is a cornerstone of steam power generation, providing a reliable and widely used method for converting thermal energy into electricity.
Renewable Energy:
While not directly tied to the back pressure steam turbine itself, the concept of renewable energy is significant in the broader context of sustainable power generation. Renewable energy sources, such as wind, solar, hydro, and biomass, are gaining prominence in the quest for cleaner and more environmentally friendly electricity production.
Back pressure steam turbines can be part of renewable energy systems, particularly when biomass is used as a fuel source. Biomass, derived from organic materials, can be burned to produce steam that powers a back pressure steam turbine for electricity generation. The utilization of renewable resources in conjunction with steam turbines contributes to reducing greenhouse gas emissions and dependence on fossil fuels.
In the ongoing transition toward a more sustainable energy landscape, the integration of renewable energy sources with technologies like back pressure steam turbines showcases a commitment to environmentally responsible power generation. This approach aligns with global efforts to address climate change and promote a cleaner and more sustainable energy future.
Steam Turbines
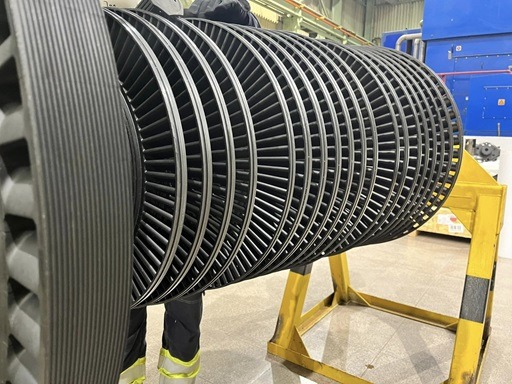
Steam turbines are remarkable machines that convert thermal energy from steam into mechanical work, widely used in power generation, industrial processes, and propulsion systems. They operate on the principle of expanding high-pressure steam through a series of blades, causing rotation that can drive generators or other machinery. Over time, engineers have developed various types of steam turbines, each designed to optimize efficiency, performance, and application-specific requirements. Below is an exploration of the primary types of steam turbines, their configurations, and their uses.
1. Impulse Turbines
Impulse turbines operate based on the impulse principle, where high-pressure steam is directed through nozzles to form high-velocity jets that strike the turbine blades. The kinetic energy of the steam is transferred to the blades, causing the rotor to spin. In this design, the pressure drop occurs entirely in the nozzles, and the blades experience no significant pressure change as the steam passes through. A classic example of an impulse turbine is the De Laval turbine, which features a single stage and is known for its simplicity and high rotational speeds. Another well-known design is the Curtis turbine, which uses multiple stages of moving and stationary blades to extract energy more efficiently in a compact form.
Impulse turbines are often used in small-scale power generation or as the high-pressure stages in larger systems. Their advantages include simplicity and the ability to handle high-pressure steam effectively, though they may be less efficient at lower speeds or with variable loads.
2. Reaction Turbines
In contrast to impulse turbines, reaction turbines rely on both pressure drop and steam expansion across the turbine blades themselves. As steam passes through the moving blades, it accelerates and expands, creating a reactive force (similar to how a rocket works) that drives the rotor. This design was pioneered by Sir Charles Parsons, and the Parsons turbine remains a foundational example. Reaction turbines typically feature multiple stages, with alternating rows of fixed (stator) and moving (rotor) blades, allowing for gradual energy extraction and higher efficiency.
Reaction turbines are widely used in large power plants because they excel at handling lower-pressure steam and can achieve greater efficiency over a range of operating conditions. However, they are more complex and costly to manufacture due to the precision required in blade design and staging.
3. Combination (Impulse-Reaction) Turbines
Many modern steam turbines combine impulse and reaction principles to optimize performance across different pressure ranges. For example, the high-pressure stages might use an impulse design to handle the initial steam conditions, while the low-pressure stages transition to a reaction design for better efficiency as the steam expands. This hybrid approach allows turbines to adapt to a wide variety of operating conditions, making them common in large-scale electricity generation plants.
4. Back-Pressure Turbines
Back-pressure turbines exhaust steam at a pressure higher than atmospheric pressure, allowing the exhaust steam to be used for industrial processes like heating, drying, or driving other machinery. These turbines are often found in cogeneration systems, where both electricity and heat are needed, such as in paper mills, chemical plants, or district heating systems. While they sacrifice some efficiency in power generation compared to condensing turbines, their ability to provide dual outputs makes them highly economical in specific applications.
5. Condensing Turbines
Condensing turbines are designed to maximize power output by exhausting steam into a vacuum, typically created by a condenser. This lowers the back pressure, allowing the steam to expand further and extract more energy. These turbines are the backbone of most large-scale power plants, including coal, nuclear, and combined-cycle gas plants. Their high efficiency comes at the cost of requiring a cooling system (often water-based), which adds complexity and environmental considerations.
6. Extraction Turbines
Extraction turbines are a versatile subtype that allow steam to be “extracted” at intermediate pressures from various stages of the turbine. This extracted steam can be used for industrial processes or heating, while the remaining steam continues through the turbine to generate power. These turbines are common in facilities needing both electricity and steam at different pressure levels, offering flexibility and efficiency in combined heat and power (CHP) systems.
7. Reheat Turbines
Reheat turbines improve efficiency by incorporating a reheat cycle. After passing through the high-pressure stages, steam is sent back to the boiler to be reheated before entering the intermediate- or low-pressure stages. This process increases the average temperature at which heat is added, boosting the turbine’s thermodynamic efficiency. Reheat designs are standard in large, high-efficiency power plants, though they require additional equipment and control systems.
8. Low-Pressure, Intermediate-Pressure, and High-Pressure Turbines
In large power plants, steam turbines are often divided into separate sections based on steam pressure: high-pressure (HP), intermediate-pressure (IP), and low-pressure (LP) turbines. These sections are typically mounted on a single shaft and work together to extract energy as the steam expands from high to low pressure. Each section is optimized for its specific pressure range, with blade sizes and designs varying accordingly—HP turbines have smaller, robust blades, while LP turbines have larger blades to handle the expanded, lower-pressure steam.
9. Single-Stage vs. Multi-Stage Turbines
Steam turbines can also be classified by the number of stages. Single-stage turbines, like the De Laval design, are simple and compact, suitable for small-scale or high-speed applications. Multi-stage turbines, such as those used in power plants, consist of multiple sets of blades, allowing for gradual energy extraction and higher efficiency. Multi-stage designs dominate in large-scale applications due to their ability to handle large steam volumes and pressure drops.
Applications and Considerations
Each type of steam turbine serves a specific purpose. Impulse turbines might power small generators or pumps, while reaction turbines drive massive gigawatt-scale power stations. The choice of turbine type depends on factors like steam conditions (pressure, temperature, and flow rate), desired output (power, heat, or both), and operational constraints (space, cost, and maintenance). Let’s dive deeper into each type with additional details on their design, mechanics, and real-world applications.
1. Impulse Turbines
Impulse turbines rely on the conversion of steam’s potential energy into kinetic energy before it interacts with the blades. The steam is accelerated through stationary nozzles, which are precisely shaped (often converging-diverging nozzles) to achieve supersonic velocities. When this high-speed jet hits the turbine’s bucket-shaped blades, the momentum transfer causes rotation. The blades are symmetrically designed to minimize axial thrust, and the steam exits at roughly the same pressure it entered, having lost much of its kinetic energy.
- De Laval Turbine: Invented by Gustaf de Laval in the late 19th century, this single-stage turbine was revolutionary for its time. It’s compact, with a single row of blades, and can reach speeds exceeding 30,000 RPM, making it ideal for driving high-speed machinery like centrifugal pumps or small generators. However, its efficiency drops with varying loads, limiting its use to niche applications.
- Curtis Turbine: Developed by Charles G. Curtis, this design adds a velocity-compounding feature. Steam passes through multiple rows of moving blades interspersed with stationary blades that redirect the flow. This staged approach reduces the rotor speed (compared to De Laval) while extracting more energy, making it suitable for early electrical generation systems.
- Applications: Impulse turbines shine in high-pressure, low-flow scenarios, such as topping turbines in combined-cycle plants or standalone units in remote locations. They’re less common in modern large-scale power generation due to efficiency limitations but remain critical in specialized industrial setups.
2. Reaction Turbines
Reaction turbines operate on a different principle: the blades act as nozzles themselves, accelerating and expanding the steam as it flows through. This creates a drop in pressure across each stage, generating a reactive force that drives the rotor. The stator blades (fixed) direct steam onto the rotor blades (moving), and the process repeats across multiple stages. The degree of reaction—typically around 50% in a Parsons turbine—refers to the proportion of energy extracted via reaction versus impulse.
- Parsons Turbine: Sir Charles Parsons’ 1884 invention introduced the multi-stage reaction concept, a breakthrough that transformed power generation. His turbines feature dozens or even hundreds of stages, with blade heights increasing as steam expands. This gradual energy extraction maximizes efficiency, especially at lower pressures.
- Design Nuances: Reaction turbine blades are airfoil-shaped, requiring precise manufacturing to handle aerodynamic forces and steam expansion. The rotor and stator blades are often paired in a 1:1 ratio, creating a balanced, continuous flow. Axial thrust is a challenge, necessitating thrust bearings to stabilize the rotor.
- Applications: Reaction turbines dominate in large fossil-fuel, nuclear, and geothermal power plants due to their scalability and efficiency at handling high steam volumes. They’re less suited to small-scale or high-pressure-only applications, where impulse designs may outperform.
3. Combination (Impulse-Reaction) Turbines
Combination turbines blend the strengths of both designs. The high-pressure section often uses impulse stages to manage the intense initial conditions (e.g., 200 bar, 540°C), where nozzles and robust blades excel. As steam pressure drops, the turbine transitions to reaction stages, leveraging expansion for efficiency in the intermediate- and low-pressure zones. This hybrid layout is tailored to the steam cycle’s thermodynamic profile.
- Mechanics: The transition between impulse and reaction stages is seamless, with blade designs and staging adjusted to match pressure gradients. For example, early stages might feature pure impulse (100% pressure drop in nozzles), while later stages approach 50% reaction.
- Advantages: This design optimizes efficiency across a wide pressure range, reduces mechanical stress, and allows for compact yet powerful turbines. It’s a staple in modern supercritical and ultra-supercritical coal plants, where steam conditions push material limits.
- Applications: Found in utility-scale power generation, especially where efficiency and output must be maximized, such as in combined-cycle plants integrating gas and steam turbines.
4. Back-Pressure Turbines
Back-pressure turbines exhaust steam at a usable pressure (e.g., 5-20 bar) rather than condensing it into a vacuum. The exhaust steam retains significant thermal energy, making it ideal for downstream processes. These turbines often operate in a non-condensing mode, with exhaust piped directly to industrial systems.
- Design Details: Simpler than condensing turbines, they lack a condenser and cooling system, reducing capital costs. Blade staging is optimized for a specific exhaust pressure, balancing power output with steam quality for process use.
- Efficiency Trade-Off: Electrical efficiency is lower than condensing turbines (since less energy is extracted), but total energy efficiency soars when process heat is factored in—sometimes exceeding 80% in cogeneration setups.
- Applications: Common in industries like pulp and paper (for drying), sugar refining (for evaporation), and petrochemical plants (for heating). They’re also used in district heating systems, where exhaust steam warms buildings.
5. Condensing Turbines
Condensing turbines push efficiency to the limit by exhausting steam into a vacuum (e.g., 0.05 bar), created by a condenser cooled with water or air. This maximizes the pressure drop across the turbine, extracting nearly all available energy from the steam.
- Mechanics: The low-pressure stages feature massive blades—sometimes over a meter long—to handle the high-volume, low-density steam. Condensers require significant infrastructure, including cooling towers or river/ocean water systems, adding complexity.
- Materials and Challenges: LP blades face erosion from wet steam (containing water droplets), necessitating alloys like titanium or protective coatings. Vacuum maintenance is critical, as leaks reduce efficiency.
- Applications: The backbone of baseload power plants—coal, nuclear, and gas-fired—where maximum electrical output is the goal. They’re less practical in small-scale or heat-focused systems due to their reliance on cooling.
6. Extraction Turbines
Extraction turbines offer flexibility by allowing steam to be tapped at intermediate points. Valves control the extraction process, diverting steam at specific pressures (e.g., 10 bar for heating, 2 bar for feedwater preheating) while the rest continues to the condenser.
- Design Complexity: Multiple extraction points require sophisticated control systems and additional piping. Blade staging must account for variable flow rates, as extraction reduces steam volume in later stages.
- Benefits: They balance power and heat output, adapting to fluctuating demands. Efficiency remains high when extraction is optimized with process needs.
- Applications: Prevalent in refineries, steel mills, and CHP plants, where steam serves dual purposes—electricity for operations and heat for processes like distillation or drying.
7. Reheat Turbines
Reheat turbines enhance efficiency by interrupting the expansion process. After the HP stages, steam (now at reduced pressure and temperature) returns to the boiler for reheating (e.g., back to 540°C), then re-enters the IP and LP stages. This raises the cycle’s average heat-addition temperature, a key thermodynamic advantage.
- Mechanics: Reheat requires additional piping, valves, and boiler capacity. Double-reheat systems (two reheats) push efficiency further but increase costs. LP stages must handle wetter steam post-reheat, requiring moisture separators.
- Efficiency Gains: Single reheat boosts efficiency by 4-5%, while double reheat adds another 2-3%, making them viable in ultra-efficient plants (e.g., 45%+ thermal efficiency).
- Applications: Standard in modern fossil-fuel plants, especially supercritical designs, and some nuclear plants with high steam output.
8. Low-Pressure, Intermediate-Pressure, and High-Pressure Turbines
In large systems, turbines are segmented into HP, IP, and LP units, often on a single shaft. Each section is a mini-turbine tailored to its steam conditions:
- HP Turbine: Small, robust blades handle ultra-high pressures (up to 300 bar) and temperatures (600°C+). Materials like chromium-steel alloys resist creep and corrosion.
- IP Turbine: Mid-sized blades manage reheated steam (20-50 bar), balancing strength and flow capacity.
- LP Turbine: Large blades (up to 1.5 meters) process low-pressure, high-volume steam, often in twin-flow designs to split the exhaust load.
- Applications: Universal in utility-scale plants, where modularity simplifies maintenance and optimization.
9. Single-Stage vs. Multi-Stage Turbines
- Single-Stage: Compact, with one set of blades, they’re fast and simple but inefficient for large power outputs. Used in small pumps, fans, or emergency generators.
- Multi-Stage: Multiple blade rows extract energy gradually, ideal for high-power applications. Complexity increases, but so does efficiency—up to 90% of available energy in modern designs.
Closing Thoughts
Steam turbines are marvels of engineering, with each type fine-tuned to its role. From the brute simplicity of a De Laval impulse turbine to the intricate staging of a reheat reaction turbine, their diversity reflects the ingenuity behind harnessing steam’s power. Whether driving a factory or lighting a city, these machines remain central to our energy landscape, evolving with advances in materials, controls, and thermodynamics.
Let’s continue expanding on the fascinating world of steam turbines, diving even deeper into the nuances of each type—covering their operational mechanics, historical context, material considerations, and modern innovations. The goal here is to provide a comprehensive picture of how these machines function and why they’re engineered the way they are.
1. Impulse Turbines
The impulse turbine’s elegance lies in its straightforward energy transfer: steam’s kinetic energy is the sole driver. The nozzles are critical—they’re often made of high-strength alloys like stainless steel or Inconel to withstand erosion from high-velocity steam, especially if it carries moisture or particulates. Blade design is equally vital; the “buckets” are typically curved and polished to minimize friction losses, with precise angles to maximize momentum transfer.
- Historical Context: Gustaf de Laval’s 1880s design was a leap forward during the Second Industrial Revolution, enabling high-speed machinery when electricity was still emerging. His turbines powered early cream separators (a key invention of his), showcasing their versatility beyond power generation.
- Velocity Compounding (Curtis): In a Curtis turbine, steam ricochets between moving and stationary blades multiple times within a stage. This reduces the rotor speed to manageable levels (e.g., 3,000-6,000 RPM) for coupling with generators, avoiding the need for gearboxes—a common requirement with De Laval’s ultra-fast designs.
- Modern Use: Today, impulse turbines are often “topping” units in hybrid plants, taking the first bite of high-pressure steam before handing it off to reaction stages. Advances in computational fluid dynamics (CFD) have refined nozzle and blade profiles, squeezing out extra efficiency.
- Challenges: Efficiency drops sharply if steam flow deviates from design conditions, and they’re prone to losses from turbulence if not perfectly aligned. Materials must also resist thermal shock from rapid startups.
2. Reaction Turbines
Reaction turbines are the workhorses of large-scale power, thriving on their ability to extract energy incrementally. Each stage contributes a small pressure drop, with steam velocity increasing as it passes through the rotor blades. This continuous expansion demands tight tolerances—gaps between rotor and stator must be minimized to prevent steam leakage, often requiring labyrinth seals or brush seals.
- Parsons’ Legacy: Parsons’ first turbine, built in 1884, powered a ship’s lighting system, proving the concept. By 1900, his multi-stage designs were generating megawatts, outpacing rivals. His insight was staging: rather than one big energy drop, he spread it across dozens of steps.
- Blade Dynamics: Blades are aerodynamically complex, resembling aircraft wings. Steam accelerates through converging passages in the rotor, creating lift-like forces. Blade lengths increase down the turbine (e.g., 20 mm in HP stages to 1 m in LP stages) to match the expanding steam volume.
- Material Science: High-temperature stages use nickel-based superalloys (e.g., Inconel 718) for creep resistance, while LP blades might use titanium to combat wet-steam erosion. Coatings like chromium carbide protect against wear.
- Applications Today: Reaction turbines power nuclear plants (handling saturated steam at 70 bar) and geothermal systems (low-pressure, corrosive steam), showcasing their adaptability. Efficiency can hit 90% of the ideal Rankine cycle in optimized designs.
3. Combination (Impulse-Reaction) Turbines
The combination turbine’s hybrid nature makes it a masterpiece of compromise. The impulse stages handle the brutal initial conditions—supercritical steam at 300 bar and 600°C—where robust, simple blades thrive. As pressure falls, reaction stages take over, leveraging expansion for finesse and efficiency. This staged approach mirrors the steam’s thermodynamic journey.
- Staging Details: A typical layout might feature 2-4 impulse stages followed by 10-20 reaction stages. The transition isn’t abrupt—designers blend characteristics, gradually increasing the reaction component (e.g., 10% to 50% across stages).
- Thermodynamic Edge: By tailoring each section to its pressure zone, losses from over-expansion or under-utilization are minimized. CFD and 3D modeling now optimize this blend, reducing shock losses where steam shifts behavior.
- Real-World Example: In a 1,000 MW coal plant, the HP turbine might be impulse-driven, the IP turbine a mix, and the LP turbine fully reaction-based, all on one shaft spinning at 3,600 RPM (for 60 Hz grids).
- Innovations: Adaptive staging, where blade angles adjust via actuators, is emerging, allowing turbines to handle variable steam inputs from renewable-integrated grids.
4. Back-Pressure Turbines
Back-pressure turbines prioritize versatility over raw power. The exhaust pressure is a design parameter—say, 10 bar for a chemical reactor or 3 bar for a dryer—set by downstream needs. This steam isn’t wasted in a condenser but repurposed, making these turbines stars of energy efficiency in the right context.
- Operational Mechanics: The turbine’s last stage is tuned to maintain the target exhaust pressure, often with fewer blades than a condensing turbine’s sprawling LP section. Steam quality (dryness) is critical—wet steam can damage process equipment downstream.
- Historical Use: Early 20th-century factories used back-pressure turbines to power machinery while heating workspaces, a precursor to modern cogeneration.
- Modern Systems: In a pulp mill, a 20 MW back-pressure turbine might generate electricity while supplying 50 tons/hour of 5-bar steam for digesters. Combined efficiency (power + heat) can exceed 85%.
- Limitations: Power output is capped by the exhaust pressure—lower pressure means more electricity, but less usable heat. Balancing these trade-offs requires precise engineering.
5. Condensing Turbines
Condensing turbines chase the thermodynamic ideal: maximum work from steam. The condenser creates a vacuum (e.g., 0.03-0.1 bar), dropping the exhaust temperature to 30-50°C, far below atmospheric boiling. This extends the Rankine cycle’s pressure range, extracting energy that other turbines leave behind.
- LP Stage Scale: LP blades are engineering marvels—some span over 1.5 meters, with tips moving at supersonic speeds (e.g., 500 m/s). Dual-flow designs split steam to reduce axial thrust and fit within casing limits.
- Cooling Systems: Water-cooled condensers dominate, using rivers or cooling towers (evaporating 1-2% of a plant’s output as waste heat). Air-cooled variants are growing in arid regions, though they’re less efficient.
- Challenges: Wet steam in LP stages erodes blades, requiring moisture separators or superheating. Vacuum leaks sap efficiency, demanding robust seals and monitoring.
- Applications: A 1,200 MW nuclear turbine might have three LP sections, each exhausting to its own condenser, producing enough power for a small city.
6. Extraction Turbines
Extraction turbines are the multitaskers of the turbine world, juggling power and process steam. Extraction points—typically 1-3 per turbine—are fitted with control valves, allowing operators to adjust flow on the fly. This adaptability suits industries with variable heat demands.
- Control Systems: Advanced turbines use digital governors to balance extraction and power output, responding to real-time needs. Steam bypassing an extraction point continues to the next stage, maintaining rotor stability.
- Design Trade-Offs: Each extraction reduces mass flow downstream, shrinking LP blade sizes compared to a pure condensing turbine. Efficiency hinges on matching extraction pressures to process requirements.
- Example: In a refinery, a 50 MW extraction turbine might supply 15 bar steam for reboilers (20 tons/hour) and 2 bar steam for tracing (10 tons/hour), with the rest condensed for power.
- Modern Twist: Some units pair with solar-thermal systems, extracting steam for industrial use while generating renewable power.
7. Reheat Turbines
Reheat turbines push the Rankine cycle’s limits by adding heat mid-process. After the HP turbine drops steam to 20-50 bar, reheating to 540-600°C boosts its enthalpy, allowing more work in the IP and LP stages. Double-reheat designs repeat this, though cost-benefit diminishes beyond two cycles.
- Mechanics: Reheat piping adds 10-20% to a plant’s footprint, with superheaters handling corrosive, high-temperature steam. Moisture separators post-reheat dry the steam, protecting LP blades.
- Efficiency Math: A single reheat lifts thermal efficiency from ~38% to 42-43%; double reheat might hit 45-47% in ultra-supercritical plants (600°C, 300 bar).
- Applications: Dominant in high-efficiency fossil plants, like Germany’s lignite stations or Japan’s advanced coal units. Nuclear plants occasionally use reheat with moisture separation for saturated steam cycles.
8. Low-Pressure, Intermediate-Pressure, and High-Pressure Turbines
These segmented turbines are a symphony of specialization. The HP turbine faces the harshest conditions, often operating near material limits (e.g., 620°C with advanced ferritic steels). IP turbines bridge the gap, while LP turbines handle the voluminous exhaust.
- Shaft Dynamics: A single shaft aligns all three, spinning at 3,000 RPM (50 Hz) or 3,600 RPM (60 Hz). Bearings and couplings absorb massive torque and thermal expansion.
- Applications: A 900 MW combined-cycle plant might pair an HP-IP turbine with twin LP units, optimizing gas-steam integration.
9. Single-Stage vs. Multi-Stage Turbines
- Single-Stage: Limited to 1-5 MW, they’re rugged and low-maintenance—think backup generators or marine pumps.
- Multi-Stage: Scaling to gigawatts, they’re the pinnacle of efficiency, with 50+ stages in some LP sections.
Final Notes
Steam turbines evolve with technology—ceramic coatings, 3D-printed blades, and AI-driven controls are pushing boundaries. Their adaptability keeps them relevant, from fossil giants to renewable hybrids, proving their enduring role in energy.
What Are Steam Turbines?
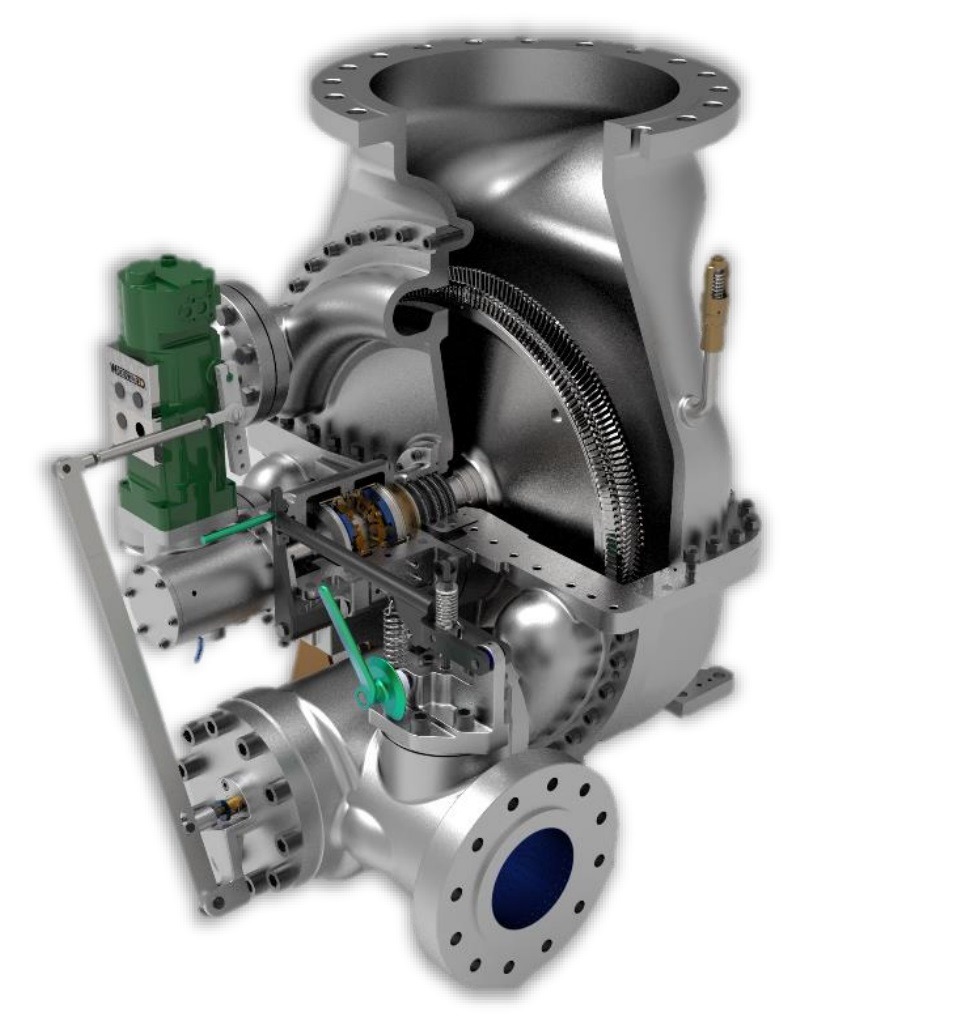
Steam turbines are mechanical devices that convert thermal energy from pressurized steam into rotational mechanical energy, which can then drive generators to produce electricity or power machinery like pumps and compressors. Invented in the late 19th century, they remain a cornerstone of power generation and industrial processes worldwide, prized for their efficiency, scalability, and adaptability to various heat sources—coal, nuclear, biomass, natural gas, or even solar thermal energy.
Basic Operating Principle
The steam turbine operates on the Rankine cycle, a thermodynamic process where heat transforms water into steam, which then expands to do work. Here’s how it unfolds:
- Heat Input: A heat source (e.g., burning fuel or nuclear fission) boils water in a boiler, creating high-pressure, high-temperature steam (typically 200-600°C, 10-300 bar).
- Expansion: This steam flows into the turbine, passing through blades mounted on a rotor. As it expands and loses pressure, it accelerates, pushing the blades and spinning the rotor.
- Work Output: The rotor’s rotation drives a connected shaft, powering a generator (for electricity) or other equipment.
- Exhaust: Spent steam exits—either to a condenser (cooling it back to water for reuse) or as usable heat (in CHP systems).
Efficiency depends on the steam’s initial conditions and the turbine’s design, typically ranging from 20% in small, simple units to over 45% in advanced power plants.
Types of Steam Turbines
Steam turbines vary widely based on design, pressure handling, and application. Here are the main categories:
- Impulse Turbines
- How They Work: Steam accelerates through stationary nozzles into high-velocity jets, striking curved blades (buckets) on the rotor. The pressure drop occurs entirely in the nozzles, not across the blades.
- Examples: The De Laval turbine (single-stage, high-speed) and Curtis turbine (velocity-compounded with multiple blade rows).
- Features: Simple, robust, and suited for high-pressure steam. Speeds can exceed 30,000 RPM in small units.
- Applications: Small-scale power generation, topping turbines in hybrid plants, or driving high-speed machinery.
- Reaction Turbines
- How They Work: Steam expands and accelerates through both fixed (stator) and moving (rotor) blades, dropping pressure gradually across each stage. The blades act like nozzles, creating a reactive force.
- Examples: The Parsons turbine, with dozens of stages for efficiency.
- Features: Complex, with airfoil-shaped blades and tight tolerances. Excels at lower pressures and high steam volumes.
- Applications: Large power plants (coal, nuclear, geothermal) needing maximum efficiency.
- Combination (Impulse-Reaction) Turbines
- How They Work: High-pressure stages use impulse designs, transitioning to reaction stages as pressure drops, optimizing energy extraction across the steam’s range.
- Features: Balances simplicity and efficiency, common in modern utility-scale turbines.
- Applications: Supercritical coal or combined-cycle gas plants.
- Condensing Turbines
- How They Work: Steam exhausts into a vacuum (0.03-0.1 bar) created by a condenser, maximizing pressure drop and energy output.
- Features: Large low-pressure (LP) blades handle high-volume steam. Requires cooling systems (water or air).
- Applications: Baseload power plants (e.g., 1,000 MW nuclear units).
- Back-Pressure Turbines
- How They Work: Steam exits at a higher pressure (e.g., 5-20 bar) for industrial use, rather than condensing.
- Features: Simpler, with dual power-and-heat output (CHP). Less efficient electrically but highly effective overall.
- Applications: Factories (e.g., paper mills) needing process steam.
- Extraction Turbines
- How They Work: Steam is tapped at intermediate stages for heating or processes, with the rest continuing to generate power.
- Features: Flexible, with control valves adjusting extraction flow.
- Applications: Refineries or district heating systems.
- Reheat Turbines
- How They Work: Steam exits the high-pressure (HP) stage, gets reheated in the boiler (e.g., to 540°C), then expands through intermediate-pressure (IP) and LP stages.
- Features: Boosts efficiency by 4-5% per reheat cycle. Complex, with extra piping.
- Applications: High-efficiency fossil-fuel plants.
- HP, IP, and LP Turbines
- How They Work: Segmented into sections on a single shaft, each optimized for its pressure range—small HP blades for high pressure, large LP blades for low pressure.
- Features: Modular, scalable, and standard in large plants.
- Applications: Gigawatt-scale power stations.
Historical Development
- Origins: Sir Charles Parsons invented the modern steam turbine in 1884, demonstrating a multi-stage reaction design that powered a ship’s lights. Gustaf de Laval’s 1880s impulse turbine complemented this, excelling at high speeds.
- Industrial Revolution: By 1900, turbines replaced steam engines in power generation, offering higher efficiency and scalability. Parsons’ designs scaled to megawatts within decades.
- 20th Century: Turbines evolved with metallurgy (e.g., chromium steels) and thermodynamics (e.g., reheat cycles), driving electrification worldwide.
Applications
- Power Generation: Over 80% of global electricity comes from steam turbines—coal (37%), nuclear (10%), gas (25%), and biomass/geothermal (small shares).
- Industrial: Drive compressors in chemical plants, pumps in refineries, or process steam in paper production.
- Marine Propulsion: Powered ships like the Titanic (29,000 hp) and warships until gas turbines took over post-WWII.
- Renewables: Biomass plants (e.g., wood-fired) and concentrated solar power use steam turbines for green energy.
Design and Materials
- Blades: HP blades (small, robust) use nickel-based superalloys (e.g., Inconel) for 600°C+ conditions. LP blades (large, lightweight) often use titanium to resist wet-steam erosion.
- Casings: Steel or alloy casings withstand pressure and thermal stress, with seals (labyrinth or brush) minimizing leaks.
- Rotor: Forged steel or alloy, balanced to handle 3,000-10,000 RPM and thermal expansion.
- Challenges: Creep (deformation at high heat), erosion (from wet steam), and fatigue (from cyclic loads) drive material innovation.
Efficiency and Performance
- Thermodynamic Limits: Ideal Rankine efficiency is ~60%, but real-world turbines hit 30-47%, depending on steam conditions (supercritical at 600°C, 300 bar pushes the upper end).
- Losses: Heat escapes via exhaust, friction, and leakage. Moisture in LP stages reduces output unless mitigated by superheating or separators.
- Enhancements: Reheat cycles, supercritical steam, and advanced blade profiles (via CFD) boost performance.
Modern Relevance
- Fossil Fuels: Coal and gas plants rely on steam turbines, though coal’s share declines with decarbonization.
- Nuclear: Essential for converting reactor heat to power, with massive LP turbines handling saturated steam.
- Renewables: Biomass (e.g., wood) and geothermal plants keep turbines relevant in green energy.
- Innovation: Ceramic blades (1,000°C tolerance), 3D-printed parts, and AI-driven controls push efficiency and durability.
Advantages and Limitations
- Advantages: High power output (up to 1,500 MW per unit), long lifespan (30-50 years), and fuel flexibility.
- Limitations: Complex infrastructure (boilers, condensers), slow startup (hours vs. minutes for gas turbines), and reliance on water for cooling.
Steam turbines are engineering marvels, blending physics, materials science, and ingenuity. From Parsons’ first 7.5 kW unit to today’s gigawatt giants, they’ve shaped our energy landscape and continue to adapt to a changing world. Whether driving a factory or lighting a city, their legacy endures.
Steam turbines are mechanical devices that transform the thermal energy stored in pressurized steam into rotational mechanical energy, which can then be used to generate electricity or power industrial equipment like pumps, compressors, or fans. They are a critical technology in modern energy production, found in power plants, factories, and even historical ships, and they operate by harnessing the expansion of steam to spin a rotor connected to a generator or machinery. Invented over a century ago, steam turbines remain vital due to their efficiency, reliability, and ability to work with diverse heat sources such as coal, natural gas, nuclear reactions, biomass like wood, or concentrated solar power. Below is a detailed exploration of how they work, their types, history, applications, design specifics, and their role today.
The basic principle behind a steam turbine is straightforward yet rooted in thermodynamics, specifically the Rankine cycle, which is a process that converts heat into work. Water is heated in a boiler using a fuel source, turning it into high-pressure steam at temperatures ranging from 200 to 600 degrees Celsius and pressures from 10 to 300 bar, depending on the system. This steam is then directed into the turbine, where it flows over a series of blades attached to a rotor. As the steam expands and loses pressure, it accelerates, pushing against the blades and causing the rotor to spin at speeds typically between 1,500 and 3,600 revolutions per minute, aligning with electrical grid frequencies of 50 or 60 Hz. The spinning rotor drives a shaft connected to a generator, producing electricity, or directly powers mechanical equipment. After passing through the turbine, the steam exits either into a condenser, where it cools back into water for reuse in a closed loop, or as usable heat for industrial processes in systems designed for combined heat and power.
Steam turbines come in several types, each designed for specific purposes based on how they handle steam and extract energy. Impulse turbines work by accelerating steam through stationary nozzles into high-speed jets that strike curved, bucket-shaped blades on the rotor. The pressure drop happens entirely in the nozzles, so the blades experience only kinetic energy transfer, not a pressure change. A famous example is the De Laval turbine, a single-stage design invented by Gustaf de Laval in the 1880s, capable of spinning at over 30,000 RPM, ideal for small, high-speed applications like pumps or early generators. Another impulse variant, the Curtis turbine, uses multiple rows of moving and stationary blades to compound velocity, reducing speed to a more manageable 3,000-6,000 RPM while extracting more energy, making it suitable for early power plants. Impulse turbines are simple, robust, and excel with high-pressure steam, but they lose efficiency at lower pressures or variable loads.
Reaction turbines, in contrast, extract energy through both pressure drop and steam expansion across the blades themselves. Steam passes through fixed stator blades that direct it onto moving rotor blades, where it accelerates and expands, creating a reactive force that drives the rotor. This design, pioneered by Sir Charles Parsons in 1884, typically features dozens of stages—alternating fixed and moving blades—to gradually extract energy, making it highly efficient for large-scale power generation. The blades are shaped like airfoils, requiring precise manufacturing to handle aerodynamic forces, and their size increases from high-pressure to low-pressure stages to accommodate expanding steam. Reaction turbines dominate in big power plants because they handle lower-pressure steam well and achieve efficiencies up to 90% of the theoretical maximum, though they’re more complex and costly than impulse designs.
Many modern turbines combine impulse and reaction principles to optimize performance across a wide pressure range. In these combination turbines, the high-pressure stages use an impulse design with nozzles and sturdy blades to manage initial conditions—say, 300 bar and 600°C—while later stages shift to reaction designs as the steam expands, improving efficiency in intermediate- and low-pressure zones. This hybrid approach is common in supercritical coal plants or combined-cycle gas plants, where steam conditions push material limits, and designers use computational fluid dynamics to fine-tune blade transitions, minimizing energy losses.
Condensing turbines are built to maximize power output by exhausting steam into a vacuum, typically 0.03 to 0.1 bar, created by a condenser cooled with water from rivers, oceans, or cooling towers, or sometimes air in dry regions. This low exhaust pressure allows the steam to expand further, extracting more energy. These turbines feature massive low-pressure blades—sometimes over 1.5 meters long—to handle the high-volume, low-density steam, often in dual-flow designs to split the exhaust and reduce axial thrust. They’re the backbone of large power plants, like a 1,200 MW nuclear unit, but require extensive cooling infrastructure, adding complexity and environmental considerations like water usage or thermal pollution.
Back-pressure turbines, on the other hand, exhaust steam at a higher pressure, such as 5 to 20 bar, so it can be used for industrial processes like heating, drying, or driving other equipment. Found in factories like paper mills or chemical plants, they sacrifice some electrical efficiency—since less energy is extracted as work—but excel in combined heat and power setups, where total energy efficiency can reach 80% or more when heat is factored in. They’re simpler than condensing turbines, lacking a condenser, and their exhaust pressure is tailored to downstream needs, making them economical for specific industries.
Extraction turbines offer flexibility by allowing steam to be tapped at intermediate stages for industrial use, while the rest continues through the turbine to generate power. For example, a refinery might extract steam at 15 bar for heating and 2 bar for feedwater preheating, with the remainder condensing for electricity. Control valves adjust the extraction flow, and the turbine’s design accounts for reduced steam volume in later stages, balancing power and process demands. These are common in facilities needing both electricity and variable steam supplies, like steel mills or district heating systems.
Reheat turbines improve efficiency by interrupting the expansion process. After passing through the high-pressure stage, steam—now at 20-50 bar—returns to the boiler to be reheated to its original temperature, say 540°C, before entering intermediate- and low-pressure stages. This increases the cycle’s average heat-addition temperature, boosting efficiency by 4-5% for a single reheat, or up to 7% with double reheat in ultra-efficient plants. Reheat designs are standard in large fossil-fuel plants, requiring extra piping and moisture separators to handle wetter steam in later stages, but the added complexity pays off in fuel savings.
In large power plants, turbines are often split into high-pressure, intermediate-pressure, and low-pressure sections, each optimized for its steam conditions and mounted on a single shaft. High-pressure turbines have small, robust blades to handle extreme conditions—up to 300 bar and 600°C—using materials like chromium-steel alloys to resist creep and corrosion. Intermediate-pressure turbines manage reheated steam at 20-50 bar, with mid-sized blades, while low-pressure turbines feature massive blades—up to 2 meters long—to process low-pressure, high-volume steam, often in twin-flow layouts. This segmentation is standard in gigawatt-scale plants, simplifying maintenance and maximizing efficiency.
The history of steam turbines begins in the late 19th century. Sir Charles Parsons built the first practical multi-stage reaction turbine in 1884, generating 7.5 kW to power a ship’s lights, proving the concept’s potential. Around the same time, Gustaf de Laval developed his impulse turbine, focusing on high-speed applications. By 1900, turbines outpaced reciprocating steam engines in power generation, offering higher efficiency and scalability. Early 20th-century advances in metallurgy—introducing heat-resistant steels—allowed turbines to handle higher temperatures and pressures, scaling to megawatts and driving the electrification of cities. During World War II, steam turbines powered massive ships like the Titanic, with 29,000 horsepower, and continued evolving with innovations like reheat cycles and supercritical steam in the mid-20th century.
Steam turbines have a vast range of applications. In power generation, they produce over 80% of the world’s electricity—37% from coal, 25% from gas in combined-cycle plants, 10% from nuclear, and smaller shares from biomass, geothermal, and solar thermal. Industrially, they drive compressors in chemical plants, pumps in oil refineries, and provide process steam in paper production. Historically, they propelled naval vessels and merchant ships until gas turbines took over post-war. Today, they’re key to renewable energy, powering biomass plants burning wood or agricultural waste, and concentrated solar power systems where mirrors heat a fluid to generate steam.
Design-wise, steam turbines are engineering marvels. Blades vary by stage—high-pressure blades are small, thick, and made of nickel-based superalloys like Inconel 718 to withstand 600°C and resist creep, while low-pressure blades are long, thin, and often titanium to combat erosion from wet steam droplets. Casings are forged steel or alloys, designed to contain high pressures and temperatures, with labyrinth or brush seals minimizing steam leakage around the rotor. The rotor itself, a massive steel forging, must be perfectly balanced to spin at thousands of RPM without vibration, expanding and contracting with heat. Challenges include creep deformation at high temperatures, blade erosion from moisture, and fatigue from years of cyclic loading, driving ongoing material research into ceramics and coatings.
Efficiency in steam turbines hinges on steam conditions and design. A basic system might convert 20-30% of heat into work, losing the rest to exhaust and friction. Advanced plants with supercritical steam—600°C, 300 bar—reach 45-47%, nearing the Rankine cycle’s ideal limit of around 60%. Losses come from heat escaping in the exhaust, turbulence in blade gaps, and moisture in low-pressure stages, which reduces energy output unless countered by superheating or moisture separators. Innovations like reheat cycles, where steam is reheated mid-process, and precise blade profiles designed with computational fluid dynamics, push performance higher.
Steam turbines remain critical today. In fossil-fuel plants, they’re the workhorse for coal and gas, though coal’s role shrinks with climate goals. Nuclear power relies on them to convert reactor heat into electricity, with huge low-pressure turbines handling saturated steam from boiling water reactors. In renewables, they power biomass plants—burning wood or waste—and geothermal facilities, while concentrated solar power uses them to turn sunlight into electricity. Modern advancements include ceramic blades tolerating 1,000°C, 3D-printed components for complex shapes, and AI-driven controls optimizing performance in real time, ensuring turbines stay relevant in a shifting energy landscape.
The advantages of steam turbines are numerous. They deliver massive power—up to 1,750 MW in nuclear units like the Arabelle turbine—run reliably for 30-50 years with maintenance, and adapt to any heat source, from fossil fuels to renewables. However, they’re not without drawbacks. They require complex infrastructure—boilers, condensers, cooling systems—take hours to start up compared to minutes for gas turbines, and need water for cooling, posing challenges in arid regions or with environmental regulations.
A standout example of their scale is the Arabelle turbine, used in French nuclear plants, producing 1,750 MW with low-pressure blades over 2 meters long, powering millions of homes. On a smaller scale, a wood-fired turbine might generate 5 kW for a cabin, burning a few kilograms of wood per hour. From these extremes, steam turbines showcase their versatility and enduring impact. Since Parsons’ first design, they’ve evolved from kilowatts to gigawatts, shaping how we generate power and likely to persist as we balance fossil fuels with sustainable energy.
Steam turbines are remarkable machines that have played a pivotal role in shaping the modern world by converting thermal energy from steam into mechanical work, primarily for generating electricity or driving industrial equipment. Their ability to harness the power of steam—produced by heating water with fuels like coal, gas, nuclear energy, biomass, or even solar heat—makes them one of the most versatile and widely used technologies in energy production. With a history stretching back to the late 19th century, steam turbines have evolved from simple experimental devices into highly efficient, complex systems that power everything from small workshops to entire cities. Let’s continue exploring their mechanics, variations, historical significance, technical details, applications, and their place in today’s energy landscape, diving deeper into each aspect.
The operation of a steam turbine is rooted in the Rankine cycle, a thermodynamic process that efficiently turns heat into usable energy. In a boiler, water is heated under pressure until it becomes steam, reaching temperatures between 200 and 600 degrees Celsius and pressures from 10 to 300 bar, depending on the system’s design and purpose. For example, a small biomass boiler might produce steam at 10 bar and 200°C, while a supercritical coal plant pushes conditions to 300 bar and 600°C for maximum efficiency. This high-pressure steam enters the turbine and flows over blades attached to a rotor—a large, cylindrical shaft. As the steam expands, its pressure and temperature drop, accelerating it into the blades and causing the rotor to spin. The rotor’s speed varies—1,500 RPM for 50 Hz grids, 3,600 RPM for 60 Hz, or even 30,000 RPM in high-speed impulse designs—driving a generator that converts the motion into electricity. After doing its work, the steam exits the turbine, either condensing into water in a closed-loop system or leaving as usable heat for industrial processes.
The blades are the heart of the turbine, and their interaction with steam defines the turbine’s type. In impulse turbines, steam passes through stationary nozzles that accelerate it into high-velocity jets—sometimes exceeding 500 meters per second—before striking curved, bucket-like blades on the rotor. The pressure drop occurs entirely in the nozzles, so the blades only convert kinetic energy into motion. Gustaf de Laval’s single-stage turbine, developed in the 1880s, is a classic impulse design, spinning at extreme speeds like 30,000 RPM to power small generators or pumps. Its simplicity made it a breakthrough, though its efficiency drops with larger steam volumes. The Curtis turbine, another impulse variant, adds velocity compounding—steam bounces between multiple rows of moving and stationary blades, extracting energy step-by-step, reducing speed to 3,000-6,000 RPM for practical use in early 20th-century power plants. Impulse turbines are rugged and ideal for high-pressure steam, but they struggle with lower-pressure conditions or fluctuating loads.
Reaction turbines take a different approach, extracting energy through continuous steam expansion across both fixed and moving blades. Fixed stator blades direct steam onto moving rotor blades, where it accelerates and drops in pressure, creating a reactive force—like a rocket’s thrust—that spins the rotor. Sir Charles Parsons pioneered this in 1884 with a multi-stage design, using dozens or even hundreds of stages to gradually extract energy. Each stage contributes a small pressure drop, with blade sizes growing from a few centimeters in high-pressure sections to over a meter in low-pressure ones, matching the steam’s expansion. The blades are airfoil-shaped, precision-crafted to minimize turbulence, and require tight tolerances—gaps as small as a millimeter—to prevent steam leakage. Reaction turbines shine in large power plants, achieving efficiencies up to 90% of the ideal Rankine cycle, but their complexity and cost make them less practical for small-scale use.
Combination turbines blend these principles for versatility. In the high-pressure stages, an impulse design handles the intense initial conditions—say, 300 bar and 600°C—with nozzles and sturdy blades, while later stages shift to reaction designs as the steam expands to 20 bar or less, optimizing energy extraction across the pressure spectrum. This hybrid setup is common in modern supercritical plants, where steam pushes material limits. Designers use computational tools to smooth the transition, ensuring minimal shock losses as steam behavior changes, making these turbines efficient and compact for utility-scale power generation.
Condensing turbines focus on maximizing power by exhausting steam into a vacuum, typically 0.03 to 0.1 bar, created by a condenser. This low pressure lets the steam expand further, extracting nearly all its energy. The low-pressure stages feature enormous blades—sometimes 1.5 to 2 meters long—spinning at tip speeds near 500 m/s, often in dual-flow configurations where steam splits to opposite sides of the turbine to balance thrust. Condensers use water from rivers or cooling towers, evaporating 1-2% of a plant’s output as waste heat, or air in water-scarce areas, though air-cooling reduces efficiency. These turbines power massive plants—coal, nuclear, or gas-fired—delivering hundreds of megawatts, but they need extensive cooling systems, raising costs and environmental concerns like thermal discharge into waterways.
Back-pressure turbines prioritize dual outputs, exhausting steam at 5 to 20 bar for industrial use—think heating pulp in a paper mill or driving a chemical reactor—rather than condensing it. The turbine’s final stage is tuned to this pressure, with fewer blades than a condensing design, and the exhaust steam retains significant heat, boosting total energy efficiency to 80% or more in combined heat and power systems. They’re simpler, skipping the condenser, and cost-effective where heat is as valuable as power, though their electrical efficiency is lower since less steam energy becomes work.
Extraction turbines add flexibility, tapping steam at intermediate points—say, 15 bar for process heat and 2 bar for boiler feedwater heating—while the rest generates power. Control valves regulate extraction, and the turbine’s staging adjusts for reduced flow in later sections, requiring sophisticated engineering to balance outputs. These are ideal for refineries or district heating, where steam demands shift, offering adaptability at the cost of added complexity.
Reheat turbines enhance efficiency by reheating steam mid-process. After the high-pressure stage drops steam to 20-50 bar, it returns to the boiler, reheating to 540-600°C, then expands through intermediate- and low-pressure stages. This raises the cycle’s average temperature, lifting efficiency by 4-5% for one reheat or 7% for two, though double-reheat adds cost and rarely justifies itself outside ultra-efficient plants. Reheat systems need extra piping and moisture separators—wet steam after reheating erodes blades—making them standard in large fossil-fuel stations chasing every percentage point of fuel savings.
Large turbines often split into high-pressure, intermediate-pressure, and low-pressure sections on one shaft, each tailored to its steam range. High-pressure turbines use small, thick blades—10-20 cm long—to handle 300 bar and 600°C, made of nickel alloys like Inconel to resist creep and corrosion. Intermediate-pressure turbines, with mid-sized blades, manage reheated steam at 20-50 bar, while low-pressure turbines have huge blades—up to 2 meters—to process low-pressure, high-volume steam, often splitting flow to twin exhausts. This modular design dominates gigawatt-scale plants, simplifying repairs and optimizing performance across the steam’s journey.
Historically, steam turbines emerged during the Second Industrial Revolution. Parsons’ 1884 reaction turbine, generating 7.5 kW, powered a ship’s lights, proving multi-stage efficiency. De Laval’s impulse design, from the same era, targeted high-speed niches. By 1900, turbines outpaced steam engines, scaling to megawatts with better steels and boiler tech. The 20th century saw them power ships—the Titanic’s 29,000 hp turbines drove it across the Atlantic—and electrify nations, with innovations like reheat cycles (1930s) and supercritical steam (1950s) pushing efficiency from 20% to over 45%.
Applications span industries. In power generation, steam turbines produce over 80% of global electricity—37% from coal, 25% from gas, 10% from nuclear, plus biomass, geothermal, and solar thermal. Industrially, they run compressors in chemical plants, pumps in oil fields, and supply steam in paper mills. They once dominated marine propulsion, powering warships and liners until gas turbines took over post-1940s. Today, they’re key to renewables—biomass plants burn wood or waste, while solar thermal systems use mirrors to heat steam—bridging fossil and green energy.
Design details reveal their complexity. High-pressure blades, small and robust, use superalloys like Inconel 718 or Rene 41, tolerating 600°C for decades, with chromium coatings fighting corrosion. Low-pressure blades, long and thin, use titanium to resist wet-steam erosion, with tips moving at supersonic speeds. Casings, forged from steel or alloys, contain pressures up to 300 bar, with labyrinth seals—layered grooves—cutting leakage. Rotors, weighing tons, are precision-balanced to spin at 3,000 RPM without vibration, expanding inches from heat. Challenges include creep (slow deformation at high heat), erosion (wet steam pitting blades), and fatigue (cracks from cyclic stress), driving research into ceramics, which handle 1,000°C, and protective coatings.
Efficiency varies widely. Small turbines hit 20-30%, losing heat to exhaust and friction. Advanced plants with supercritical steam—600°C, 300 bar—reach 45-47%, nearing the Rankine ideal of 60%. Losses stem from exhaust heat, blade-gap leaks, and moisture in low-pressure stages, which drops output unless mitigated by superheating to 540°C or separators removing water droplets. Reheat cycles, supercritical conditions, and CFD-optimized blades—curved for minimal turbulence—push performance, with top plants achieving over 90% of theoretical efficiency.
Today, steam turbines remain vital. Fossil-fuel plants—coal and gas—rely on them, though coal declines with emissions goals. Nuclear plants use them to turn reactor heat into power, with massive low-pressure turbines handling saturated steam at 70 bar. Renewables lean on them—biomass burns wood for 1-50 MW, geothermal taps underground steam, and solar thermal generates steam from sunlight. Innovations keep them competitive: ceramic blades for higher heat, 3D-printed parts for precision, and AI controls for real-time optimization, ensuring they adapt to a decarbonizing world.
Their strengths are clear—massive output (1,750 MW in nuclear units), longevity (30-50 years), and fuel flexibility. But they’re not perfect—complex setups with boilers and condensers, slow startups (hours vs. minutes for gas turbines), and water-heavy cooling limit them in dry areas or fast-response grids. The Arabelle turbine, at 1,750 MW, powers 2 million homes with 2-meter blades, while a 5 kW wood-fired unit lights a cabin—proof of their range. From Parsons’ 7.5 kW to today’s giants, steam turbines have electrified the world and will evolve as we balance legacy fuels with sustainability.
Industrial Steam Turbine
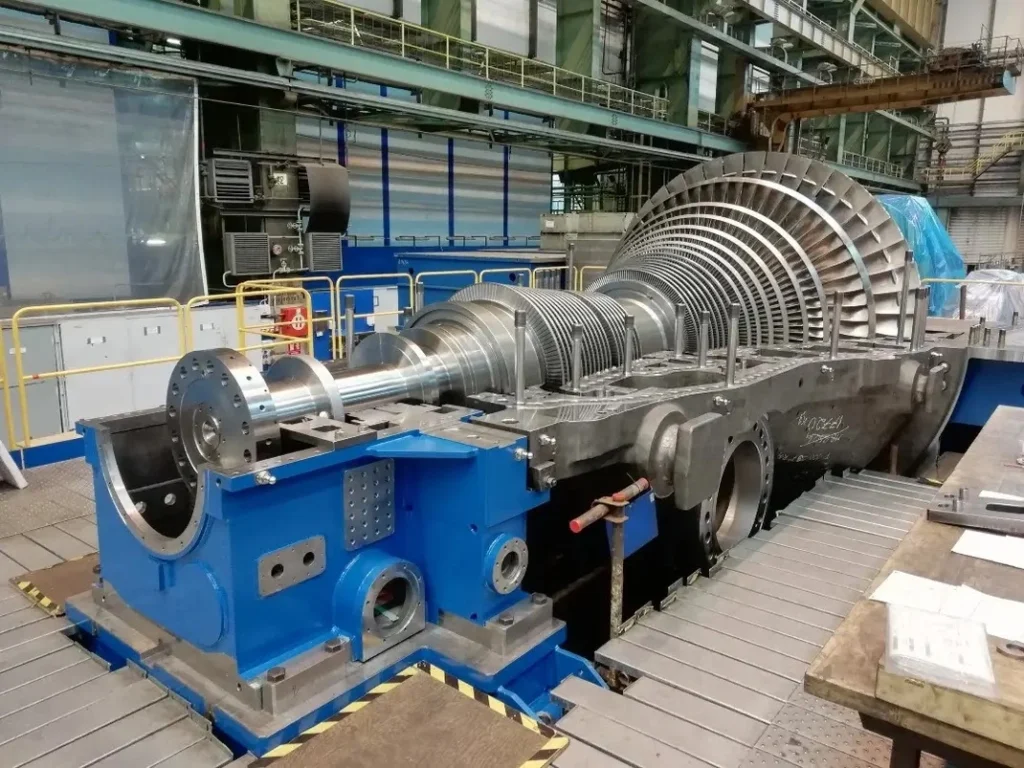
Industrial steam turbines are a specialized subset of steam turbines designed specifically for use in industrial settings, where they generate electricity, drive machinery, or provide process steam for manufacturing and production processes. Unlike utility-scale turbines found in large power plants, which focus on maximizing electrical output for the grid, industrial steam turbines are tailored to meet the unique energy demands of factories, refineries, chemical plants, and other industrial facilities. They prioritize flexibility, efficiency in combined heat and power (CHP) applications, and integration with industrial processes, often operating at smaller scales—ranging from a few kilowatts to tens of megawatts—while still leveraging the same fundamental principles as their larger counterparts. Below is a detailed exploration of industrial steam turbines, covering their mechanics, types, applications, design features, and significance in modern industry.
Industrial steam turbines work by converting thermal energy from steam into mechanical energy, typically within the framework of the Rankine cycle. Steam is produced in a boiler heated by fuels like natural gas, coal, biomass (such as wood or agricultural waste), oil, or waste heat from industrial processes. The steam, pressurized to between 5 and 100 bar and heated to 200-540°C, enters the turbine and expands across a series of blades mounted on a rotor. This expansion drives the rotor, spinning it at speeds like 3,000 RPM (for 50 Hz systems) or 3,600 RPM (60 Hz), which then powers a generator for electricity or directly drives equipment such as pumps, compressors, or fans. The steam’s exit path depends on the turbine’s purpose—some exhaust into a condenser for maximum power, while others release steam at usable pressures (e.g., 2-20 bar) for heating or process use, a hallmark of industrial applications.
The types of industrial steam turbines reflect their adaptability to factory needs. Back-pressure turbines are common, exhausting steam at a higher pressure—say, 5-20 bar—rather than condensing it, so the exhaust can heat buildings, dry materials, or power downstream processes. For example, in a paper mill, a back-pressure turbine might generate 5 MW of electricity while supplying 10 tons/hour of 10-bar steam to dry pulp, achieving total energy efficiency above 80% when heat is included, though electrical efficiency alone might be 20-30%. Extraction turbines offer more versatility, allowing steam to be tapped at intermediate stages—perhaps 15 bar for a chemical reactor and 3 bar for preheating—while the rest generates power. These turbines use control valves to adjust extraction flow, balancing electricity and steam output, making them ideal for refineries or food processing plants with variable demands.
Condensing turbines, though less frequent in industrial settings, are used when electricity is the primary goal. They exhaust steam into a vacuum (0.05-0.1 bar) via a condenser, maximizing energy extraction with efficiencies up to 35-40%. A small factory might use a 1 MW condensing turbine to power its grid, condensing steam with a water-cooled system tied to a cooling tower. Combination turbines, blending impulse and reaction designs, also appear in larger industrial setups—impulse stages handle high-pressure steam (e.g., 50 bar), transitioning to reaction stages for lower pressures, optimizing performance in plants with diverse steam conditions. Single-stage turbines, simple and compact, suit small applications like a 50 kW unit driving a pump, while multi-stage designs dominate in bigger systems needing higher efficiency, such as a 10 MW turbine in a steel mill.
Applications of industrial steam turbines are vast and tied to specific industries. In chemical plants, they generate power (e.g., 2-20 MW) while supplying steam for reactions or distillation, often using extraction turbines to match process needs. Pulp and paper mills rely on back-pressure turbines, producing 5-15 MW of electricity and steam at 5-10 bar to dry paper, leveraging wood waste as fuel for sustainability. Oil refineries use extraction or condensing turbines, delivering 10-50 MW and steam for cracking or heating, often fueled by refinery byproducts. Sugar mills burn bagasse (cane waste) to power turbines—typically 1-5 MW—while heating juice evaporators. Textile factories use small turbines (100 kW-1 MW) for power and steam dyeing, and food processing plants like breweries employ CHP turbines for electricity and sterilization heat. Mining operations drive compressors or pumps with turbines, often in remote areas using diesel or biomass.
Design features of industrial steam turbines reflect their practical demands. They’re typically smaller than utility turbines—ranging from 50 kW to 50 MW—making them compact enough to fit within factory footprints. Blades vary by stage: high-pressure blades, 5-20 cm long, use nickel alloys like Inconel to handle 540°C and 100 bar, resisting creep and corrosion, while low-pressure blades, up to 1 meter, might use stainless steel or titanium for durability against wet steam. Rotors, forged from steel, spin at 3,000-10,000 RPM, balanced to minimize vibration, with couplings or gearboxes linking to machinery. Casings, made of cast steel, withstand pressures up to 100 bar, with seals like labyrinth designs reducing steam leaks. Boilers are often integrated, sized for 1-100 tons/hour of steam, and fuel flexibility—natural gas, biomass, or waste heat—lets them adapt to local resources. Control systems, increasingly digital, adjust steam flow and extraction in real time, optimizing for shifting loads.
Efficiency in industrial steam turbines depends on their role. Electrical efficiency ranges from 20-40%, lower than utility turbines (45%+), due to smaller scale and heat-focused designs. However, in CHP setups, total efficiency—power plus usable heat—can hit 70-85%, far surpassing condensing-only systems. For instance, a 5 MW back-pressure turbine might convert 25% of fuel energy to electricity and 50% to process steam, losing only 25% to waste heat. Losses come from exhaust heat, friction, and blade-gap leaks, mitigated by superheating steam to 540°C or using moisture separators to dry low-pressure steam. Multi-stage designs with 5-20 stages extract energy gradually, boosting efficiency over single-stage units, though at higher cost.
Historically, industrial steam turbines evolved alongside the Industrial Revolution. Early 20th-century factories used steam engines, but by the 1910s, turbines—building on Parsons’ and De Laval’s work—offered better efficiency and power density. World War II saw them power factories and ships, often fueled by coal or oil. Post-war, they adapted to industrial CHP, with the 1970s energy crisis spurring biomass and waste-heat use. Today, they’re modernizing with automation and sustainable fuels, reflecting industry’s push for efficiency and lower emissions.
Their significance in modern industry is profound. They provide on-site power, reducing grid reliance and costs—critical in remote or unstable regions. CHP capability slashes energy bills; a 10 MW turbine might save a factory $1-2 million yearly by reusing steam. Fuel flexibility supports sustainability—biomass turbines burn wood chips or sawdust, cutting fossil fuel use, while waste-heat recovery harnesses exhaust from furnaces or engines. In a steel plant, a 20 MW turbine might use blast furnace gas, generating power and steam without extra fuel. Reliability is key—turbines run 20-30 years with maintenance, handling 24/7 loads in harsh conditions.
Challenges exist. Initial costs are high—a 5 MW system might run $2-5 million, including boiler and installation—though payback comes in 3-7 years with CHP savings. Maintenance involves blade inspections, seal replacements, and boiler cleaning, costing $50,000-$100,000 yearly for a mid-sized unit. Emissions—CO2, particulates—require scrubbers or filters to meet regulations, especially with biomass or coal. Space needs—boilers, turbines, condensers—limit use in cramped facilities, and startup times (30 minutes to hours) lag behind gas turbines’ responsiveness.
Examples highlight their range. A 2 MW extraction turbine in a chemical plant burns natural gas, producing 1.5 MW electricity and 5 tons/hour of 10-bar steam for reactors, with a $1 million setup cost offset by $300,000 annual savings. A 500 kW back-pressure turbine in a sawmill uses wood waste, powering tools and drying lumber, costing $200,000 but paying back in two years. A 10 MW condensing turbine in a refinery, fueled by oil residues, runs at 35% efficiency, powering pumps and compressors with a water-cooled condenser.
Industrial steam turbines bridge power and process needs, offering tailored solutions where utility turbines don’t fit. Their adaptability—handling diverse fuels, scales, and outputs—keeps them vital in manufacturing, from small workshops to sprawling plants. As industries chase efficiency and sustainability, these turbines evolve with smarter controls, tougher materials, and greener fuels, ensuring they remain a workhorse of industrial energy.
Industrial steam turbines are robust, versatile machines engineered to meet the specific energy demands of industrial facilities, converting steam’s thermal energy into mechanical work for electricity generation, machinery operation, or process steam supply. Unlike their utility-scale counterparts focused on massive grid power, industrial steam turbines are designed for flexibility, often prioritizing combined heat and power (CHP) efficiency and integration with manufacturing processes. Found in factories, refineries, and mills, they range from compact units producing a few kilowatts to larger systems delivering tens of megawatts, balancing power needs with industrial heat requirements. Let’s delve deeper into their mechanics, types, applications, design intricacies, historical context, modern role, and operational details, expanding on their significance in industry.
The core operation of an industrial steam turbine follows the Rankine cycle, a thermodynamic process tailored to industrial settings. A boiler generates steam by burning fuels like natural gas, coal, oil, biomass (e.g., wood chips, bagasse), or capturing waste heat from processes like furnace exhausts. Steam conditions vary—small systems might produce 5-20 bar at 200-300°C, while larger ones reach 50-100 bar at 500-540°C, depending on efficiency goals and fuel quality. This steam enters the turbine, flowing over blades fixed to a rotor. As it expands, its pressure drops—say, from 50 bar to 5 bar—accelerating across the blades and spinning the rotor at speeds like 3,000 RPM (50 Hz grids), 3,600 RPM (60 Hz), or higher (up to 10,000 RPM) in smaller, high-speed units. The rotor drives a generator for electricity or directly powers equipment—pumps, fans, compressors—via a shaft or gearbox. Exiting steam either condenses in a vacuum (0.05-0.1 bar) for maximum power or leaves at usable pressures (2-20 bar) for heating, drying, or chemical reactions, a key feature in industrial CHP systems.
Types of industrial steam turbines are defined by their steam handling and output priorities. Back-pressure turbines exhaust steam at elevated pressures—typically 5-20 bar—rather than condensing it, supplying heat for industrial tasks. In a textile plant, a 3 MW back-pressure turbine might burn biomass, generating 2.5 MW of electricity while providing 8 tons/hour of 10-bar steam to dye fabrics, achieving 75-85% total efficiency by reusing heat, though electrical efficiency stays at 20-30%. Extraction turbines add control, tapping steam at multiple stages—e.g., 15 bar for a distillation column, 3 bar for boiler feedwater heating—while the rest powers the rotor. A 10 MW extraction turbine in a refinery could produce 8 MW and extract 20 tons/hour of steam at two pressures, using valves to adjust flow based on demand, ideal for fluctuating process needs. Condensing turbines, less common industrially, focus on power, exhausting into a condenser’s vacuum to hit 35-40% electrical efficiency. A 5 MW condensing turbine in a mining operation might power compressors, using a water-cooled condenser tied to a nearby stream.
Combination turbines, mixing impulse and reaction designs, suit larger industrial systems. High-pressure stages (e.g., 50 bar) use impulse blades—steam jets from nozzles onto buckets—while lower-pressure stages (e.g., 10 bar) shift to reaction blades, where steam expands across moving blades for efficiency. A 15 MW combination turbine in a chemical plant might handle 540°C steam, optimizing energy extraction across stages. Single-stage turbines, with one blade set, are compact and simple—think a 100 kW unit driving a pump in a small factory—while multi-stage turbines, with 5-20 stages, boost efficiency in bigger setups like a 20 MW sugar mill turbine. Reheat turbines, rare industrially due to cost, reheat steam mid-process (e.g., from 20 bar back to 540°C), lifting efficiency by 4-5%, used in high-output plants like steelworks needing every edge.
Applications tie directly to industrial sectors. Chemical plants use 2-20 MW turbines for power and steam—e.g., a 5 MW extraction unit supplies 10-bar steam for reactors while running agitators. Pulp and paper mills favor back-pressure turbines—say, a 10 MW unit burning wood waste, producing 8 MW and 15 tons/hour of 5-bar steam to dry paper. Oil refineries deploy 10-50 MW turbines, often extraction or condensing, using byproduct fuels like petroleum coke to power pumps and supply steam for cracking. Sugar mills burn bagasse for 1-5 MW turbines, driving mills and heating evaporators—e.g., a 3 MW unit processes 500 tons of cane daily. Textile plants use 100 kW-1 MW turbines for power and steam dyeing, while food processors like dairies run 500 kW-2 MW CHP turbines for sterilization and electricity. Mining sites power compressors or hoists with 1-10 MW turbines, often in remote areas using diesel or local biomass.
Design specifics reflect industrial practicality. Sizes range from 50 kW to 50 MW—smaller than utility turbines (100 MW+)—fitting tight factory spaces. High-pressure blades, 5-20 cm long, use nickel-based superalloys (e.g., Inconel 718) to handle 540°C and 100 bar, resisting creep and oxidation, often coated with chromium carbide for durability. Low-pressure blades, up to 1 meter, use stainless steel or titanium to combat wet-steam erosion, with tips moving at 300-500 m/s. Rotors, forged from high-strength steel, weigh tons yet spin precisely, balanced to avoid vibration, with couplings or gearboxes (e.g., reducing 10,000 RPM to 1,500 RPM) linking to loads. Casings, cast steel or alloys, contain pressures up to 100 bar, with labyrinth seals—concentric grooves—cutting leakage by 5-10%. Boilers, producing 1-100 tons/hour of steam, integrate with turbines, burning diverse fuels—natural gas (clean, efficient), biomass (sustainable), or waste heat (free energy). Digital controls monitor steam flow, pressure, and extraction, adjusting in seconds for optimal output.
Efficiency balances power and heat. Electrical efficiency spans 20-40%—a 5 MW condensing turbine might hit 35%, converting 35% of fuel energy to power, losing 65% to exhaust and friction. CHP systems shine, with back-pressure or extraction turbines reaching 70-85% total efficiency—e.g., a 3 MW unit turns 25% into electricity, 50% into usable steam, losing 25% to waste. Losses stem from exhaust heat, blade turbulence, and moisture—wet steam in low-pressure stages cuts output by 5-10% unless superheated to 540°C or dried with separators. Multi-stage designs extract energy gradually—5-20 stages vs. 1—lifting efficiency by 10-15% over single-stage units, though complexity rises. Fuel quality matters—dry biomass (4.5 kWh/kg) outperforms wet wood (3 kWh/kg), reducing steam output if moisture exceeds 20%.
Historically, industrial steam turbines grew from late 19th-century innovations. Parsons’ 1884 reaction turbine and De Laval’s impulse design laid the groundwork, but by the 1910s, factories adopted turbines over steam engines for higher efficiency—10-20% vs. 5-10%. Early 20th-century mills used coal-fired turbines for power and heat, scaling to megawatts by the 1930s. WWII spurred industrial use—turbines ran factories on coal or oil when grids faltered. The 1970s energy crisis boosted CHP and biomass turbines, cutting costs as oil prices soared. Today, they align with sustainability—waste-heat turbines in steelworks or biomass units in sawmills reflect greener priorities.
Their modern role is critical. On-site power cuts grid dependence—a 5 MW turbine saves a factory $500,000-$1 million yearly vs. utility rates. CHP slashes energy costs—a 10 MW unit might recover $2 million annually in heat value. Sustainability drives adoption—biomass turbines burn wood waste (1-2 tons/hour for 5 MW), reducing fossil fuel use, while waste-heat units harness kiln exhaust, needing no extra fuel. Reliability stands out—turbines run 20-30 years, handling 8,000 hours/year in harsh conditions like dusty mines or humid mills. A 15 MW turbine in a petrochemical plant might use flare gas, producing power and steam without added emissions.
Challenges persist. Costs are steep—a 5 MW system with boiler and piping runs $2-5 million, though CHP payback hits 3-5 years. Maintenance—blade checks, seal swaps, boiler descaling—costs $50,000-$150,000 yearly, with downtime cutting output. Emissions require controls—biomass emits 50-200 mg/m³ of particulates, needing $100,000 scrubbers for compliance (e.g., U.S. EPA limits at 25 mg/m³). Space demands—10×20 meters for a 5 MW setup—clash with cramped plants, and startups take 30-60 minutes, lagging gas turbines’ 5-10 minutes. Fuel supply matters—wet biomass or inconsistent waste heat drops performance.
Examples illustrate their impact. A 1 MW back-pressure turbine in a dairy burns gas, producing 800 kW and 3 tons/hour of 5-bar steam for pasteurization, costing $500,000 but saving $150,000 yearly. A 20 MW extraction turbine in a steel mill uses blast furnace gas, delivering 15 MW and 30 tons/hour of 15-bar steam for rolling, with a $10 million cost offset by $3 million annual savings. A 200 kW condensing turbine in a remote mine, diesel-fueled, powers drills at 35% efficiency, costing $100,000 but cutting generator fuel by 50%.
Industrial steam turbines are industrial energy’s unsung heroes, blending power and heat with unmatched flexibility. Their evolution—from coal-fired mills to biomass-driven plants—mirrors industry’s shift to efficiency and sustainability. With smart controls, tough materials, and green fuels, they’ll keep factories humming, proving their worth in a changing world.
Industrial steam turbines are highly specialized machines engineered to meet the diverse energy needs of industrial environments, transforming the thermal energy of steam into mechanical work to generate electricity, power machinery, or supply process steam for manufacturing. Unlike utility-scale turbines that dominate large power plants with outputs in the hundreds of megawatts, industrial steam turbines are typically smaller—ranging from tens of kilowatts to 50 megawatts—and are designed for flexibility, often prioritizing combined heat and power (CHP) efficiency over raw electrical output. They serve as critical assets in factories, refineries, mills, and mines, integrating seamlessly with industrial processes to provide on-site power and heat. Let’s expand further on their mechanics, types, applications, design details, historical evolution, modern significance, and operational nuances, diving deeper into their role in industry.
The operation of an industrial steam turbine is based on the Rankine cycle, adapted to industrial demands. Steam is generated in a boiler using fuels such as natural gas, coal, oil, biomass like wood chips or bagasse, or waste heat from industrial processes—think exhaust from a cement kiln or refinery flare gas. Steam conditions vary widely: a small system might produce 5-20 bar at 200-300°C, suitable for a food processing plant, while a larger one reaches 50-100 bar at 500-540°C, common in chemical manufacturing. This steam enters the turbine, flowing over blades attached to a rotor—a heavy steel shaft. As it expands—dropping from, say, 50 bar to 5 bar—it accelerates, pushing the blades and spinning the rotor at speeds like 3,000 RPM for 50 Hz electrical grids, 3,600 RPM for 60 Hz, or up to 10,000 RPM in high-speed industrial units. The rotor’s motion drives a generator for electricity or directly powers equipment—pumps, compressors, fans—via a shaft, often with a gearbox to match equipment speeds. Exiting steam either condenses into a vacuum (0.05-0.1 bar) for maximum power or leaves at usable pressures (2-20 bar) for heating or process use, a defining trait of industrial turbines in CHP setups.
Types of industrial steam turbines are tailored to specific industrial roles. Back-pressure turbines exhaust steam at higher pressures—typically 5-20 bar—rather than condensing it, supplying heat for tasks like drying lumber or heating chemical vats. A 5 MW back-pressure turbine in a paper mill might burn wood waste, producing 4 MW of electricity and 10 tons/hour of 10-bar steam, hitting 80% total efficiency by reusing heat, though electrical efficiency is 25-30%. Extraction turbines provide more control, tapping steam at multiple points—e.g., 15 bar for a distillation tower, 3 bar for preheating boiler water—while the rest generates power. A 10 MW extraction turbine in a petrochemical plant could deliver 7 MW and 15 tons/hour of steam at two pressures, using automated valves to adjust flow, perfect for variable process demands. Condensing turbines, less common in industry, exhaust into a condenser’s vacuum, maximizing power at 35-40% efficiency. A 3 MW condensing turbine in a mining camp might power lighting and drills, using a water-cooled condenser tied to a local river.
Combination turbines mix impulse and reaction designs for larger systems. High-pressure stages (e.g., 50 bar, 540°C) use impulse blades—steam jets from nozzles onto buckets—while lower-pressure stages (e.g., 10 bar) shift to reaction blades, where steam expands across moving blades, boosting efficiency. A 20 MW combination turbine in a steel mill might optimize steam from blast furnace gas across 10 stages. Single-stage turbines, with one blade set, are simple and compact—say, a 200 kW unit driving a fan—while multi-stage turbines, with 5-20 stages, enhance efficiency in bigger setups like a 15 MW refinery turbine. Reheat turbines, though rare industrially, reheat steam mid-process—e.g., from 20 bar back to 540°C—adding 4-5% efficiency, used in high-value plants like fertilizer production needing top performance.
Applications span key industries. Chemical plants use 2-20 MW turbines for power and steam—e.g., a 5 MW extraction unit supplies 12-bar steam for reactors while running pumps. Pulp and paper mills rely on back-pressure turbines—say, a 10 MW unit burning sawdust, producing 8 MW and 20 tons/hour of 5-bar steam to dry pulp. Oil refineries deploy 10-50 MW turbines, often extraction or condensing, using byproduct fuels like heavy oil to power compressors and supply steam for cracking. Sugar mills burn bagasse for 1-5 MW turbines—e.g., a 2 MW unit processes 400 tons of cane daily, heating evaporators. Textile plants use 100 kW-1 MW turbines for power and steam dyeing, while food processors like breweries run 500 kW-2 MW CHP turbines for bottling and sterilization. Mining operations power hoists or ventilation with 1-10 MW turbines, often in remote sites using diesel or local wood.
Design details highlight their industrial focus. Sizes range from 50 kW to 50 MW, compact enough for factory floors—e.g., a 5 MW unit fits in a 10×20-meter space. High-pressure blades, 5-20 cm long, use nickel alloys like Inconel 718 or Hastelloy, handling 540°C and 100 bar, resisting creep and oxidation, often coated with ceramic or chromium for longevity. Low-pressure blades, up to 1 meter, use stainless steel or titanium, with tips at 300-500 m/s resisting wet-steam erosion. Rotors, forged from high-strength steel, weigh 1-10 tons, balanced to spin at 3,000-10,000 RPM, with couplings or gearboxes (e.g., 5:1 ratio) matching equipment speeds. Casings, cast steel or alloys, contain 100 bar, with labyrinth seals reducing leakage by 5-10%. Boilers, producing 1-100 tons/hour, integrate tightly—natural gas offers clean burns, biomass leverages waste, waste heat taps free energy. Digital controls—PLCs or SCADA—monitor pressure, temperature, and flow, adjusting in milliseconds for load shifts.
Efficiency balances electrical and thermal output. Electrical efficiency ranges from 20-40%—a 5 MW condensing turbine hits 35%, losing 65% to exhaust and friction, while CHP systems like back-pressure turbines reach 70-85% total efficiency. A 3 MW unit might convert 25% to power, 50% to 10-bar steam, losing 25% to waste. Losses include exhaust heat, blade turbulence, and moisture—wet steam cuts output by 5-10% unless superheated to 540°C or dried with separators. Multi-stage designs—5-20 stages—lift efficiency by 10-15% over single-stage, though costs rise. Fuel impacts performance—dry biomass (4.5 kWh/kg) beats wet (3 kWh/kg), with moisture above 20% slashing steam quality.
Historically, industrial steam turbines emerged from late 19th-century breakthroughs. Parsons’ 1884 reaction turbine and De Laval’s impulse design set the stage, but by the 1910s, factories swapped steam engines for turbines—10-20% efficiency vs. 5-10%. Early 20th-century coal-fired turbines powered mills, scaling to megawatts by the 1930s. WWII saw turbines run factories on oil or coal when grids failed. The 1970s energy crisis drove CHP adoption—biomass and waste-heat turbines cut oil reliance. Today, they align with green goals—wood-fired turbines in sawmills or waste-heat units in glassworks reduce carbon footprints.
Their modern significance is multifaceted. On-site power slashes costs—a 5 MW turbine saves $500,000-$1 million yearly vs. grid power. CHP doubles value—a 10 MW unit recovers $2-3 million in heat annually. Sustainability shines—biomass turbines burn 1-2 tons/hour of waste for 5 MW, while waste-heat units use kiln exhaust, needing no fuel. Reliability is unmatched—turbines run 20-30 years, 8,000 hours/year, in dust, heat, or humidity. A 15 MW turbine in a fertilizer plant might use ammonia process heat, producing power and steam emissions-free.
Challenges remain. Costs hit $2-5 million for a 5 MW system—boiler, turbine, piping—though CHP payback is 3-5 years. Maintenance—blade checks, seal swaps, boiler cleaning—runs $50,000-$150,000 yearly, with 1-2 days downtime. Emissions need controls—biomass emits 50-200 mg/m³ particulates, requiring $100,000 filters for compliance (e.g., EU limits at 20 mg/m³). Space—10×20 meters for 5 MW—strains tight plants, and startups (30-60 minutes) lag gas turbines (5-10 minutes). Fuel logistics—wet biomass or uneven waste heat—cut output if inconsistent.
Examples show their range. A 1 MW back-pressure turbine in a dairy burns gas, producing 800 kW and 3 tons/hour of 5-bar steam for pasteurization, costing $500,000 but saving $150,000 yearly. A 20 MW extraction turbine in a steel mill uses furnace gas, delivering 15 MW and 30 tons/hour of 15-bar steam, costing $10 million but saving $3 million annually. A 200 kW condensing turbine in a remote mine, diesel-fueled, powers drills at 35% efficiency, costing $100,000 but halving fuel use.
Industrial steam turbines are industrial energy’s backbone, merging power and heat with precision. Their evolution—from coal-fired mills to biomass-driven plants—tracks industry’s efficiency and sustainability push. With smart tech, durable materials, and green fuels, they’ll keep driving production, proving their enduring value.
Steam Turbine Generator
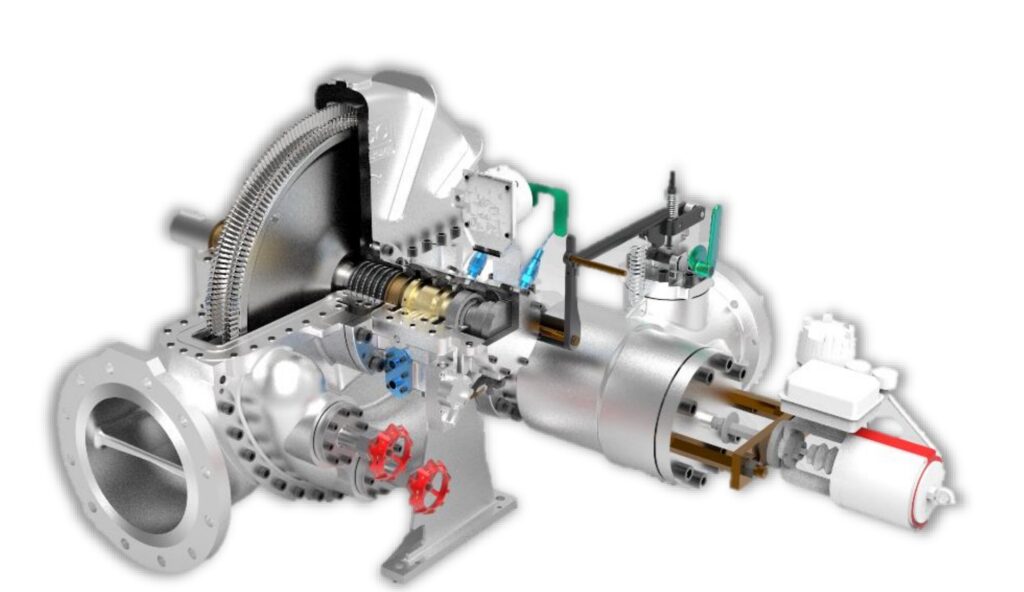
It seems like you’re asking for a detailed exploration of steam turbine generators, which are systems where a steam turbine is paired with an electric generator to produce electricity. Given our prior discussions, I’ll assume you want a general, informative overview of steam turbine generators, building on the foundation of steam turbines but focusing on their role in power generation, particularly in industrial and utility contexts. I’ll cover their mechanics, types, applications, design specifics, historical development, and modern significance, providing a comprehensive picture without tying it strictly to previous topics unless relevant. Here’s an in-depth look at steam turbine generators.
Steam turbine generators are integrated systems that convert thermal energy from steam into electrical energy, combining a steam turbine’s mechanical power with a generator’s ability to produce electricity. They are a cornerstone of modern power generation, used in industrial plants, utility-scale power stations, and even smaller off-grid setups, harnessing heat from fuels like coal, natural gas, nuclear reactions, biomass, or solar thermal sources. The steam turbine spins a rotor, which drives the generator to produce electricity, typically synchronized with electrical grids at 50 Hz (3,000 RPM) or 60 Hz (3,600 RPM). These systems range from small units generating a few kilowatts to massive installations producing over 1,000 megawatts, making them vital to both industrial self-sufficiency and global energy supply.
The mechanics of a steam turbine generator begin with the Rankine cycle. A boiler heats water using a heat source—say, burning coal at 1,200°C or nuclear fission at 300°C—producing high-pressure steam at 10-300 bar and 200-600°C, depending on the system. This steam enters the turbine, flowing over blades on a rotor. As it expands—dropping from, say, 100 bar to 0.05 bar—it accelerates, pushing the blades and spinning the rotor at speeds like 3,000-10,000 RPM. The rotor connects to a generator via a shaft, often with a gearbox in smaller units to adjust speed. Inside the generator, the rotor’s motion—surrounded by a stator with copper windings—induces an electromagnetic field, generating alternating current (AC) electricity. Steam exits either into a condenser (cooling it back to water for reuse) or at a usable pressure for industrial processes in CHP setups, with the condenser path maximizing electrical output.
Types of steam turbine generators reflect their turbine designs and applications. Condensing steam turbine generators exhaust steam into a vacuum (0.05-0.1 bar) via a condenser, optimizing power output at 35-45% efficiency. A 500 MW coal plant might use a multi-stage condensing turbine with high-pressure (HP), intermediate-pressure (IP), and low-pressure (LP) sections, each with blades tailored to steam conditions—small HP blades for 300 bar, huge LP blades for 0.05 bar. Back-pressure steam turbine generators exhaust at 5-20 bar for industrial heat, common in factories. A 5 MW unit in a paper mill might produce 4 MW and 10 tons/hour of 10-bar steam, hitting 80% total efficiency in CHP mode. Extraction steam turbine generators tap steam at intermediate pressures—e.g., 15 bar and 3 bar—while generating power, ideal for refineries needing both. A 10 MW extraction unit might deliver 8 MW and 20 tons/hour of steam.
Combination steam turbine generators blend impulse and reaction designs. High-pressure stages use impulse blades—steam jets from nozzles onto buckets—while lower stages use reaction blades, where steam expands across moving blades, common in industrial or mid-sized utility systems like a 50 MW biomass plant. Reheat steam turbine generators reheat steam mid-process—e.g., from 20 bar back to 540°C—boosting efficiency by 4-5%, used in large fossil-fuel plants like a 1,000 MW coal unit. Single-stage units, simple and compact, suit small setups—say, a 100 kW generator—while multi-stage designs with 5-50 stages dominate larger systems for efficiency, like a 200 MW nuclear generator.
Applications span scales and sectors. Utility power plants use massive steam turbine generators—coal (37% of global power), gas (25%), nuclear (10%)—producing 100-1,750 MW. A 1,200 MW nuclear plant might power 1.5 million homes, with three LP turbines exhausting to condensers. Industrial facilities deploy smaller units—50 kW to 50 MW—for on-site power and heat. A 5 MW CHP generator in a chemical plant burns gas, supplying 4 MW and steam for reactors. Biomass plants burn wood or waste—e.g., a 10 MW unit uses 2 tons/hour of wood chips—supporting rural grids or factories. Geothermal and solar thermal plants use steam turbine generators—5-100 MW—converting earth or sun heat into power. Off-grid systems, like a 50 kW unit in a remote mine, provide backup or standalone electricity.
Design specifics tie the turbine and generator into a cohesive unit. Turbine blades vary—HP blades (5-20 cm) use nickel alloys like Inconel 718 for 600°C and 300 bar, resisting creep, while LP blades (up to 2 meters) use titanium to handle wet steam at 500 m/s tip speeds. Rotors, forged steel, spin at 3,000-10,000 RPM, balanced to avoid vibration, with couplings or gearboxes (e.g., 5:1 ratio) linking to generators. Generators use synchronous designs—rotors with magnetic poles spin within stator windings—producing AC at 50/60 Hz, with outputs from 400 V (small units) to 13.8 kV (large plants). Casings, steel or alloys, contain 300 bar, with seals like labyrinths cutting leakage. Boilers, producing 1-1,000 tons/hour of steam, integrate with fuel systems—coal, gas, biomass—or heat recovery units. Cooling systems—water towers or air fans—support condensing designs, rejecting 50-60% of heat as waste.
Efficiency hinges on turbine design and steam conditions. Condensing units hit 35-45%—a 1,000 MW coal generator converts 40% of fuel energy to electricity, losing 60% to exhaust and friction. CHP units reach 70-85% total efficiency—e.g., a 5 MW back-pressure generator turns 25% into power, 50% into steam, losing 25%. Losses include exhaust heat, blade turbulence, and moisture—wet steam cuts output by 5-10% unless superheated to 540°C or dried. Multi-stage turbines (10-50 stages) extract energy gradually, boosting efficiency by 10-15% over single-stage. Supercritical steam (600°C, 300 bar) in large units pushes electrical efficiency to 47%, nearing the Rankine ideal of 60%.
Historically, steam turbine generators trace to the late 19th century. Sir Charles Parsons’ 1884 turbine, paired with a dynamo, generated 7.5 kW, proving the concept. Gustaf de Laval’s impulse design followed, powering early generators. By 1900, turbines outpaced steam engines, scaling to megawatts with better steels. The 20th century saw growth—coal-fired units electrified cities, nuclear turbines emerged in the 1950s, and reheat cycles (1930s) lifted efficiency. WWII used them in ships and factories, while the 1970s energy crisis spurred industrial CHP adoption.
Today, steam turbine generators remain essential. Utility plants—coal, gas, nuclear—rely on them for baseload power, though coal declines with emissions rules. Industrial CHP units save costs—a 10 MW generator cuts a factory’s $2 million yearly energy bill by half. Renewables use them—biomass (1-50 MW), geothermal (5-100 MW), and solar thermal (10-200 MW)—supporting green grids. Innovations enhance them—ceramic blades handle 1,000°C, 3D-printed parts improve precision, and AI optimizes performance. The Arabelle generator, at 1,750 MW, powers 2 million homes with 2-meter LP blades, while a 50 kW biomass unit lights a village.
Advantages include high output (up to 1,750 MW), longevity (30-50 years), and fuel flexibility. Challenges are cost ($2-10 million for 5-50 MW), complexity (boilers, condensers), and slow startups (hours vs. minutes for gas turbines). Steam turbine generators bridge past and future, driving industry and grids with proven tech, evolving for sustainability.
Below is a detailed exploration of steam turbine generators, broken into distinct sections for clarity. Each section expands on the mechanics, types, applications, design specifics, historical context, and modern significance of these systems, which pair steam turbines with electric generators to convert thermal energy into electricity. This format provides a comprehensive, structured overview of steam turbine generators, used in industrial plants, utility power stations, and renewable energy systems.
What Are Steam Turbine Generators?
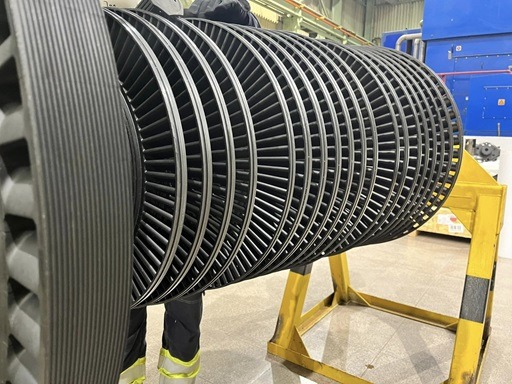
Steam turbine generators are integrated systems that transform the thermal energy of steam into electrical energy by combining a steam turbine’s mechanical power with a generator’s electrical output. They are foundational to power generation, converting heat from diverse sources—coal, natural gas, nuclear reactions, biomass, or solar thermal—into electricity for industrial, utility, or off-grid use. The steam turbine spins a rotor, driving a generator that produces alternating current (AC), typically synchronized with electrical grids at 50 Hz (3,000 RPM) or 60 Hz (3,600 RPM). These systems scale from small units generating a few kilowatts to massive installations exceeding 1,000 megawatts, making them versatile for factories, power plants, and sustainable energy projects.
How They Work
The operation of a steam turbine generator follows the Rankine cycle, a thermodynamic process optimized for power production. A boiler heats water using a fuel source—coal burning at 1,200°C, nuclear fission at 300°C, or biomass at 800-1,000°C—producing steam at pressures of 10-300 bar and temperatures of 200-600°C. This steam enters the turbine, flowing over blades mounted on a rotor. As it expands—say, from 100 bar to 0.05 bar—it accelerates to speeds like 500 m/s, pushing the blades and spinning the rotor at 3,000-10,000 RPM, depending on design. The rotor connects to a generator via a shaft, often with a gearbox in smaller units to adjust speed. Inside the generator, the rotor—fitted with magnetic poles—spins within a stator’s copper windings, inducing an electromagnetic field that generates AC electricity at voltages from 400 V to 13.8 kV. Steam exits into a condenser, cooling to water for reuse in condensing systems, or at usable pressures (e.g., 5-20 bar) for industrial heat in CHP setups, with condensing maximizing electrical output.
Types of Steam Turbine Generators
Steam turbine generators vary by turbine design and purpose, tailored to specific energy needs:
- Condensing Steam Turbine Generators: Exhaust steam into a vacuum (0.05-0.1 bar) via a condenser, maximizing power at 35-45% efficiency. A 500 MW coal unit might use HP (300 bar), IP (50 bar), and LP (0.05 bar) stages, with LP blades up to 2 meters long handling high-volume steam.
- Back-Pressure Steam Turbine Generators: Exhaust at 5-20 bar for industrial heat, common in CHP. A 5 MW unit in a paper mill produces 4 MW and 10 tons/hour of 10-bar steam, achieving 80% total efficiency.
- Extraction Steam Turbine Generators: Tap steam at intermediate pressures—e.g., 15 bar and 3 bar—while generating power. A 10 MW refinery unit delivers 8 MW and 20 tons/hour of steam, adjustable via valves.
- Combination Steam Turbine Generators: Blend impulse (high-pressure jets) and reaction (expansion across blades) designs. A 50 MW biomass unit optimizes steam across 10-20 stages.
- Reheat Steam Turbine Generators: Reheat steam mid-process—e.g., from 20 bar back to 540°C—adding 4-5% efficiency. A 1,000 MW coal unit uses this for fuel savings.
- Single-Stage vs. Multi-Stage: Single-stage suits small units (100 kW), multi-stage (5-50 stages) boosts efficiency in larger systems (200 MW).
Applications
Steam turbine generators serve diverse sectors:
- Utility Power Plants: Generate 100-1,750 MW for grids—coal (37% of global power), gas (25%), nuclear (10%). A 1,200 MW nuclear unit powers 1.5 million homes.
- Industrial Facilities: Produce 50 kW-50 MW for on-site power and heat. A 5 MW CHP unit in a chemical plant supplies 4 MW and steam for reactors.
- Biomass Plants: Burn wood or waste—e.g., a 10 MW unit uses 2 tons/hour of wood chips—for rural grids or factories.
- Geothermal and Solar Thermal: Convert earth or sun heat into 5-100 MW. A 50 MW solar thermal unit uses mirrors to heat steam.
- Off-Grid Systems: Provide backup or standalone power—e.g., a 50 kW unit in a remote mine runs on diesel or biomass.
Design Specifics
Design integrates turbine and generator components:
- Turbine Blades: HP blades (5-20 cm) use nickel alloys (Inconel 718) for 600°C, 300 bar, with coatings resisting creep. LP blades (up to 2 meters) use titanium for wet steam at 500 m/s tip speeds.
- Rotor: Forged steel, 1-20 tons, spins at 3,000-10,000 RPM, balanced to avoid vibration, with couplings or gearboxes (e.g., 5:1 ratio) linking to generators.
- Generator: Synchronous design—rotor poles spin in stator windings—produces AC at 50/60 Hz, 400 V to 13.8 kV, with cooling (air or hydrogen) for heat dissipation.
- Casings: Steel or alloys contain 300 bar, with labyrinth seals cutting leakage by 5-10%.
- Boilers: Produce 1-1,000 tons/hour of steam, fueled by coal, gas, biomass, or waste heat recovery.
- Cooling Systems: Water towers or air fans reject 50-60% of heat in condensing units, using 1-2% of output as waste.
Efficiency and Performance
Efficiency depends on design and steam conditions:
- Condensing Units: 35-45% electrical efficiency—e.g., a 1,000 MW unit converts 40% of fuel energy, losing 60% to exhaust and friction.
- CHP Units: 70-85% total efficiency—e.g., a 5 MW unit turns 25% into power, 50% into steam, losing 25%.
- Losses: Exhaust heat, blade turbulence, moisture (5-10% output drop unless superheated to 540°C or dried).
- Enhancements: Multi-stage (10-50 stages) adds 10-15% efficiency; supercritical steam (600°C, 300 bar) hits 47%, nearing Rankine’s 60% ideal.
Historical Development
Steam turbine generators trace to the late 19th century:
- Origins: Parsons’ 1884 turbine (7.5 kW) and De Laval’s impulse design pioneered the concept, paired with early dynamos.
- Early 20th Century: Outpaced steam engines by 1900, scaling to megawatts with steel advances.
- Mid-20th Century: Coal and nuclear units grew, with reheat cycles (1930s) and supercritical steam (1950s) lifting efficiency.
- WWII and Beyond: Powered ships and factories; 1970s energy crisis spurred industrial CHP.
Modern Significance
Steam turbine generators remain vital:
- Utility Role: Coal, gas, nuclear plants rely on them, though coal fades with emissions goals.
- Industrial Impact: CHP units save $1-3 million yearly—e.g., a 10 MW unit halves a factory’s energy costs.
- Renewables: Biomass (1-50 MW), geothermal (5-100 MW), solar thermal (10-200 MW) support green grids.
- Innovations: Ceramic blades (1,000°C), 3D-printed parts, AI controls enhance performance.
Advantages and Challenges
- Advantages: High output (1,750 MW max), 30-50 year lifespan, fuel flexibility.
- Challenges: High cost ($2-10 million for 5-50 MW), complex infrastructure (boilers, condensers), slow startups (hours vs. minutes).
Examples
- Utility: The Arabelle (1,750 MW) powers 2 million homes with 2-meter LP blades.
- Industrial: A 5 MW CHP unit in a mill burns wood, producing 4 MW and 10 tons/hour of steam.
- Off-Grid: A 50 kW biomass unit lights a village, using 20 kg/hour of wood.
Steam turbine generators bridge heat and electricity, evolving from Parsons’ 7.5 kW to today’s gigawatt giants, driving industry and grids with adaptability and innovation.
Here’s a continued exploration of steam turbine generators, organized into sections for clarity and depth. Each section builds on the previous discussion, diving deeper into their mechanics, types, applications, design specifics, historical context, modern significance, and operational details. Steam turbine generators pair a steam turbine’s mechanical power with a generator’s electrical output to convert thermal energy from steam into electricity, serving industrial plants, utility power stations, and renewable energy systems with remarkable versatility.
What Are Steam Turbine Generators?
Steam turbine generators are sophisticated systems that transform thermal energy from steam into electrical energy, integrating a steam turbine’s rotational force with a generator’s ability to produce alternating current (AC). They harness heat from sources like coal, natural gas, nuclear fission, biomass (e.g., wood or agricultural waste), or solar thermal energy, making them essential for power generation across scales—from small industrial units producing a few kilowatts to utility-scale systems exceeding 1,000 megawatts. The turbine spins a rotor, driving a generator typically synchronized with electrical grids at 50 Hz (3,000 RPM) or 60 Hz (3,600 RPM), delivering electricity for factories, cities, or remote sites. Their adaptability and efficiency have made them a backbone of modern energy infrastructure.
How They Work
Steam turbine generators operate on the Rankine cycle, a thermodynamic process tailored for efficient power production. A boiler heats water using a heat source—coal combusting at 1,200°C, nuclear reactions at 300°C, or biomass at 800-1,000°C—generating steam at pressures from 10 to 300 bar and temperatures from 200 to 600°C. This steam flows into the turbine, passing over blades mounted on a rotor. As it expands—dropping from, say, 100 bar to 0.05 bar—it accelerates to speeds like 500 meters per second, pushing the blades and spinning the rotor at 3,000-10,000 RPM, depending on design and load. The rotor connects to a generator via a shaft, often with a gearbox in smaller systems to adjust speed—e.g., reducing 10,000 RPM to 1,500 RPM for a pump. Inside the generator, the rotor’s magnetic poles spin within stator windings, inducing an electromagnetic field that produces AC electricity at voltages from 400 V (small units) to 13.8 kV (large plants). Steam exits into a condenser, cooling to water at 30-50°C for reuse in condensing systems, or at usable pressures like 5-20 bar for industrial heat in combined heat and power (CHP) setups, with condensing maximizing electrical yield.
Types of Steam Turbine Generators
Steam turbine generators vary by turbine design and application, each suited to specific needs:
- Condensing Steam Turbine Generators: Exhaust steam into a vacuum (0.05-0.1 bar) via a condenser, optimizing power output at 35-45% efficiency. A 500 MW coal unit uses multi-stage turbines—high-pressure (HP) at 300 bar, intermediate-pressure (IP) at 50 bar, low-pressure (LP) at 0.05 bar—with LP blades up to 2 meters long handling expanded steam.
- Back-Pressure Steam Turbine Generators: Exhaust at 5-20 bar for industrial heat, ideal for CHP. A 5 MW unit in a sugar mill produces 4 MW and 10 tons/hour of 10-bar steam, achieving 80% total efficiency.
- Extraction Steam Turbine Generators: Tap steam at intermediate pressures—e.g., 15 bar for heating, 3 bar for preheating—while generating power. A 10 MW refinery unit delivers 8 MW and 20 tons/hour of steam, adjustable with control valves.
- Combination Steam Turbine Generators: Blend impulse (high-pressure jets onto buckets) and reaction (expansion across blades) designs. A 50 MW biomass unit optimizes steam across 10-20 stages.
- Reheat Steam Turbine Generators: Reheat steam mid-process—e.g., from 20 bar back to 540°C—adding 4-5% efficiency. A 1,000 MW coal unit uses this for fuel economy.
- Single-Stage vs. Multi-Stage: Single-stage suits small units (100 kW, simple), multi-stage (5-50 stages) enhances efficiency in larger systems (200 MW).
Applications
Steam turbine generators serve a wide range of purposes:
- Utility Power Plants: Generate 100-1,750 MW for national grids—coal (37% of global power), gas (25% in combined-cycle), nuclear (10%). A 1,200 MW nuclear unit powers 1.5 million homes with three LP turbines.
- Industrial Facilities: Produce 50 kW-50 MW for on-site power and heat. A 5 MW CHP unit in a chemical plant supplies 4 MW and steam for distillation.
- Biomass Plants: Burn wood or waste—e.g., a 10 MW unit uses 2 tons/hour of wood chips—for rural grids or factory self-sufficiency.
- Geothermal and Solar Thermal: Convert natural or solar heat into 5-100 MW. A 50 MW geothermal unit taps underground steam, while a solar thermal unit uses mirrors.
- Off-Grid Systems: Provide standalone power—e.g., a 50 kW unit in a remote mine runs on diesel or local biomass, supporting critical loads.
Design Specifics
The design integrates turbine and generator components for seamless operation:
- Turbine Blades: HP blades (5-20 cm) use nickel alloys like Inconel 718, handling 600°C and 300 bar, with coatings (e.g., chromium carbide) resisting creep and corrosion. LP blades (up to 2 meters) use titanium, enduring wet steam at 500 m/s tip speeds.
- Rotor: Forged steel, 1-20 tons, spins at 3,000-10,000 RPM, precision-balanced to avoid vibration, with couplings or gearboxes (e.g., 5:1 ratio) linking to generators.
- Generator: Synchronous design—rotor poles spin in stator windings—produces AC at 50/60 Hz, 400 V to 13.8 kV. Cooling (air for small units, hydrogen for large) dissipates heat from 50-60% losses.
- Casings: Steel or alloys contain 300 bar, with labyrinth seals reducing leakage by 5-10%, critical for efficiency.
- Boilers: Produce 1-1,000 tons/hour of steam, fueled by coal, gas, biomass, or waste heat recovery from industrial exhausts.
- Cooling Systems: Water towers or air fans reject 50-60% of heat in condensing units, evaporating 1-2% of output as waste, with water needs of 1-2 liters/kWh.
Efficiency and Performance
Efficiency varies by design and steam conditions:
- Condensing Units: 35-45% electrical efficiency—e.g., a 1,000 MW unit converts 40% of fuel energy, losing 60% to exhaust, friction, and turbulence.
- CHP Units: 70-85% total efficiency—e.g., a 5 MW unit turns 25% into power, 50% into steam, losing 25% to waste heat.
- Losses: Exhaust heat (50-60%), blade-gap leaks (2-5%), moisture (5-10% output drop unless superheated to 540°C or dried with separators).
- Enhancements: Multi-stage (10-50 stages) adds 10-15% efficiency; supercritical steam (600°C, 300 bar) hits 47%; reheat cycles boost 4-5%.
Historical Development
Steam turbine generators evolved over a century:
- Origins: Parsons’ 1884 turbine (7.5 kW) and De Laval’s impulse design paired with dynamos, proving the concept.
- Early 20th Century: By 1900, turbines scaled to megawatts, outpacing steam engines with better steels and boilers.
- Mid-20th Century: Coal and nuclear units grew—reheat cycles (1930s), supercritical steam (1950s)—lifting efficiency from 20% to 45%.
- WWII and Beyond: Powered ships and factories; 1970s energy crisis drove industrial CHP adoption with biomass and waste heat.
Modern Significance
Steam turbine generators remain critical:
- Utility Role: Coal, gas, nuclear plants rely on them—e.g., a 1,000 MW gas unit supports baseload, though coal declines with emissions rules.
- Industrial Impact: CHP units save costs—a 10 MW unit cuts a factory’s $2-3 million yearly energy bill by half.
- Renewables: Biomass (1-50 MW), geothermal (5-100 MW), solar thermal (10-200 MW) align with green goals—e.g., a 20 MW wood-fired unit powers a rural grid.
- Innovations: Ceramic blades (1,000°C tolerance), 3D-printed parts, AI-driven controls enhance efficiency and durability.
Advantages and Challenges
- Advantages: High output (1,750 MW max), 30-50 year lifespan, fuel flexibility (fossil, nuclear, renewable).
- Challenges: High cost ($2-10 million for 5-50 MW), complex setups (boilers, condensers), slow startups (hours vs. minutes for gas turbines), water-heavy cooling (1-2 liters/kWh).
Examples
- Utility: The Arabelle (1,750 MW) powers 2 million homes with 2-meter LP blades in nuclear plants.
- Industrial: A 5 MW CHP unit in a mill burns wood, producing 4 MW and 10 tons/hour of steam, saving $1 million yearly.
- Off-Grid: A 50 kW biomass unit in a village uses 20 kg/hour of wood, powering lights and pumps.
Steam turbine generators are a testament to engineering ingenuity, evolving from kilowatt-scale pioneers to gigawatt powerhouses, driving industry, grids, and sustainability with unmatched versatility.
Micro Steam Turbines
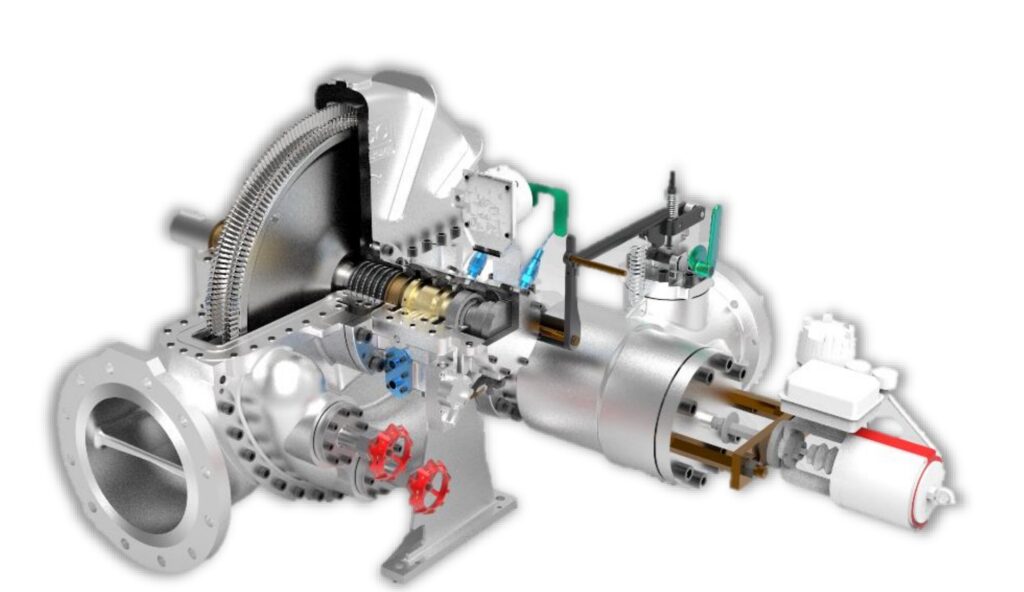
Micro steam turbines are compact, small-scale versions of traditional steam turbines, designed to generate electricity or mechanical power in applications where large turbines are impractical. Typically ranging from a few watts to about 1 megawatt (MW) in capacity, with most falling between 1 kilowatt (kW) and 500 kW, these turbines cater to niche markets such as off-grid power, small industrial operations, rural electrification, and renewable energy systems like biomass or solar thermal setups. They operate on the same fundamental principles as their larger counterparts—converting thermal energy from steam into mechanical work—but are engineered for simplicity, portability, and efficiency at lower outputs. Micro steam turbines are particularly appealing in settings where fuel sources like wood, agricultural waste, or waste heat are abundant, offering a sustainable, decentralized energy solution. Below is a detailed exploration of micro steam turbines, covering their mechanics, design, applications, advantages, challenges, and relevance in today’s energy landscape.
The operation of a micro steam turbine mirrors the Rankine cycle used in larger systems, scaled down for smaller heat inputs and power needs. A heat source—often a small boiler or heat exchanger—burns fuel like biomass (wood chips, pellets, or straw), biogas, or fossil fuels (diesel, natural gas), or captures waste heat from engines or industrial processes. This heats water to produce steam, typically at pressures of 5 to 20 bar and temperatures of 200 to 300°C, though some advanced designs reach 50 bar and 400°C for higher efficiency. The steam enters the turbine, flowing over blades attached to a rotor. As it expands—dropping from, say, 10 bar to 1 bar—it accelerates, pushing the blades and spinning the rotor at speeds ranging from 3,000 to 30,000 revolutions per minute (RPM), depending on size and design. The rotor connects to a generator via a shaft, often with a gearbox to adjust speed—for example, reducing 10,000 RPM to 3,000 RPM for a 50 Hz grid—or directly drives small machinery like pumps or fans. After expansion, the steam either exhausts to the atmosphere (in open systems), condenses into water for reuse (in closed systems with a condenser), or exits at a usable pressure (e.g., 2-5 bar) for heating in combined heat and power (CHP) setups.
The design of micro steam turbines emphasizes compactness and simplicity. Most are single-stage or dual-stage, unlike the multi-stage designs of large turbines, with a single set of blades or two sets (one fixed, one moving) to extract energy from steam. Impulse designs, inspired by Gustaf de Laval’s 19th-century turbine, are common—steam accelerates through nozzles into high-speed jets (up to 500 m/s) that strike curved, bucket-shaped blades, spinning the rotor at high speeds like 20,000-30,000 RPM. Reaction designs, based on Charles Parsons’ concept, are less frequent but used in slightly larger micro turbines (e.g., 100-500 kW), where steam expands across moving blades for gradual energy extraction, operating at lower speeds like 3,000-10,000 RPM. Blades are small—typically 2-10 centimeters long—made of stainless steel or lightweight alloys like aluminum for cost and durability, though high-end units use titanium or nickel alloys for better heat resistance. Rotors, often forged steel or aluminum, weigh a few kilograms, balanced to minimize vibration at high speeds. Casings, cast iron or steel, contain pressures up to 50 bar, with basic seals (e.g., packing glands) to reduce steam leakage, though efficiency suffers compared to the labyrinth seals of big turbines.
Micro steam turbine generators pair the turbine with a small alternator or dynamo, producing AC or DC electricity. A 10 kW unit might generate 230 V AC for household use or 12-48 V DC for battery charging, with outputs scaled to match load—e.g., 1 kW for lights, 50 kW for a workshop. Boilers are compact, producing 10-500 kg/hour of steam, fueled by wood (4.5 kWh/kg dry), biogas (5-6 MJ/m³), or waste heat (free energy). Cooling in condensing systems uses air fans or small water loops—e.g., a 5 kW unit might need 10 liters/hour of water—while CHP setups skip condensers, piping exhaust steam to radiators or dryers. Control systems are basic—manual valves or simple electronic governors—keeping costs low, though advanced units add microcontrollers for automation.
Applications of micro steam turbines are diverse and practical. In off-grid settings, a 5 kW turbine powered by wood might run a cabin’s lights, fridge, and pump, burning 2-3 kg/hour of logs, ideal for remote areas with forest access. Small industries use them for localized power—a 50 kW unit in a sawmill burns wood waste (20 kg/hour) to drive tools and dry lumber, cutting grid reliance. Biomass systems leverage them for rural electrification—e.g., a 100 kW turbine in an Indian village burns rice husks, powering 50 homes and a water pump. Waste heat recovery is a growing niche—a 20 kW turbine in a factory captures exhaust from a diesel engine (200-300°C), generating power without extra fuel. Educational labs and hobbyists use tiny units—1-10 kW—for experiments or DIY projects, often paired with solar concentrators or small stoves. Marine use exists in small boats—a 10 kW turbine might replace a diesel generator, running on biofuel.
Efficiency in micro steam turbines is lower than in large systems, typically 10-20% for electrical output, due to scale and simplicity. A 10 kW unit burning 5 kg/hour of wood (22.5 kWh thermal) might produce 2 kW (9% efficiency), losing 80% to exhaust heat, friction, and leaks. CHP boosts total efficiency to 50-70%—e.g., a 5 kW turbine generates 1 kW and 3 kW of heat via 2-bar steam, losing 30% to waste. Losses stem from small blade sizes (less energy extraction), poor sealing (5-10% steam escapes), and low steam quality—wet steam (10-20% moisture) cuts output unless superheated to 300°C. Multi-stage designs or higher pressures (20-50 bar) can hit 25-30% electrical efficiency, but complexity rises. Fuel quality matters—dry wood outperforms wet (50% moisture halves energy), and consistent heat input prevents steam fluctuations.
Historically, micro steam turbines trace to early 20th-century innovations. De Laval’s 1880s impulse turbine, spinning at 30,000 RPM, inspired small-scale designs for pumps and generators. Parsons’ multi-stage concept scaled down by the 1920s for factories, though steam engines dominated until turbines shrank further. WWII saw micro turbines in portable units—e.g., 5-10 kW systems powered field hospitals on wood gas. Post-war, interest waned with cheap oil, but the 1970s energy crisis revived them for biomass and off-grid use. Today’s designs build on this, with 3D printing and microelectronics enabling affordable, efficient units.
Advantages of micro steam turbines include their small size—e.g., a 10 kW unit fits in a 1×1-meter space—making them portable or factory-friendly. Fuel flexibility is key—they burn wood, waste, or biogas, or use waste heat, ideal where fossil fuels are scarce. Low cost appeals to niche users—a 5 kW DIY turbine might cost $1,000-$3,000 vs. $5,000 for solar. CHP capability doubles value—e.g., a 10 kW unit powers a home and heats it, slashing energy bills. Sustainability shines—biomass units are near carbon-neutral with regrowth, and waste heat recovery cuts emissions. Reliability is decent—simple designs run 5-10 years with basic upkeep, handling 1,000-5,000 hours/year.
Challenges are significant. Low efficiency (10-20%) means high fuel use—a 5 kW unit needs 2-3 kg/hour of wood vs. 0.5 liters/hour of diesel for a generator. Labor is intensive—chopping wood, feeding boilers (every 1-2 hours), and removing ash take 1-2 hours daily. Maintenance includes blade cleaning, seal replacement, and boiler descaling—$100-$500 yearly for a 10 kW unit, with downtime cutting output. Emissions—smoke, particulates (50-200 mg/m³)—need filters ($200-$500) for compliance (e.g., U.S. EPA limits at 25 mg/m³). Complexity rises with condensing or multi-stage designs, pushing costs to $5,000-$20,000 for 50-100 kW units. Scale limits competitiveness—solar or wind often beat them below 100 kW on cost ($1-2/W vs. $3-5/W).
Modern relevance ties to decentralization and renewables. Off-grid demand grows—a 10 kW turbine powers a homestead where solar fails in winter. Biomass adoption rises—e.g., a 50 kW unit in a Brazilian farm burns sugarcane waste, supporting microgrids. Waste heat recovery gains traction—a 20 kW turbine in a glass factory uses kiln exhaust, saving $10,000 yearly. Innovations help—3D-printed blades cut costs, microcontrollers boost efficiency, and ceramic coatings handle 400°C, though adoption lags behind solar’s simplicity. Examples include a 1 kW turbine on a wood stove (50 W power, 500 W heat) for cabins, or a 100 kW unit in a Thai village burning coconut shells for 80 homes.
Micro steam turbines fill a unique niche, blending old-school steam tech with modern needs. They’re not grid-scale giants but excel where small, flexible power meets abundant local fuel. As energy diversifies, their role in off-grid, biomass, and waste-heat systems keeps them relevant, a pint-sized echo of steam’s industrial legacy.
Here’s a continued exploration of micro steam turbines, presented as a long, detailed plain text that builds on the previous discussion. Micro steam turbines are small-scale power generation devices that convert thermal energy from steam into mechanical work, typically driving a generator to produce electricity or powering small machinery in applications where larger turbines are impractical. Ranging from a few watts to about 1 megawatt, with most units falling between 1 kilowatt and 500 kilowatts, they serve niche roles in off-grid setups, small industries, rural communities, and renewable energy systems like biomass or waste heat recovery. Their compact size, fuel flexibility, and ability to integrate with local resources make them a compelling option for decentralized energy, despite their challenges. Let’s dive deeper into their mechanics, design variations, applications, operational details, historical context, modern relevance, advantages, and limitations.
Micro steam turbines operate on the same Rankine cycle principle as larger turbines, scaled down for lower power outputs and simpler setups. A heat source—typically a small boiler or heat exchanger—heats water to produce steam, using fuels like biomass (wood logs, pellets, or agricultural waste such as rice husks), biogas from anaerobic digesters, fossil fuels like diesel or propane, or waste heat from engines, kilns, or industrial exhausts. Steam conditions are modest compared to utility turbines—pressures range from 5 to 20 bar, with temperatures of 200 to 300°C, though some advanced micro turbines push to 50 bar and 400°C to improve efficiency. This steam enters the turbine, flowing over blades attached to a rotor, a lightweight steel or aluminum shaft typically weighing a few kilograms. As the steam expands—dropping from, say, 10 bar to 1 bar or even atmospheric pressure—it accelerates to speeds of 300-500 meters per second, striking or flowing past the blades to spin the rotor at high speeds, often between 3,000 and 30,000 RPM, depending on the turbine’s size and design. The rotor connects to a generator—either directly or through a gearbox that adjusts speed, such as reducing 20,000 RPM to 3,000 RPM for a 50 Hz grid—to produce electricity, or it drives small mechanical loads like pumps, fans, or mills. After expansion, the steam exhausts in one of three ways: to the atmosphere in open systems (simplest but least efficient), into a condenser where it cools back to water at 30-50°C for reuse in closed loops, or at a usable pressure like 2-5 bar for heating in combined heat and power (CHP) configurations, a common feature in micro systems aiming to maximize energy use.
The design of micro steam turbines prioritizes compactness, affordability, and ease of use over the complexity of large-scale turbines. Most are single-stage, featuring one set of blades, or dual-stage, with a fixed stator directing steam onto a moving rotor, unlike the dozens of stages in utility turbines. Impulse designs dominate smaller units—steam accelerates through nozzles into high-velocity jets that hit curved, bucket-shaped blades, spinning the rotor at speeds like 20,000-30,000 RPM, a concept rooted in Gustaf de Laval’s 1880s turbine. Reaction designs, inspired by Charles Parsons’ multi-stage approach, appear in larger micro turbines (100-500 kW), where steam expands across moving blades, extracting energy gradually at lower speeds like 3,000-10,000 RPM for better generator compatibility. Blades are tiny—2 to 10 centimeters long—crafted from stainless steel or aluminum for cost and corrosion resistance, though high-performance units use titanium for lightweight strength or nickel alloys for heat tolerance up to 400°C. Rotors, often just a few kilograms, are precision-balanced to handle high speeds without vibration, a critical factor given their small scale. Casings, made of cast iron or low-grade steel, contain pressures up to 50 bar, with basic seals like packing glands or simple labyrinths to minimize steam leakage, though these are less effective than the advanced seals in big turbines, leading to 5-10% efficiency losses.
Micro steam turbine generators pair the turbine with a small alternator or dynamo to produce electricity, tailored to the application. A 5 kW unit might generate 230 V AC for household appliances or 12-48 V DC for battery charging, while a 50 kW unit could produce 400 V AC for a small factory. Generators are often synchronous, with a rotor spinning inside stator windings to produce AC at 50 or 60 Hz, cooled by air to manage heat from 50-60% energy losses. Boilers are compact, producing 10 to 500 kg/hour of steam—e.g., a 10 kW unit needs 20-30 kg/hour—fueled by wood (4.5 kWh/kg dry), biogas (5-6 MJ/m³), or waste heat (200-400°C exhaust). Condensing systems use air fans or small water loops—a 5 kW unit might need 10-20 liters/hour of cooling water—while CHP setups pipe exhaust steam to radiators, dryers, or water heaters, skipping the condenser for simplicity. Controls are basic—manual valves or mechanical governors adjust steam flow—though modern units add microcontrollers or sensors for automated startups and load matching, keeping costs between $1,000 and $20,000 depending on size and features.
Applications for micro steam turbines are practical and varied, excelling where small-scale, localized power is needed. In off-grid homes or cabins, a 5 kW turbine burns 2-3 kg/hour of wood to power lights, a fridge, and a well pump, perfect for forested areas where solar falters in winter—e.g., a Canadian homestead might use local birch, adding heat for a greenhouse. Small industries adopt them for self-sufficiency—a 50 kW turbine in a sawmill burns 20 kg/hour of sawdust, driving tools and drying lumber, saving $10,000-$20,000 yearly on grid power. Biomass-driven rural electrification uses them widely—a 100 kW turbine in an Indonesian village burns coconut shells, powering 50-80 homes and a rice mill, supporting microgrids where grid extension costs millions. Waste heat recovery is a key niche—a 20 kW turbine in a bakery captures oven exhaust (300°C), generating power without extra fuel, cutting costs by $5,000-$10,000 annually. Educational settings and hobbyists favor tiny units—1-10 kW—for experiments or prototypes, often paired with solar concentrators (mirrors heating a boiler) or wood stoves. Small boats use them too—a 10 kW turbine might replace a diesel generator, running on biofuel for quiet, eco-friendly power.
Efficiency in micro steam turbines is modest, typically 10-20% for electrical output, far below the 35-45% of large turbines, due to their small size and simplified design. A 10 kW unit burning 5 kg/hour of wood (22.5 kWh thermal input) might produce 2 kW of electricity (9% efficiency), losing 80% to exhaust heat, friction, and steam leaks. CHP configurations improve this to 50-70% total efficiency—e.g., a 5 kW turbine generates 1 kW of power and 3 kW of heat via 2-bar steam, losing 30% to waste. Losses come from small blades (less surface for energy transfer), basic seals (5-10% steam escapes), and wet steam (10-20% moisture unless superheated to 300°C), which erodes blades and cuts output. Higher pressures (20-50 bar) or dual-stage designs can reach 25-30% electrical efficiency, but added cost and complexity often outweigh gains. Fuel quality is critical—dry wood (10% moisture) yields 4.5 kWh/kg, while wet wood (50% moisture) drops to 3 kWh/kg, halving steam production if not pre-dried, a common issue in humid climates.
The historical roots of micro steam turbines stretch back to the late 19th century. De Laval’s 1880s impulse turbine, spinning at 30,000 RPM, pioneered small-scale steam power for pumps and early generators, inspiring micro designs. Parsons’ 1884 multi-stage turbine scaled down by the 1920s for small factories, though reciprocating steam engines ruled until turbines shrank further. World War II boosted their use—portable 5-10 kW units powered field stations or hospitals on wood or coal when fuel was scarce. Post-war, cheap oil sidelined them, but the 1970s energy crisis revived interest, with biomass and waste heat driving micro turbine development for off-grid and industrial use. Modern designs leverage this legacy, enhanced by 3D printing for cheap blades and electronics for better control, though they remain a niche compared to solar or wind.
Advantages of micro steam turbines are compelling for their context. Their small size—a 10 kW unit fits in a 1×1-meter space—makes them portable or easy to install in tight spots, unlike solar arrays needing acres. Fuel flexibility is a strength—they burn wood, waste, or biogas, or tap waste heat, thriving where diesel is costly or unavailable—e.g., a 20 kW unit in a remote mine uses local scrub wood, saving $5,000 yearly on fuel transport. Low cost appeals to budget users—a 5 kW DIY turbine costs $1,000-$3,000 vs. $5,000-$7,000 for solar with batteries. CHP doubles their value—a 10 kW unit powers a workshop and heats it, cutting energy bills by 50-70%. Sustainability is a draw—biomass units offset CO2 with regrowth, and waste heat recovery slashes emissions, aligning with green goals. Reliability suits rugged use—simple designs run 5-10 years, 1,000-5,000 hours/year, with minimal parts to fail.
Challenges limit their reach. Low efficiency (10-20%) demands high fuel input—a 5 kW unit burns 2-3 kg/hour of wood vs. 0.5 liters/hour of diesel for a generator, tripling fuel mass. Labor is a burden—chopping wood, feeding boilers every 1-2 hours, and clearing ash take 1-2 hours daily, vs. solar’s near-zero upkeep. Maintenance—blade cleaning, seal swaps, boiler descaling—costs $100-$500 yearly for a 10 kW unit, with 1-2 days downtime cutting power. Emissions—smoke, particulates (50-200 mg/m³)—require filters ($200-$500) to meet limits like the EU’s 20 mg/m³, adding cost and complexity. Initial investment scales poorly—$5,000-$20,000 for 50-100 kW units vs. $1-2/W for solar, losing competitiveness above 10 kW. Wet fuel or inconsistent heat (e.g., variable wood moisture) disrupts steam, dropping output by 20-30% if unmanaged.
Modern relevance hinges on niche energy needs. Off-grid growth fuels demand—a 10 kW turbine powers an Alaskan cabin where solar struggles in dark winters, burning local spruce. Biomass adoption rises—a 50 kW unit in a Kenyan farm burns maize stalks, supporting a microgrid for 30 homes. Waste heat recovery expands—a 20 kW turbine in a ceramic kiln uses 300°C exhaust, saving $8,000 yearly. Innovations push progress—3D-printed blades cut costs by 20-30%, microcontrollers lift efficiency to 25%, and ceramic coatings handle 400°C, though solar’s simplicity ($1/W installed) often wins. Examples include a 1 kW turbine on a stove (50 W power, 500 W heat) for rural charging, or a 100 kW unit in a Philippine village burning palm waste for 90 homes.
Micro steam turbines are small but mighty, merging steam’s legacy with modern ingenuity. They excel where fuel is local, power needs are modest, and flexibility matters—off-grid homes, small factories, or waste-heat sites. As energy decentralizes, their role grows, a compact testament to steam’s enduring utility.
Here’s another detailed expansion on micro steam turbines, presented as a long plain text that builds on the prior discussions while offering fresh depth. Micro steam turbines are small-scale devices that convert thermal energy from steam into mechanical work, typically driving a generator for electricity or powering machinery in applications where larger turbines are impractical or oversized. With power outputs ranging from a few watts to about 1 megawatt, though most commonly between 1 kilowatt and 500 kilowatts, they cater to specialized needs like off-grid power, small industrial processes, rural electrification, and renewable energy systems using biomass, biogas, or waste heat. These compact turbines balance simplicity and functionality, making them a viable option for decentralized energy in resource-rich settings. Let’s explore their mechanics, design nuances, applications, operational intricacies, historical evolution, modern significance, advantages, and challenges in greater detail.
The mechanics of a micro steam turbine follow the Rankine cycle, scaled down for modest power demands. A heat source—often a small boiler, stove, or heat exchanger—generates steam by burning fuels such as biomass (wood logs, sawdust, crop residues like corn stalks), biogas from manure or organic waste, fossil fuels like kerosene or natural gas, or by capturing waste heat from engines, ovens, or industrial exhausts at 200-400°C. Steam is produced at pressures of 5 to 20 bar and temperatures of 200 to 300°C in basic setups, though advanced micro turbines push to 50 bar and 400°C for better performance. This steam enters the turbine, flowing over blades fixed to a rotor—a lightweight shaft made of steel or aluminum, typically weighing 1-5 kilograms. As the steam expands—say, from 15 bar to 2 bar—it accelerates to 300-500 meters per second, striking or passing through the blades to spin the rotor at speeds between 3,000 and 30,000 RPM, depending on the turbine’s size and configuration. The rotor drives a generator through a direct shaft connection or a gearbox—for instance, stepping down 15,000 RPM to 3,000 RPM for a 50 Hz grid—or powers small mechanical loads like water pumps or grain mills. Post-expansion, steam exits either to the atmosphere in open-cycle systems (simplest but wasteful), into a condenser where it cools to water at 30-50°C for reuse in closed cycles, or at a usable pressure like 2-5 bar for heating in combined heat and power (CHP) setups, a feature that enhances their utility in small-scale applications.
Design-wise, micro steam turbines prioritize portability and cost over the elaborate staging of large turbines. Most are single-stage, with one set of blades, or dual-stage, pairing a fixed stator with a moving rotor, contrasting with the 10-50 stages of utility turbines. Impulse designs are prevalent, especially in smaller units—steam accelerates through nozzles into high-speed jets that hit curved, bucket-like blades, spinning the rotor at 20,000-30,000 RPM, echoing Gustaf de Laval’s high-speed turbine from the 1880s. Reaction designs appear in larger micro turbines (100-500 kW), where steam expands across moving blades, extracting energy more gradually at speeds like 3,000-10,000 RPM, inspired by Charles Parsons’ multi-stage approach. Blades are compact—2 to 10 centimeters long—made of stainless steel or aluminum for affordability and resistance to corrosion, though premium units use titanium for strength or nickel alloys for heat tolerance up to 400°C, especially in high-pressure models. Rotors, weighing a few kilograms, are carefully balanced to handle high speeds, minimizing vibration that could wear bearings or misalign shafts. Casings, typically cast iron or mild steel, withstand pressures up to 50 bar, using simple seals like packing glands or basic labyrinths to curb steam leakage, though these allow 5-10% loss, a trade-off for lower cost compared to the precision seals of industrial turbines.
Micro steam turbine generators couple the turbine with a small alternator or dynamo to produce electricity, sized for the task. A 5 kW unit might deliver 230 V AC for home use or 12-48 V DC for battery banks, while a 50 kW unit generates 400 V AC for a workshop. Generators are usually synchronous—rotor poles spin within stator windings to produce AC at 50 or 60 Hz—cooled by air to manage heat from 50-60% energy losses, with outputs adjustable via voltage regulators. Boilers are small, producing 10 to 500 kg/hour of steam—a 10 kW unit needs 20-30 kg/hour—fueled by wood (4.5 kWh/kg dry), biogas (5-6 MJ/m³), or waste heat (free if consistent). Condensing systems rely on air fans or minimal water loops—a 5 kW unit might use 10-20 liters/hour of water—while CHP setups pipe exhaust steam to heat sinks like radiators or dryers, avoiding condensers for simplicity. Controls range from manual valves and mechanical governors in basic models to microcontrollers or sensors in modern units, enabling automated operation and load tracking, with costs spanning $1,000 for a 1 kW DIY kit to $20,000 for a 100 kW commercial system.
Applications highlight their versatility in small-scale settings. Off-grid homes use them for reliable power—a 5 kW turbine burns 2-3 kg/hour of wood to run lights, a fridge, and a pump, thriving in forested regions like the Pacific Northwest where solar struggles in cloudy months. Small industries benefit from localized energy—a 50 kW turbine in a rice mill burns 20 kg/hour of husks, powering grinders and drying grain, saving $15,000-$25,000 yearly on grid costs. Biomass-driven rural electrification is a growing use—a 100 kW turbine in a Peruvian village burns sugarcane bagasse, supplying 60-100 homes and a school, supporting microgrids where grid lines cost $50,000 per kilometer. Waste heat recovery shines in efficiency—a 20 kW turbine in a brewery uses 300°C oven exhaust, generating power without fuel, cutting bills by $7,000-$12,000 annually. Hobbyists and educators use tiny units—1-10 kW—for projects or labs, pairing them with solar dishes or wood stoves, while small boats adopt 5-10 kW turbines on biofuel, replacing noisy diesel generators with quieter steam power.
Efficiency in micro steam turbines lags behind larger systems, typically 10-20% for electrical output, reflecting their small scale and simplified construction. A 10 kW unit burning 5 kg/hour of wood (22.5 kWh thermal) might produce 2 kW (9% efficiency), losing 80% to exhaust heat, friction, and leaks, compared to 35-45% in utility turbines. CHP boosts total efficiency to 50-70%—a 5 kW turbine generates 1 kW of power and 3 kW of heat via 2-bar steam, losing 30% to waste. Losses arise from small blades (less energy capture), basic seals (5-10% steam escapes), and wet steam (10-20% moisture unless superheated to 300°C), which erodes blades and reduces output. Higher pressures (20-50 bar) or dual-stage designs can reach 25-30% electrical efficiency, but costs jump from $2,000 to $10,000 for a 10 kW unit. Fuel quality impacts performance—dry wood (10% moisture) delivers 4.5 kWh/kg, while wet wood (50% moisture) falls to 3 kWh/kg, cutting steam by 30-50% if not dried, a frequent hurdle in damp climates.
Historically, micro steam turbines emerged from late 19th-century innovations. De Laval’s 1880s impulse turbine, with its 30,000 RPM speed, set the stage for small-scale steam power, initially driving pumps and early dynamos. Parsons’ 1884 multi-stage design scaled down by the 1920s for small factories, though steam engines held sway until turbines miniaturized further. World War II saw them in portable 5-10 kW units—e.g., powering remote bases on coal or wood when fuel was tight. Post-war, cheap oil dimmed their appeal, but the 1970s oil crisis sparked a revival, with biomass and waste heat driving micro turbine use in off-grid and industrial niches. Today’s designs refine this heritage—3D printing slashes blade costs by 20-30%, and electronics improve control, though they remain a specialty tool.
Advantages make them attractive in context. Their small footprint—a 10 kW unit fits in a 1×1-meter space—suits tight installations or portability, unlike solar needing large panels. Fuel flexibility excels—they burn wood, waste, or biogas, or use waste heat, perfect where diesel costs $1-$2/liter or grids fail—e.g., a 20 kW unit in an African village uses crop waste, avoiding $6,000 yearly fuel imports. Low cost draws users—a 5 kW turbine at $1,000-$3,000 undercuts $5,000-$8,000 solar-plus-battery setups. CHP enhances value—a 10 kW unit powers a farm and heats a barn, saving 60-80% on energy costs. Sustainability aligns with green trends—biomass units offset CO2 with regrowth, and waste heat recovery cuts fuel use, appealing to eco-conscious users. Reliability fits tough conditions—simple designs run 5-10 years, 1,000-5,000 hours/year, with few moving parts to break.
Challenges temper their appeal. Low efficiency (10-20%) means high fuel demand—a 5 kW unit burns 2-3 kg/hour of wood vs. 0.5 liters/hour of diesel, a 5-6x mass penalty. Labor is demanding—chopping fuel, feeding boilers every 1-2 hours, and clearing ash take 1-2 hours daily, vs. wind’s near-zero effort. Maintenance—blade cleaning, seal swaps, boiler descaling—costs $100-$500 yearly for a 10 kW unit, with downtime disrupting power. Emissions—smoke, particulates (50-200 mg/m³)—need filters ($200-$500) to meet limits like the EU’s 20 mg/m³, raising complexity. Costs scale poorly—$5,000-$20,000 for 50-100 kW units vs. $1-2/W for solar, losing ground above 10 kW. Fuel inconsistency—wet wood or uneven heat—drops output by 20-40% if unmanaged.
Modern significance reflects niche energy trends. Off-grid needs grow—a 10 kW turbine powers a Montana ranch where solar fades in snow. Biomass use expands—a 50 kW unit in a Thai farm burns palm fronds, supporting a microgrid for 40 homes. Waste heat recovery rises—a 20 kW turbine in a pottery kiln uses 300°C exhaust, saving $9,000 yearly. Innovations aid adoption—3D-printed blades cut costs, sensors lift efficiency to 25%, and coatings handle 400°C, though solar’s $1/W simplicity often prevails. Examples include a 1 kW turbine on a stove (50 W power, 500 W heat) for rural cabins, or a 100 kW unit in a Colombian village burning coffee husks for 70 homes.
Micro steam turbines are small-scale powerhouses, merging steam’s heritage with practical ingenuity. They thrive where fuel is local, power is modest, and flexibility counts—off-grid sites, biomass-rich areas, or waste-heat zones—offering a tailored energy fix as the world diversifies beyond centralized grids.
Non Condensing Steam Turbine
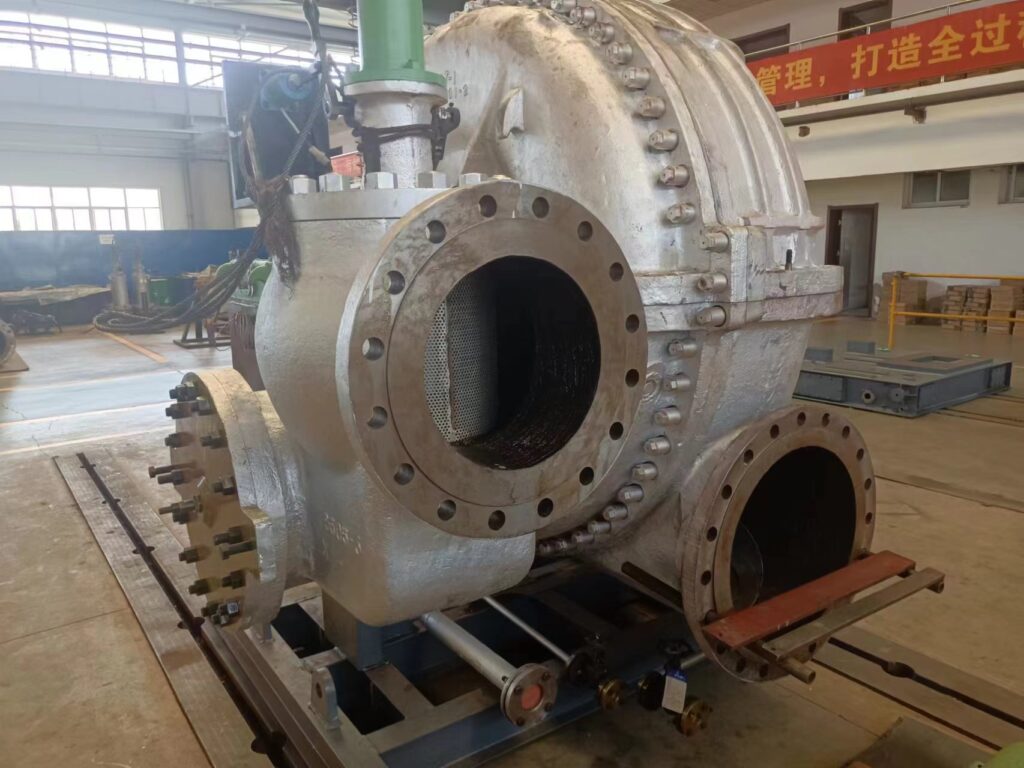
Here’s a detailed exploration of non-condensing steam turbines, presented as a long plain text. Non-condensing steam turbines, also known as back-pressure turbines, are a type of steam turbine where the steam exits at a pressure higher than atmospheric, rather than being condensed into a vacuum as in condensing turbines. These turbines are designed to produce mechanical work—typically to generate electricity or drive machinery—while also supplying usable exhaust steam for industrial processes, heating, or other thermal applications. They are widely used in settings where both power and heat are needed, such as industrial plants, small power stations, and combined heat and power (CHP) systems, with power outputs ranging from a few kilowatts to tens of megawatts. Non-condensing steam turbines are valued for their simplicity, efficiency in CHP applications, and ability to leverage steam as a dual-purpose resource. Let’s dive into their mechanics, design features, applications, operational details, historical context, modern relevance, advantages, and challenges.
The operation of a non-condensing steam turbine follows the Rankine cycle, adapted to prioritize both mechanical power and usable exhaust steam over maximum electrical efficiency. A boiler generates steam by burning fuels like coal, natural gas, oil, biomass (e.g., wood chips, bagasse), or capturing waste heat from industrial processes—say, furnace exhaust at 400-600°C. Steam enters the turbine at pressures typically between 10 and 100 bar and temperatures of 200-540°C, depending on the system’s scale and fuel source. This steam flows over blades attached to a rotor—a steel shaft weighing from a few kilograms in small units to tons in larger ones. As the steam expands—dropping from, say, 50 bar to 5-20 bar—it accelerates to speeds of 300-500 meters per second, pushing the blades and spinning the rotor at speeds like 3,000 RPM for 50 Hz grids, 3,600 RPM for 60 Hz, or up to 10,000 RPM in high-speed designs. The rotor drives a generator to produce electricity or powers machinery like pumps, compressors, or fans via a shaft, often with a gearbox to match equipment speeds. Unlike condensing turbines, the steam does not exhaust into a vacuum (0.05-0.1 bar); instead, it exits at a higher pressure—typically 2-20 bar—determined by downstream needs, such as heating a building, drying materials, or feeding a process like chemical distillation. This exhaust steam retains significant thermal energy, making non-condensing turbines ideal for CHP setups where total energy efficiency is the goal.
The design of non-condensing steam turbines reflects their dual-purpose role and simpler construction compared to condensing systems. They can be single-stage for small applications—say, a 50 kW unit with one blade set—or multi-stage with 5-20 stages for larger outputs like 10 MW, extracting energy gradually across multiple blade rows. Impulse designs are common, especially in smaller units—steam accelerates through nozzles into high-velocity jets that strike curved, bucket-shaped blades, spinning the rotor at high speeds like 10,000-20,000 RPM, a nod to Gustaf de Laval’s 1880s turbine. Reaction designs, inspired by Charles Parsons’ multi-stage concept, dominate in larger non-condensing turbines—steam expands across fixed stator and moving rotor blades, spinning at 3,000-6,000 RPM for efficiency and generator compatibility. Combination designs blend impulse and reaction stages—impulse for high-pressure entry (e.g., 50 bar), reaction for lower-pressure exhaust (e.g., 10 bar)—optimizing energy extraction in mid-sized systems. Blades are sized for the pressure range—5-20 cm in high-pressure sections, up to 50 cm in low-pressure stages—made of stainless steel or nickel alloys (e.g., Inconel) for heat resistance up to 540°C, with coatings like chromium to combat corrosion. Rotors, forged steel, are balanced to handle high speeds, while casings—cast steel or alloys—contain pressures up to 100 bar, with seals like labyrinths or packing glands minimizing leakage, though less critical than in condensing turbines since exhaust pressure is higher.
Non-condensing steam turbine generators pair the turbine with an alternator or dynamo to produce electricity, tailored to the system’s scale. A 1 MW unit might generate 400 V AC for industrial use, while a 50 kW unit produces 230 V AC for a small facility, synchronized at 50 or 60 Hz. Generators are synchronous—rotor poles spin within stator windings—cooled by air or water to manage heat from 50-70% energy losses, with voltage adjustable via regulators. Boilers, producing 10-100 tons/hour of steam, integrate with fuels like natural gas (clean, efficient), biomass (sustainable), or waste heat (cost-free). Unlike condensing systems, non-condensing turbines skip the condenser and cooling towers, reducing complexity—exhaust steam pipes directly to process equipment, radiators, or heat exchangers. Controls vary—manual valves in basic setups, digital systems with sensors in modern units—adjusting steam flow to balance power and heat output, with costs from $10,000 for a 50 kW unit to $1 million for a 10 MW system.
Applications of non-condensing steam turbines center on industries needing both power and heat. Pulp and paper mills use them extensively—a 5 MW turbine burns wood waste, producing 4 MW of electricity and 10 tons/hour of 5-bar steam to dry pulp, leveraging abundant biomass. Chemical plants deploy them for process steam—a 10 MW unit generates 8 MW and 20 tons/hour of 15-bar steam for reactors or distillation, often fueled by natural gas. Sugar mills rely on them with bagasse—a 3 MW turbine powers milling equipment and supplies 8 tons/hour of 10-bar steam for juice evaporation, using cane waste. Textile factories use smaller units—a 500 kW turbine produces 400 kW and 2 tons/hour of 3-bar steam for dyeing or heating, often coal-fired. District heating systems adopt them in cold regions—a 2 MW turbine generates 1.5 MW and heats a small town with 5-bar steam, burning local biomass. Small power plants or cogeneration units use them for grid support—a 20 MW turbine supplies 15 MW and steam for nearby factories, fueled by oil or gas. Off-grid setups benefit too—a 50 kW turbine in a remote farm burns wood, powering tools and heating barns.
Efficiency in non-condensing steam turbines varies by focus. Electrical efficiency is lower than condensing turbines—typically 20-30%—since less steam energy converts to work. A 5 MW unit burning 20 tons/hour of steam (50 bar, 540°C) might produce 1.5 MW (25% efficiency), with 70% of energy exiting as usable 10-bar steam, losing 5-10% to friction and leaks. In CHP mode, total efficiency—power plus heat—reaches 70-85%, far exceeding condensing systems’ 35-45%. For example, a 3 MW turbine converts 25% to electricity (750 kW), 55% to 5-bar steam (1.65 MW thermal), losing 20% to waste. Losses stem from incomplete expansion (exhaust at 5-20 bar vs. 0.05 bar), blade turbulence, and leakage (5-10% with basic seals). Multi-stage designs or superheating to 540°C boost electrical efficiency to 30-35%, but exhaust pressure limits gains—lower exhaust (e.g., 2 bar) increases power but reduces usable heat. Fuel quality affects output—dry biomass (4.5 kWh/kg) outperforms wet (3 kWh/kg), with moisture above 20% cutting steam by 30%.
Historically, non-condensing steam turbines evolved from early steam power needs. Parsons’ 1884 multi-stage turbine and De Laval’s impulse design laid the groundwork, but by the early 20th century, industries adopted them for dual power-and-heat roles, replacing steam engines (5-10% efficiency) with turbines (15-25%). Pre-WWII factories used coal-fired units for machinery and heating, scaling to megawatts by the 1930s. WWII saw them in small plants or ships—e.g., a 1 MW unit powered a factory and warmed it on limited fuel. Post-war, condensing turbines dominated large grids, but the 1970s energy crisis revived non-condensing designs for CHP, especially with biomass and waste heat. Modern units refine this—automation and materials like nickel alloys enhance performance, though they remain niche vs. condensing giants.
Modern relevance ties to energy efficiency and sustainability. Industrial CHP thrives—a 10 MW turbine saves a factory $2-3 million yearly by reusing steam, vs. separate power and heat systems costing 50% more. Biomass use grows—a 5 MW unit burns 2 tons/hour of wood waste, cutting fossil fuel reliance in rural mills. Waste heat recovery expands—a 2 MW turbine uses 400°C exhaust from a steel furnace, generating power and steam emissions-free. District heating in Europe leans on them—a 3 MW turbine heats 500 homes and powers local loads, fueled by gas or biomass. Decentralized power benefits—off-grid sites use 50-500 kW units where grids falter. Innovations help—digital controls optimize steam use, coatings handle 540°C, and modular designs cut costs, though condensing turbines lead in raw power output.
Advantages of non-condensing steam turbines include simplicity—no condenser or cooling towers slashes setup costs ($500-$1,000/kW vs. $1,500-$2,000/kW for condensing). High CHP efficiency (70-85%) maximizes energy use—a 5 MW unit delivers 4 MW and 10 tons/hour of steam, beating standalone generators (30-40%). Fuel flexibility suits local resources—wood, waste, or gas work seamlessly. Compactness fits tight spaces—a 1 MW unit needs 5×10 meters vs. 10×20 for condensing. Reliability endures—20-30 years with maintenance, running 5,000-8,000 hours/year. Sustainability aligns with green goals—biomass units offset CO2, and waste heat cuts fuel bills.
Challenges limit their scope. Lower electrical efficiency (20-30%) caps power output—a 5 MW unit yields 1.5 MW vs. 2 MW from a condensing turbine, needing more fuel (e.g., 2 tons/hour of wood vs. 1.5 tons). Exhaust pressure trade-offs constrain design—5-20 bar balances heat and power but sacrifices electrical gains. Maintenance—blade wear, seal leaks, boiler scaling—costs $10,000-$50,000 yearly for a 5 MW unit, with downtime cutting production. Emissions from biomass (50-200 mg/m³ particulates) need filters ($10,000-$50,000) for compliance (e.g., U.S. EPA 25 mg/m³). Initial costs—$500,000 for 1 MW—lag solar ($1-2/W) for small scales. Fuel logistics—wet biomass or inconsistent heat—drop output by 20-30%.
Examples illustrate their role. A 1 MW turbine in a textile plant burns coal, producing 800 kW and 3 tons/hour of 5-bar steam for dyeing, saving $200,000 yearly. A 10 MW unit in a paper mill uses wood waste, generating 8 MW and 20 tons/hour of 10-bar steam, cutting costs by $2 million. A 50 kW off-grid turbine burns logs, powering a farm and heating it with 2-bar steam, costing $10,000 but saving $5,000 annually.
Non-condensing steam turbines excel where power and heat intertwine, offering a practical, efficient solution for industry and small-scale energy. Their legacy endures, adapting to modern needs with a focus on resourcefulness and sustainability.
Here’s a continued exploration of non-condensing steam turbines, presented as a detailed plain text that builds on the previous discussion while offering deeper insights. Non-condensing steam turbines, often referred to as back-pressure turbines, are a specialized type of steam turbine where the exhaust steam exits at a pressure above atmospheric levels, typically between 2 and 20 bar, rather than being condensed into a vacuum as in condensing turbines. These turbines are engineered to produce mechanical work—usually driving a generator for electricity or powering machinery—while simultaneously providing usable exhaust steam for heating, industrial processes, or other thermal applications. They are particularly suited for combined heat and power (CHP) systems, small industrial plants, and settings where both electrical and thermal energy are valuable, with power outputs ranging from a few kilowatts to tens of megawatts. Non-condensing steam turbines stand out for their simplicity, high total energy efficiency in CHP configurations, and adaptability to diverse fuel sources. Let’s expand further on their mechanics, design characteristics, applications, operational specifics, historical evolution, modern significance, advantages, and challenges.
The mechanics of a non-condensing steam turbine are rooted in the Rankine cycle, tailored to balance mechanical power and thermal output rather than maximizing electrical efficiency alone. A boiler generates steam by combusting fuels such as coal, natural gas, oil, or biomass like wood chips, sawdust, or agricultural residues (e.g., rice husks, sugarcane bagasse), or by harnessing waste heat from industrial processes—imagine exhaust from a kiln at 400-600°C or a diesel engine at 300°C. Steam enters the turbine at pressures typically ranging from 10 to 100 bar and temperatures from 200 to 540°C, depending on the system’s size and fuel quality. This steam flows over blades mounted on a rotor—a forged steel shaft that can weigh a few kilograms in small units or several tons in larger ones. As the steam expands—say, from 50 bar to 10 bar—it accelerates to speeds of 300-500 meters per second, pushing the blades and spinning the rotor at speeds like 3,000 RPM for 50 Hz electrical grids, 3,600 RPM for 60 Hz, or up to 10,000 RPM in high-speed industrial designs. The rotor’s motion drives a generator to produce electricity or powers mechanical equipment such as pumps, compressors, fans, or mills through a shaft, often paired with a gearbox to adjust speed—for example, stepping down 10,000 RPM to 1,500 RPM for a pump. Unlike condensing turbines, which exhaust into a vacuum (0.05-0.1 bar) to extract maximum energy, non-condensing turbines release steam at a higher pressure—2 to 20 bar—set by the needs of downstream applications like heating a factory, drying lumber, or supplying steam for chemical reactions. This exhaust steam retains significant thermal energy, often 50-70% of the input, making these turbines ideal for CHP systems where total energy utilization is prioritized over electrical output alone.
The design of non-condensing steam turbines reflects their practical focus on dual-purpose energy production and reduced complexity compared to condensing systems. They can be single-stage for small-scale use—such as a 50 kW unit with one blade set extracting energy in a single pass—or multi-stage with 5 to 20 stages for larger capacities like 10 MW, allowing progressive energy extraction across multiple blade rows. Impulse designs are frequent in smaller units—steam accelerates through nozzles into high-velocity jets that strike curved, bucket-shaped blades, spinning the rotor at high speeds like 10,000-20,000 RPM, a design echoing Gustaf de Laval’s 1880s impulse turbine. Reaction designs, drawing from Charles Parsons’ multi-stage approach, are more common in larger non-condensing turbines—steam expands across alternating fixed stator and moving rotor blades, spinning at 3,000-6,000 RPM for better efficiency and compatibility with standard generators. Combination designs integrate impulse and reaction stages—impulse for the high-pressure entry (e.g., 50 bar) and reaction for the lower-pressure exhaust (e.g., 10 bar)—optimizing performance in mid-sized systems. Blades vary in size—5-20 cm in high-pressure sections, up to 50 cm in low-pressure stages—crafted from stainless steel for durability or nickel-based alloys like Inconel for heat resistance up to 540°C, often coated with chromium or ceramic to resist corrosion and erosion from wet steam. Rotors, made of forged steel, are precision-balanced to handle high speeds without vibration, while casings—cast steel or alloys—contain pressures up to 100 bar, using seals like labyrinths or packing glands to minimize leakage, though sealing is less critical here since exhaust pressure exceeds atmospheric levels, reducing the pressure differential that drives leaks.
Non-condensing steam turbine generators couple the turbine with an alternator or dynamo to produce electricity, scaled to the system’s needs. A 1 MW unit might generate 400 V AC for industrial machinery, while a 50 kW unit produces 230 V AC for a small facility, synchronized at 50 or 60 Hz for grid or local use. Generators are typically synchronous—rotor poles spin within stator windings—cooled by air or water to dissipate heat from 50-70% energy losses, with voltage adjustable via regulators to match loads. Boilers, producing 10 to 100 tons/hour of steam, integrate with diverse fuels—natural gas offers clean combustion, biomass leverages local waste, and waste heat taps free energy from processes like steelmaking or cement production. Unlike condensing turbines, non-condensing systems eliminate the need for a condenser and associated cooling infrastructure—exhaust steam is piped directly to heat exchangers, process equipment, or heating systems, simplifying installation and maintenance. Control systems range from manual valves and mechanical governors in basic setups to digital controls with sensors in advanced units, adjusting steam flow to optimize the balance between power and heat output, with costs ranging from $10,000 for a 50 kW unit to $1-2 million for a 10 MW system, depending on features and capacity.
Applications of non-condensing steam turbines are centered in industries and settings where both electrical power and thermal energy are in demand. Pulp and paper mills are a prime example—a 5 MW turbine burns wood waste from sawmill operations, producing 4 MW of electricity to run machinery and 10 tons/hour of 5-bar steam to dry paper pulp, capitalizing on abundant biomass to offset grid costs by $1-2 million annually. Chemical plants use them for process steam—a 10 MW turbine generates 8 MW of power and 20 tons/hour of 15-bar steam for reactors, distillation columns, or heating, often fueled by natural gas for consistent output. Sugar mills rely heavily on them with bagasse—a 3 MW turbine powers milling equipment and delivers 8 tons/hour of 10-bar steam to evaporate cane juice, using cane waste to achieve near-self-sufficiency during harvest season. Textile factories employ smaller units—a 500 kW turbine produces 400 kW and 2 tons/hour of 3-bar steam for dyeing fabrics or heating dye vats, often coal-fired in regions with cheap coal access. District heating systems in colder climates adopt them—a 2 MW turbine generates 1.5 MW of electricity and heats a small town with 5-bar steam piped to radiators, burning local biomass like wood pellets for sustainability. Small cogeneration plants use them for grid support—a 20 MW turbine supplies 15 MW to the grid and steam to nearby factories, fueled by oil or gas in urban areas. Off-grid applications benefit as well—a 50 kW turbine in a remote farm burns wood, powering tools and heating livestock barns with 2-bar steam, providing energy independence where grid extension is impractical.
Efficiency in non-condensing steam turbines depends on their operational goals. Electrical efficiency is typically lower than that of condensing turbines—ranging from 20-30%—because the steam expansion stops at a higher exhaust pressure, leaving much of its energy as usable heat. A 5 MW turbine burning 20 tons/hour of steam (50 bar, 540°C) might produce 1.5 MW of electricity (25% efficiency), with 70% of the energy exiting as 10-bar steam (3.5 MW thermal equivalent), and 5-10% lost to friction, turbulence, and leakage. In CHP mode, total efficiency—combining power and heat—reaches 70-85%, far surpassing the 35-45% electrical efficiency of condensing systems. For instance, a 3 MW turbine might convert 25% to electricity (750 kW), 55% to 5-bar steam (1.65 MW thermal), and lose 20% to waste heat. Losses come from incomplete expansion (exhaust at 5-20 bar vs. 0.05 bar in condensing units), blade inefficiencies (less staging than large turbines), and leakage (5-10% with basic seals). Multi-stage designs with 5-20 stages or superheating steam to 540°C can boost electrical efficiency to 30-35%, but lowering exhaust pressure (e.g., to 2 bar) increases power at the expense of usable heat, a trade-off that CHP systems carefully balance. Fuel quality impacts performance—dry biomass at 4.5 kWh/kg outperforms wet biomass at 3 kWh/kg, with moisture levels above 20% reducing steam output by 30% if not pre-dried, a common challenge in biomass-heavy applications.
The historical development of non-condensing steam turbines traces back to the early days of steam power. Charles Parsons’ 1884 multi-stage turbine and Gustaf de Laval’s impulse design provided the foundation, but by the early 20th century, industries adopted non-condensing turbines for their ability to supply both power and heat, outpacing less efficient steam engines (5-10% efficiency) with turbines offering 15-25%. Pre-World War II factories used coal-fired units to drive machinery and heat workspaces, scaling to megawatts by the 1930s as metallurgy improved—chromium steels allowed higher temperatures and pressures. During WWII, non-condensing turbines powered small plants or military facilities—e.g., a 1 MW unit ran a factory and warmed it on rationed fuel, proving their resilience. Post-war, condensing turbines took over large-scale power generation, but the 1970s energy crisis revived non-condensing designs for CHP, particularly with biomass and waste heat as oil prices soared. Modern units enhance this legacy—digital automation optimizes steam use, and materials like nickel alloys withstand 540°C, though they remain a specialized tool compared to condensing systems.
The modern significance of non-condensing steam turbines lies in their efficiency and adaptability in energy-conscious applications. Industrial CHP systems benefit greatly—a 10 MW turbine saves a factory $2-3 million annually by reusing steam for processes, far more cost-effective than separate power generation (30-40% efficiency) and heating systems (50-60% fuel loss). Biomass utilization is on the rise—a 5 MW turbine burns 2 tons/hour of wood waste, reducing fossil fuel dependence in rural industries like sawmills or paper plants, aligning with carbon reduction goals. Waste heat recovery expands their reach—a 2 MW turbine uses 400°C exhaust from a glass furnace, generating power and steam without additional fuel, cutting emissions and costs by $500,000 yearly. District heating systems in Europe and North America rely on them—a 3 MW turbine heats 500 homes and powers local loads with 5-bar steam, often fueled by gas or biomass for reliability and sustainability. Decentralized power applications grow too—a 50-500 kW turbine serves off-grid communities or farms where grid access costs exceed $10,000 per kilometer. Innovations bolster their role—digital controls with real-time sensors balance power and heat, advanced coatings handle higher temperatures, and modular designs reduce installation costs, though condensing turbines dominate in pure power generation.
The advantages of non-condensing steam turbines are numerous and context-specific. Their simplicity—no need for a condenser or cooling towers—reduces initial costs to $500-$1,000 per kW, compared to $1,500-$2,000/kW for condensing systems, and cuts maintenance by eliminating complex cooling infrastructure. High CHP efficiency (70-85%) maximizes energy use—a 5 MW turbine delivers 4 MW of electricity and 10 tons/hour of usable steam, outperforming standalone generators or boilers in total energy output. Fuel flexibility accommodates local resources—wood, agricultural waste, gas, or waste heat work effectively, making them viable in diverse settings like forested regions or industrial zones. Their compact size fits constrained spaces—a 1 MW unit requires a 5×10-meter footprint versus 10×20 meters for a condensing setup, easing installation in factories or small plants. Reliability is strong—units run 20-30 years with regular maintenance, operating 5,000-8,000 hours/year in harsh conditions like dusty mills or humid climates. Sustainability appeals to green initiatives—biomass-driven units achieve near carbon neutrality with regrowth, and waste heat recovery minimizes fuel use, supporting eco-friendly operations.
Challenges, however, temper their broader adoption. Their lower electrical efficiency (20-30%) limits power output—a 5 MW turbine yields 1.5 MW of electricity compared to 2 MW from a condensing turbine with the same input, requiring more fuel (e.g., 2 tons/hour of wood versus 1.5 tons) for equivalent power. The trade-off between exhaust pressure and power constrains design—higher exhaust pressures (10-20 bar) preserve heat but reduce electrical yield, while lower pressures (2-5 bar) boost power at the cost of thermal utility, a delicate balance for CHP optimization. Maintenance needs—blade wear from wet steam, seal leakage, and boiler scaling—cost $10,000-$50,000 annually for a 5 MW unit, with downtime interrupting power or heat supply, a concern in continuous operations. Emissions from biomass fuels—particulates at 50-200 mg/m³ and CO—require filters or scrubbers costing $10,000-$50,000 to meet regulations like the U.S. EPA’s 25 mg/m³ limit, adding expense and complexity. Initial costs, while lower than condensing systems, remain significant—$500,000 for a 1 MW unit can’t compete with solar’s $1-2/W for small-scale power. Fuel logistics pose risks—wet biomass (e.g., 50% moisture) or inconsistent waste heat reduces steam quality, cutting output by 20-30% if not managed with drying or buffering systems.
Examples showcase their practical impact. A 1 MW non-condensing turbine in a textile plant burns coal, producing 800 kW of electricity and 3 tons/hour of 5-bar steam for dyeing, saving $200,000 annually on energy bills with a $400,000 setup cost. A 10 MW turbine in a paper mill uses wood waste, generating 8 MW and 20 tons/hour of 10-bar steam for drying, reducing costs by $2 million yearly against a $2 million investment. A 50 kW off-grid turbine in a rural farm burns logs, powering tools with 40 kW and heating a barn with 2-bar steam, costing $10,000 but saving $5,000 annually over diesel generators.
Non-condensing steam turbines shine where power and heat converge, offering a streamlined, efficient solution for industries, small plants, and off-grid sites. Their evolution—from early 20th-century factories to modern CHP systems—reflects a focus on resource efficiency and adaptability, ensuring they remain relevant in a world balancing energy needs with sustainability.
Condensing Steam Turbine
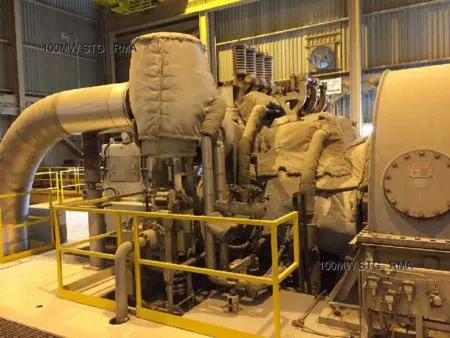
Here’s a detailed exploration of condensing steam turbines, presented as a long plain text. Condensing steam turbines are a type of steam turbine designed to maximize electrical power generation by exhausting steam into a vacuum, typically at 0.05 to 0.1 bar, rather than at a higher pressure as in non-condensing turbines. This low exhaust pressure allows the steam to expand fully, extracting the maximum possible energy to drive a rotor, which in turn powers a generator or machinery. Widely used in utility-scale power plants, large industrial facilities, and some renewable energy systems, condensing steam turbines range in capacity from a few kilowatts to over 1,000 megawatts, with a focus on achieving high electrical efficiency—typically 35-45%—at the expense of rejecting significant heat as waste. They are complex systems requiring boilers, condensers, and cooling infrastructure, making them suited for applications where electricity is the primary goal. Let’s dive into their mechanics, design features, applications, operational details, historical context, modern relevance, advantages, and challenges.
The mechanics of a condensing steam turbine are based on the Rankine cycle, optimized to convert as much thermal energy as possible into mechanical work. A boiler generates steam by burning fuels like coal, natural gas, oil, or biomass (e.g., wood chips, agricultural waste), or using heat from nuclear fission or concentrated solar power, producing steam at high pressures—typically 50 to 300 bar—and temperatures of 400-600°C, depending on the system’s scale and technology. This steam enters the turbine, flowing over blades attached to a rotor—a massive forged steel shaft weighing tons in large units or kilograms in smaller ones. As the steam expands—dropping from, say, 100 bar to 0.05 bar—it accelerates to speeds of 500-700 meters per second, pushing the blades and spinning the rotor at speeds like 3,000 RPM for 50 Hz grids, 3,600 RPM for 60 Hz, or up to 10,000 RPM in smaller, high-speed designs. The rotor drives a generator to produce electricity or, less commonly, powers machinery like pumps or compressors via a shaft, often with a gearbox to adjust speed. Unlike non-condensing turbines, which exhaust at 2-20 bar for heat use, condensing turbines release steam into a condenser—a heat exchanger that cools the steam back to water at 30-50°C using cold water from rivers, oceans, or cooling towers, or air in dry regions—creating a vacuum that maximizes the pressure drop and energy extraction. This condensed water is then pumped back to the boiler, closing the cycle, while the cooling process rejects 50-60% of the input energy as waste heat.
The design of condensing steam turbines is complex and tailored for high efficiency across a wide pressure range. They are typically multi-stage, with 10 to 50 stages grouped into high-pressure (HP), intermediate-pressure (IP), and low-pressure (LP) sections, each optimized for its steam conditions. HP stages handle initial conditions—e.g., 300 bar, 600°C—with small, robust blades (5-20 cm) made of nickel-based alloys like Inconel to withstand extreme heat and pressure, often coated with chromium or ceramics to resist creep and corrosion. IP stages manage reheated steam—say, 50 bar, 540°C—while LP stages process low-pressure, high-volume steam (0.05 bar) with massive blades up to 2 meters long, made of titanium or stainless steel to resist erosion from wet steam at tip speeds of 500-700 m/s. Impulse designs dominate HP stages—steam jets from nozzles strike buckets—while reaction designs prevail in LP stages, where steam expands across moving blades, blending both in combination turbines for optimal energy extraction. Rotors, weighing 10-100 tons in large units, are precision-balanced to spin without vibration, connected to generators via direct shafts or couplings. Casings, forged steel or alloys, contain pressures up to 300 bar, with labyrinth seals minimizing leakage across stages—critical given the huge pressure drop to vacuum. Condensers, often shell-and-tube or surface types, use 1-2 liters of cooling water per kWh, rejecting heat to maintain the vacuum, supported by cooling towers or air fans that evaporate 1-2% of output as waste.
Condensing steam turbine generators pair the turbine with a synchronous generator to produce electricity, scaled to the system’s capacity. A 500 MW unit generates 13.8 kV AC for grid transmission, while a 5 MW industrial unit produces 400 V AC, synchronized at 50 or 60 Hz. Generators feature rotor poles spinning within stator windings, cooled by hydrogen (large units) or air (smaller ones) to dissipate heat from 50-60% losses, with transformers stepping up voltage for distribution. Boilers, producing 100-1,000 tons/hour of steam, integrate with fuels like coal (high energy), gas (clean), or biomass (sustainable), or heat from nuclear reactors or solar mirrors. Cooling systems are extensive—water towers process millions of liters daily, while air-cooled condensers suit arid areas, though less efficient. Controls are sophisticated—digital systems with sensors monitor pressure, temperature, and vacuum, adjusting steam flow and cooling to optimize output, with costs from $50,000 for a 5 MW unit to $50 million for a 500 MW plant.
Applications of condensing steam turbines are primarily in power generation. Utility power plants dominate—coal-fired units (37% of global power) range from 100-1,000 MW, gas-fired combined-cycle plants (25%) hit 500-1,500 MW, and nuclear plants (10%) reach 1,000-1,750 MW, powering millions of homes—e.g., a 1,200 MW nuclear turbine serves 1.5 million households. Large industrial facilities use smaller units—a 5-50 MW turbine powers a steel mill or refinery, fueled by gas or waste heat, supplying all onsite needs. Biomass plants adopt them for renewables—a 10-50 MW unit burns 2-10 tons/hour of wood, supporting rural grids or factories. Geothermal systems use them—a 5-100 MW turbine taps underground steam at 10-50 bar, common in Iceland or the U.S. West. Solar thermal plants employ them—a 50-200 MW unit uses mirrors to heat steam, as in Spain’s solar farms. Off-grid or backup systems use small units—a 50 kW turbine in a remote mine runs on diesel, powering critical loads. Their focus is electricity, not heat, making them less common in CHP compared to non-condensing turbines.
Efficiency in condensing steam turbines is higher than non-condensing types, typically 35-45% for electrical output, thanks to the large pressure drop to vacuum. A 500 MW turbine with supercritical steam (600°C, 300 bar) might convert 45% of fuel energy to electricity, losing 55% to condenser heat, friction, and leaks. Subcritical units (540°C, 100 bar) hit 35-40%, while small units (5 MW, 20 bar) drop to 25-30%. Losses include condenser heat (50-60%), blade turbulence (2-5%), and moisture in LP stages (5-10% output drop unless superheated or dried with separators). Enhancements like reheat—returning steam to the boiler at 20-50 bar for reheating to 540°C—add 4-5% efficiency, common in coal plants. Supercritical or ultra-supercritical steam (600-700°C, 300 bar) pushes efficiency to 47-50%, nearing the Rankine ideal of 60%. Fuel quality matters—coal (30 MJ/kg) outperforms wet biomass (10-15 MJ/kg), with moisture reducing steam output by 20-30% if not managed.
Historically, condensing steam turbines emerged with the rise of centralized power. Parsons’ 1884 turbine pioneered multi-stage design, scaling to megawatts by 1900 as electricity demand grew, outpacing steam engines (5-10% efficiency) with 20-30%. Early 20th-century coal plants used them, with metallurgy advances—nickel steels—enabling higher pressures by the 1930s. WWII saw them in naval ships and power stations, while post-war nuclear and gas plants adopted them, with reheat (1930s) and supercritical steam (1950s) lifting efficiency to 40%+. The 1970s energy crisis spurred efficiency focus, though renewables later diversified their use. Modern designs leverage CFD for blade optimization and AI for control, maintaining their dominance in power generation.
Modern relevance centers on large-scale electricity. Utility plants rely on them—coal, gas, and nuclear units like the 1,750 MW Arabelle power grids, though coal declines with emissions rules (e.g., Paris Agreement). Industrial self-generation grows—a 20 MW turbine cuts a factory’s $5 million yearly grid bill. Renewables expand their role—biomass (10-50 MW), geothermal (5-100 MW), and solar thermal (50-200 MW) support green energy, with a 20 MW wood-fired unit powering 10,000 homes. Innovations enhance them—ceramic blades handle 1,000°C, 3D-printed parts cut costs, and AI optimizes vacuum, though gas turbines challenge them in flexibility. Their scale—1,000 MW+—keeps them vital for baseload power.
Advantages include high electrical efficiency (35-45%), maximizing power—a 500 MW unit outproduces a non-condensing twin by 50%. Large capacity (up to 1,750 MW) suits grids, with a single turbine powering cities. Fuel flexibility spans coal, gas, nuclear, and renewables, adapting to local resources. Longevity—30-50 years—ensures reliability, running 8,000 hours/year. Challenges are complexity—condensers and cooling towers raise costs to $1,500-$2,000/kW vs. $500-$1,000/kW for non-condensing, with $100,000-$1 million yearly maintenance. Water use (1-2 liters/kWh) strains dry regions, and heat rejection (50-60%) wastes energy, unfit for CHP. Slow startups (hours vs. minutes for gas turbines) limit responsiveness.
Examples highlight their impact. A 1,000 MW coal turbine produces 800 MW, powering 1 million homes with a $50 million cost. A 10 MW biomass unit burns 2 tons/hour of wood, generating 8 MW for a rural grid, costing $5 million. A 50 kW diesel-fired turbine in a mine delivers 40 kW, costing $50,000.
Condensing steam turbines are power generation titans, excelling where electricity reigns supreme, their complexity offset by unmatched output and efficiency in a grid-driven world.
Here’s a continued exploration of condensing steam turbines, presented as a detailed plain text that builds on the previous discussion while offering deeper insights. Condensing steam turbines are engineered to maximize electrical power generation by expanding steam to a low-pressure vacuum, typically 0.05 to 0.1 bar, allowing for the greatest possible energy extraction from steam before it is condensed back into water. Unlike non-condensing turbines, which exhaust at higher pressures for heat use, condensing turbines prioritize electricity over thermal output, making them a cornerstone of utility-scale power plants, large industrial facilities, and certain renewable energy systems. With capacities ranging from a few kilowatts to over 1,000 megawatts, they achieve electrical efficiencies of 35-45%, or even higher with advanced designs, at the cost of rejecting significant waste heat. Their complexity—requiring boilers, condensers, and extensive cooling systems—suits applications where power generation is the primary goal. Let’s delve further into their mechanics, design intricacies, applications, operational specifics, historical evolution, modern significance, advantages, and challenges.
The mechanics of a condensing steam turbine are rooted in the Rankine cycle, optimized to extract maximum mechanical work from steam. A boiler generates steam by combusting fuels like coal, natural gas, oil, or biomass (e.g., wood pellets, straw), or harnessing heat from nuclear reactors, geothermal wells, or concentrated solar power, producing steam at pressures of 50 to 300 bar and temperatures of 400-600°C, with supercritical designs reaching 700°C. This high-pressure steam enters the turbine, flowing over blades mounted on a rotor—a massive forged steel shaft that can weigh 10-100 tons in large units or just a few kilograms in smaller ones. As the steam expands—dropping from, say, 100 bar to 0.05 bar—it accelerates to speeds of 500-700 meters per second, pushing the blades and spinning the rotor at speeds like 3,000 RPM for 50 Hz electrical grids, 3,600 RPM for 60 Hz, or up to 10,000 RPM in smaller, high-speed configurations. The rotor drives a generator to produce electricity or, less commonly, powers machinery such as pumps or compressors through a shaft, often with a gearbox to adjust speed—for instance, reducing 10,000 RPM to 1,500 RPM for industrial equipment. The steam then exhausts into a condenser—a heat exchanger that cools it back to water at 30-50°C using cold water from rivers, oceans, or cooling towers, or air in water-scarce areas—creating a vacuum that maximizes the pressure differential and energy yield. This condensed water is pumped back to the boiler, completing the closed cycle, while the cooling process discards 50-60% of the input energy as waste heat, a trade-off for high electrical output.
The design of condensing steam turbines is intricate, built to handle a broad pressure range and optimize efficiency. They are almost always multi-stage, featuring 10 to 50 stages divided into high-pressure (HP), intermediate-pressure (IP), and low-pressure (LP) sections, each tailored to its steam conditions. HP stages manage the initial high-pressure, high-temperature steam—e.g., 300 bar, 600°C—with small, sturdy blades (5-20 cm) made of nickel-based superalloys like Inconel 718 or Rene 41, designed to resist creep and corrosion at extreme conditions, often coated with chromium carbide or ceramics for durability. IP stages process reheated steam—say, 50 bar, 540°C—while LP stages handle low-pressure, high-volume steam (0.05 bar) with enormous blades, sometimes 1.5-2 meters long, made of titanium or stainless steel to withstand erosion from wet steam droplets at tip speeds of 500-700 m/s. Impulse designs are typical in HP stages—steam accelerates through nozzles into jets that strike bucket-shaped blades—while reaction designs dominate LP stages, where steam expands across moving blades, with combination turbines blending both for efficiency across stages. Rotors, weighing tons, are precision-balanced to spin without vibration, connected to generators via direct shafts or couplings. Casings, forged from high-strength steel or alloys, contain pressures up to 300 bar, with labyrinth seals or brush seals minimizing leakage—a critical feature given the steep drop to vacuum. Condensers, often shell-and-tube or surface designs, use 1-2 liters of cooling water per kWh or air fans, maintaining the vacuum while rejecting heat, supported by cooling towers that evaporate 1-2% of output as waste or air systems that reduce water use at a 5-10% efficiency cost.
Condensing steam turbine generators pair the turbine with a synchronous generator to produce electricity, sized for the application. A 500 MW utility unit generates 13.8 kV AC for grid transmission, stepped up to 220-500 kV, while a 5 MW industrial unit produces 400 V AC for local use, synchronized at 50 or 60 Hz. Generators feature rotor poles spinning within stator windings, cooled by hydrogen in large units (better heat transfer) or air in smaller ones, dissipating heat from 50-60% energy losses, with transformers and regulators adjusting output. Boilers, producing 100-1,000 tons/hour of steam, integrate with fuels like coal (30 MJ/kg), natural gas (clean, 50 MJ/kg), or biomass (10-20 MJ/kg), or heat from nuclear reactors (saturated steam at 70 bar) or solar mirrors (400-540°C). Cooling systems are extensive—water-based condensers process millions of liters daily, with cooling towers rejecting heat via evaporation, while air-cooled condensers suit arid regions, though they lower efficiency by 5-10%. Controls are advanced—digital systems with sensors monitor pressure, temperature, vacuum levels, and rotor speed, adjusting steam flow and cooling water to maximize output, with costs ranging from $50,000 for a 5 MW unit to $50-100 million for a 500-1,000 MW plant.
Applications of condensing steam turbines focus on power generation where electricity is the priority. Utility power plants are their stronghold—coal-fired units (37% of global power) range from 100-1,000 MW, gas-fired combined-cycle plants (25%) reach 500-1,500 MW with gas turbine integration, and nuclear plants (10%) hit 1,000-1,750 MW, powering millions—e.g., a 1,200 MW nuclear turbine serves 1.5-2 million homes. Large industrial facilities use them for self-generation—a 5-50 MW turbine powers a chemical plant or steel mill, fueled by gas, coal, or waste heat, meeting onsite demand without grid reliance. Biomass plants leverage them for renewable energy—a 10-50 MW unit burns 2-10 tons/hour of wood or agricultural waste, supplying rural grids or industrial parks, common in Scandinavia or North America. Geothermal systems depend on them—a 5-100 MW turbine uses steam from underground reservoirs at 10-50 bar, as in New Zealand or the U.S. Geothermal Belt. Solar thermal plants employ them—a 50-200 MW unit uses concentrated sunlight to heat steam, as in California’s solar farms. Off-grid or backup systems use smaller units—a 50 kW turbine in a remote mine runs on diesel or biomass, powering critical equipment. Unlike non-condensing turbines, they rarely serve CHP, as their heat is discarded, not reused.
Efficiency in condensing steam turbines is among the highest for steam-based systems, typically 35-45% for electrical output, driven by the large pressure drop to vacuum. A 500 MW turbine with supercritical steam (600°C, 300 bar) might achieve 45% efficiency, converting 45% of fuel energy to electricity and losing 55% to condenser heat, friction, and leaks. Subcritical units (540°C, 100 bar) reach 35-40%, while small units (5 MW, 20 bar) drop to 25-30% due to scale and simpler staging. Losses include condenser heat rejection (50-60%), blade turbulence (2-5%), and moisture in LP stages (5-10% output reduction unless superheated to 540-600°C or dried with moisture separators). Reheat cycles—returning steam to the boiler at 20-50 bar for reheating to 540°C—boost efficiency by 4-5%, standard in coal and nuclear plants, while double reheat adds another 2-3% in ultra-efficient designs. Supercritical (600°C, 300 bar) and ultra-supercritical (700°C, 350 bar) steam push efficiency to 47-50%, nearing the Rankine ideal of 60%, though material limits (creep, corrosion) cap gains. Fuel quality impacts output—coal (30 MJ/kg) or gas (50 MJ/kg) outperforms wet biomass (10-15 MJ/kg), with high moisture (20-50%) cutting steam production by 20-30% unless pre-dried.
Historically, condensing steam turbines emerged as electricity demand surged. Charles Parsons’ 1884 multi-stage turbine laid the foundation, generating 7.5 kW and proving the concept, scaling to megawatts by 1900 as grids expanded, surpassing steam engines (5-10% efficiency) with 20-30%. Early 20th-century coal plants adopted them, with metallurgy advances—nickel and chromium steels—enabling higher pressures and temperatures by the 1930s. World War II saw them in naval ships (e.g., 29,000 hp on the Titanic) and power stations, while post-war nuclear plants (1950s) and gas combined-cycle systems (1970s) embraced them, with reheat (1930s) and supercritical steam (1950s) lifting efficiency to 40%+. The 1970s energy crisis drove efficiency improvements, and renewables later diversified their fuel sources. Modern designs use computational fluid dynamics (CFD) for blade optimization, advanced alloys for durability, and AI for real-time control, cementing their role in power generation.
Modern significance reflects their dominance in electricity production. Utility plants depend on them—coal, gas, and nuclear units like the 1,750 MW Arabelle turbine power grids, though coal’s share shrinks under emissions policies (e.g., net-zero by 2050 goals). Industrial self-generation rises—a 20 MW turbine saves a refinery $5-10 million yearly vs. grid power, fueled by gas or waste heat. Renewables expand their scope—biomass (10-50 MW), geothermal (5-100 MW), and solar thermal (50-200 MW) align with green energy, with a 20 MW biomass unit powering 10,000 homes on 4 tons/hour of wood. Innovations keep them competitive—ceramic blades tolerate 1,000°C, 3D-printed components reduce costs by 10-20%, and AI maintains vacuum efficiency, though gas turbines (faster startups) and solar (lower cost/W) challenge them. Their scale—500-1,000 MW+—ensures they remain critical for baseload power in a decarbonizing world.
Advantages include high electrical efficiency (35-45%), outpacing non-condensing turbines (20-30%)—a 500 MW unit produces 225 MW vs. 150 MW from a non-condensing peer. Massive capacity (up to 1,750 MW) powers entire regions—a single turbine supports 2 million homes. Fuel flexibility spans coal, gas, nuclear, biomass, and solar, adapting to local conditions. Longevity—30-50 years with maintenance—delivers reliability, running 8,000 hours/year. Challenges are significant—complexity drives costs to $1,500-$2,000/kW vs. $500-$1,000/kW for non-condensing, with $100,000-$1 million yearly maintenance for condensers, blades, and boilers. Water demand (1-2 liters/kWh) strains arid areas—e.g., a 500 MW plant uses 2 million liters/hour—while air cooling cuts efficiency by 5-10%. Heat rejection (50-60%) wastes energy, making them unfit for CHP, and slow startups (hours vs. minutes for gas turbines) limit flexibility.
Examples illustrate their scope. A 1,000 MW coal turbine generates 800 MW, powering 1 million homes with a $50-70 million cost. A 10 MW biomass unit burns 2 tons/hour of wood, producing 8 MW for a rural grid, costing $5-7 million. A 50 kW diesel-fired turbine in a mine delivers 40 kW, costing $50,000 but saving $20,000 yearly vs. generators.
Condensing steam turbines are power generation heavyweights, excelling where electricity is king, their intricate design and high output anchoring grids and industries despite a shifting energy landscape.
Automatic Extraction Non Condensing Turbine
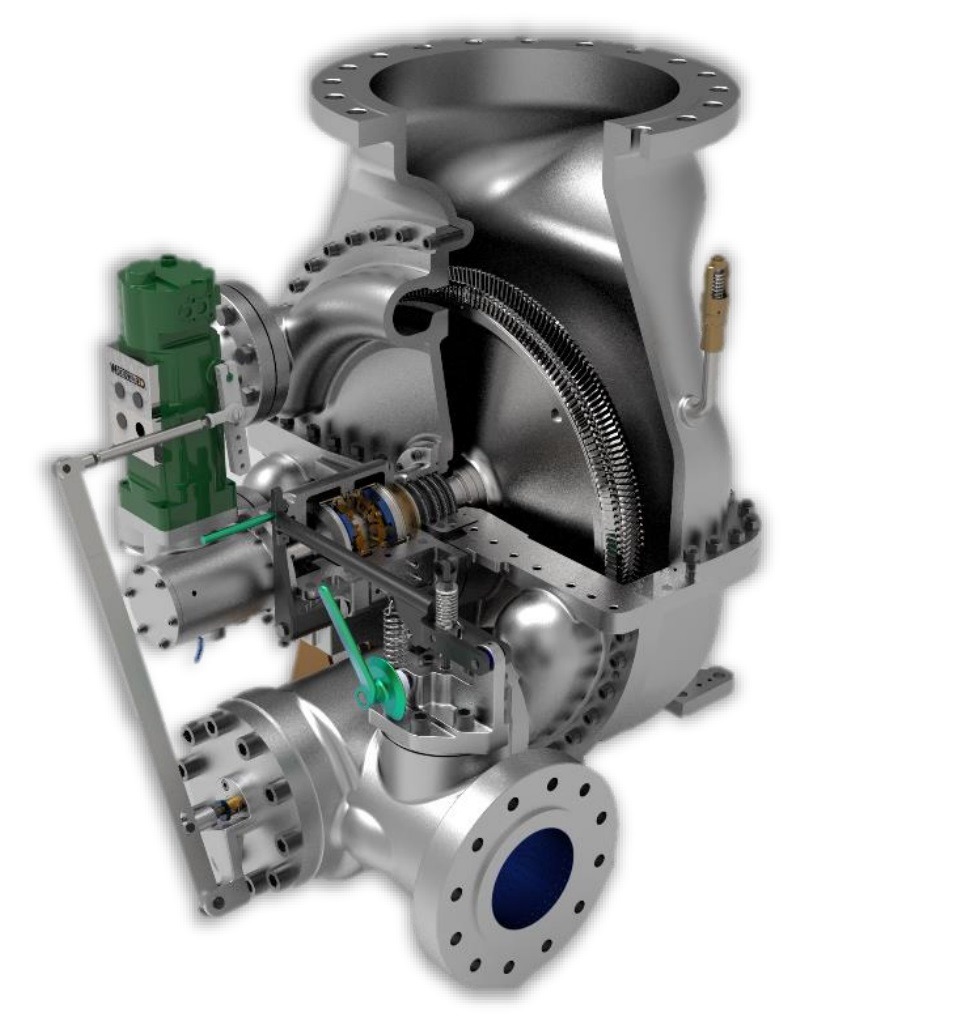
Here’s a detailed exploration of automatic extraction non-condensing steam turbines, presented as a long plain text. Automatic extraction non-condensing steam turbines, also known as extraction back-pressure turbines, are a specialized type of steam turbine designed to produce mechanical power—typically for electricity generation or driving machinery—while simultaneously extracting steam at one or more intermediate pressures for industrial processes or heating, with the final exhaust exiting at a pressure above atmospheric levels, usually 2-20 bar. Unlike condensing turbines, which exhaust into a vacuum to maximize power, these turbines prioritize flexibility, balancing electrical output with the delivery of usable steam at specific pressures tailored to downstream needs. They are widely used in industrial settings like chemical plants, refineries, and paper mills, as well as in combined heat and power (CHP) systems, with capacities ranging from a few kilowatts to tens of megawatts. The “automatic” feature refers to sophisticated control systems that adjust extraction flows in real time, ensuring optimal performance under varying demands. Let’s dive into their mechanics, design features, applications, operational details, historical context, modern relevance, advantages, and challenges.
The mechanics of an automatic extraction non-condensing steam turbine are based on the Rankine cycle, modified to allow controlled steam extraction and a non-condensing exhaust. A boiler generates steam by burning fuels such as coal, natural gas, oil, or biomass (e.g., wood chips, bagasse), or capturing waste heat from industrial processes—say, exhaust from a furnace at 400-600°C. Steam enters the turbine at high pressures, typically 20-100 bar, and temperatures of 200-540°C, depending on the system’s scale and fuel source. This steam flows over blades mounted on a rotor—a forged steel shaft weighing from a few kilograms in small units to several tons in larger ones. As the steam expands—dropping from, say, 50 bar to an extraction point at 15 bar, then to a final exhaust at 5 bar—it accelerates to speeds of 300-500 meters per second, pushing the blades and spinning the rotor at speeds like 3,000 RPM for 50 Hz grids, 3,600 RPM for 60 Hz, or up to 10,000 RPM in high-speed designs. The rotor drives a generator to produce electricity or powers machinery like pumps or compressors via a shaft, often with a gearbox to adjust speed. Steam is extracted at one or more intermediate stages—e.g., 15 bar for a chemical process, 3 bar for heating—through extraction ports controlled by automatic valves, with the remaining steam exhausting at a higher-than-atmospheric pressure (2-20 bar) for additional thermal use, such as drying or district heating. This dual-purpose operation, managed by automated controls, distinguishes them from simpler non-condensing turbines.
The design of automatic extraction non-condensing steam turbines is complex, blending flexibility with efficiency. They are multi-stage, typically with 5-20 stages divided into sections: an initial high-pressure (HP) section before the first extraction, intermediate sections for each extraction point, and a final low-pressure (LP) section before exhaust. HP stages handle entry conditions—e.g., 50 bar, 540°C—with small blades (5-20 cm) made of nickel alloys like Inconel to resist heat and pressure, coated with chromium for durability. Extraction stages manage mid-range pressures—e.g., 15 bar—with blades sized accordingly (10-30 cm), while LP stages process the final expansion to 5-20 bar with larger blades (up to 50 cm), often stainless steel to handle wetter steam. Impulse designs are used in HP stages—steam jets strike buckets—while reaction designs dominate LP stages, with combination turbines blending both for efficiency across pressure drops. Extraction ports, fitted with throttling valves or nozzles, divert steam to external piping, with flow rates adjusted by automatic control systems—e.g., PLCs or DCS—responsive to downstream demand signals. Rotors, forged steel, are balanced for high speeds, while casings—cast steel or alloys—contain 100 bar, with labyrinth seals minimizing leakage, less critical here than in condensing turbines due to higher exhaust pressure. No condenser is needed, simplifying the system—exhaust steam pipes directly to process or heating loads.
Automatic extraction non-condensing steam turbine generators pair the turbine with a synchronous generator, sized for the application. A 10 MW unit might produce 400 V or 13.8 kV AC, synchronized at 50 or 60 Hz, while a 500 kW unit generates 230 V AC for local use. Generators feature rotor poles spinning in stator windings, cooled by air or water, with voltage regulators adjusting output as extraction varies power. Boilers, producing 10-100 tons/hour, use fuels like natural gas (clean), biomass (local), or waste heat (free). Controls are the heart of the “automatic” feature—sensors monitor steam pressure, temperature, and flow at extraction points and exhaust, feeding data to a control system that adjusts valves in real time, ensuring stable power and steam delivery under fluctuating loads. Costs range from $50,000 for a 500 kW unit to $2-5 million for a 10-20 MW system, reflecting added complexity.
Applications center on industries needing variable steam and power. Chemical plants use them extensively—a 10 MW turbine extracts 15-bar steam (10 tons/hour) for reactors and 3-bar steam (5 tons/hour) for heating, producing 7 MW of electricity, fueled by gas or waste heat. Refineries rely on them—a 20 MW unit extracts 20-bar steam for cracking and 5-bar steam for distillation, generating 15 MW, often burning refinery byproducts. Paper mills adopt them—a 5 MW turbine extracts 10-bar steam (8 tons/hour) to dry pulp and exhausts at 2 bar for heating, producing 4 MW from wood waste. Sugar mills use them with bagasse—a 3 MW turbine extracts 15-bar steam for evaporators and exhausts at 5 bar, generating 2 MW. Food processing plants employ smaller units—a 1 MW turbine extracts 5-bar steam for sterilization and exhausts at 2 bar, producing 800 kW. District heating with power generation uses them—a 5 MW turbine extracts 10-bar steam for industrial use and exhausts at 3 bar for homes, generating 4 MW. Off-grid setups benefit too—a 100 kW turbine extracts 5-bar steam for a farm process and exhausts at 2 bar, powering 80 kW.
Efficiency balances power and heat. Electrical efficiency is 20-35%, lower than condensing turbines (35-45%) due to extraction and higher exhaust pressure. A 10 MW turbine (50 bar, 540°C) might produce 3 MW (30% efficiency), with 40% as extracted steam (15 bar), 25% as exhaust steam (5 bar), and 5-10% lost to leaks and friction. Total CHP efficiency hits 70-85%, excelling in energy use—e.g., a 5 MW unit yields 1.5 MW power, 2 MW extracted steam, and 1 MW exhaust heat. Losses include incomplete expansion (5-20 bar exhaust vs. 0.05 bar), blade inefficiencies, and leakage (5-10%). Multi-stage designs and superheating to 540°C boost electrical efficiency to 35%, but extraction reduces available steam for power. Fuel quality matters—dry biomass (4.5 kWh/kg) outperforms wet (3 kWh/kg), with 20%+ moisture cutting output by 30%.
Historically, these turbines evolved from early 20th-century needs for flexible steam use. Parsons’ 1884 multi-stage design and De Laval’s impulse turbine enabled extraction concepts, adopted by industries by the 1920s over steam engines (5-10% efficiency) for 15-25% efficiency plus heat. Pre-WWII plants used coal-fired units for power and process steam, scaling up by the 1930s. WWII saw them in factories needing adaptability, while post-war automation (1950s-60s) added control sophistication. The 1970s energy crisis boosted CHP use, with biomass and waste heat rising. Modern designs leverage digital controls and alloys for efficiency.
Modern relevance lies in industrial flexibility and CHP. Chemical and refining sectors save $1-3 million yearly—a 10 MW turbine meets variable steam needs cost-effectively. Biomass use grows—a 5 MW unit burns 2 tons/hour of waste, cutting fossil fuel costs. Waste heat recovery expands—a 2 MW turbine uses 400°C exhaust, saving $500,000 annually. District heating pairs them with power—a 5 MW unit heats 1,000 homes and generates 4 MW. Automation—PLCs, sensors—optimizes performance, while coatings handle 540°C, though condensing turbines lead in pure power.
Advantages include flexibility—automatic extraction meets shifting demands, e.g., 15-bar to 5-bar steam on the fly. High CHP efficiency (70-85%) maximizes energy—a 5 MW unit delivers 4 MW total output. Simplicity—no condenser—cuts costs to $500-$1,000/kW vs. $1,500-$2,000/kW for condensing. Fuel flexibility suits coal, biomass, or waste heat. Reliability lasts 20-30 years, running 5,000-8,000 hours/year. Challenges are lower electrical efficiency (20-35%)—a 10 MW unit yields 3 MW vs. 4-5 MW condensing. Complexity of controls raises costs—$50,000-$100,000 for automation. Maintenance—valves, blades, seals—costs $10,000-$50,000 yearly. Biomass emissions (50-200 mg/m³) need filters ($10,000-$50,000). Fuel inconsistency cuts output by 20-30%.
Examples show their value. A 10 MW refinery turbine extracts 20-bar and 5-bar steam, producing 7 MW, saving $2 million yearly. A 1 MW food plant unit extracts 5-bar steam, generates 800 kW, costing $100,000 but saving $50,000 annually. A 5 MW paper mill turbine extracts 10-bar steam, exhausts at 2 bar, producing 4 MW from wood, saving $1 million.
Automatic extraction non-condensing turbines excel in balancing power and steam, their adaptability and efficiency making them vital for industries needing both, bridging legacy steam tech with modern demands.
Here’s a continued exploration of automatic extraction non-condensing steam turbines, presented as a detailed plain text that builds on the previous discussion with added depth and nuance. Automatic extraction non-condensing steam turbines, often called extraction back-pressure turbines, are advanced steam turbines designed to generate mechanical power—typically for electricity or machinery—while extracting steam at one or more intermediate pressures for industrial processes or heating, with the final exhaust exiting at a pressure above atmospheric levels, usually 2-20 bar. Unlike condensing turbines that exhaust into a vacuum for maximum power, these turbines offer a flexible balance between electrical output and thermal energy delivery, with automatic control systems adjusting extraction flows to meet real-time demands. They are ideal for industrial applications like refineries, chemical plants, and pulp mills, as well as combined heat and power (CHP) setups, with capacities ranging from a few kilowatts to tens of megawatts. Their ability to adapt to variable steam needs while maintaining efficiency makes them a standout choice in complex energy systems. Let’s delve deeper into their mechanics, design intricacies, applications, operational specifics, historical evolution, modern significance, advantages, and challenges.
The mechanics of an automatic extraction non-condensing steam turbine follow the Rankine cycle, tailored to provide both power and controlled steam output. A boiler produces steam by combusting fuels such as coal, natural gas, oil, or biomass (e.g., wood pellets, rice husks, or sugarcane bagasse), or by capturing waste heat from sources like industrial exhausts at 400-600°C or engine tailpipes at 300-400°C. Steam enters the turbine at high pressures—typically 20-100 bar—and temperatures of 200-540°C, depending on the system’s design and fuel. This steam flows over blades mounted on a rotor—a forged steel shaft ranging from a few kilograms in small units to several tons in larger ones. As the steam expands—say, from 50 bar to an extraction point at 15 bar, then to a final exhaust at 5 bar—it accelerates to speeds of 300-500 meters per second, driving the blades and spinning the rotor at speeds like 3,000 RPM for 50 Hz grids, 3,600 RPM for 60 Hz, or up to 10,000 RPM in high-speed configurations. The rotor powers a generator for electricity or drives machinery like pumps, fans, or compressors via a shaft, often with a gearbox—e.g., reducing 10,000 RPM to 1,500 RPM for a pump. Steam is extracted at intermediate stages—e.g., 15 bar for a process, 3 bar for preheating—through ports with automatic valves, while the remaining steam exhausts at 2-20 bar for additional thermal use, such as drying or heating. Automated controls—sensors and actuators tied to a programmable logic controller (PLC) or distributed control system (DCS)—adjust extraction flows dynamically, ensuring stable power and steam delivery as demands shift, a key feature setting them apart from manual extraction turbines.
The design of these turbines is sophisticated, engineered for flexibility and multi-stage operation. They feature 5-20 stages, segmented into high-pressure (HP) sections before the first extraction, intermediate sections for each extraction point, and a low-pressure (LP) section before exhaust. HP stages handle inlet steam—e.g., 50 bar, 540°C—with small blades (5-20 cm) made of nickel-based alloys like Inconel 718 or Hastelloy, resistant to 540°C and 100 bar, often coated with chromium carbide or ceramics to combat creep and corrosion. Extraction stages manage mid-range pressures—e.g., 15 bar or 5 bar—with blades (10-30 cm) sized for flow, while LP stages process the final drop to 5-20 bar with larger blades (up to 50 cm), typically stainless steel to handle wetter steam. Impulse designs dominate HP stages—steam jets from nozzles strike buckets—while reaction designs prevail in LP stages, with steam expanding across moving blades; combination turbines blend both for efficiency across the pressure range. Extraction ports, equipped with throttling valves or adjustable nozzles, divert steam to external piping, controlled by servo-motors linked to the automation system, which responds to pressure, temperature, or flow signals from downstream processes. Rotors, forged steel, are balanced for high speeds, while casings—cast steel or alloys—contain up to 100 bar, with labyrinth seals or packing glands reducing leakage, though less critical than in condensing turbines due to the higher exhaust pressure. No condenser is required—exhaust steam feeds directly into process lines or heat exchangers, simplifying the setup compared to condensing systems.
Automatic extraction non-condensing steam turbine generators couple the turbine with a synchronous generator, scaled to the system’s needs. A 10 MW unit might produce 400 V or 13.8 kV AC for industrial or grid use, synchronized at 50 or 60 Hz, while a 500 kW unit generates 230 V AC for smaller loads. Generators feature rotor poles spinning within stator windings, cooled by air (smaller units) or water (larger ones) to manage heat from 50-70% energy losses, with voltage regulators adjusting output as extraction alters power. Boilers, producing 10-100 tons/hour, integrate with fuels like natural gas (high efficiency), biomass (local sustainability), or waste heat (cost-free energy). The automation system is central—sensors at extraction points and exhaust monitor conditions, feeding data to a PLC or DCS that adjusts valves in milliseconds, balancing power and steam under variable loads—e.g., increasing 15-bar extraction when a reactor demands more, reducing it when power is prioritized. Costs reflect this complexity—$50,000-$100,000 for a 500 kW unit, $2-5 million for a 10-20 MW system, driven by controls and staging.
Applications focus on industries with fluctuating steam and power needs. Chemical plants are a key user—a 10 MW turbine extracts 15-bar steam (10 tons/hour) for reactors and 3-bar steam (5 tons/hour) for preheating, generating 7 MW, fueled by gas or process waste heat. Oil refineries depend on them—a 20 MW turbine extracts 20-bar steam for cracking units and 5-bar steam for distillation, producing 15 MW, often using byproduct fuels like petroleum coke. Pulp and paper mills leverage them—a 5 MW turbine extracts 10-bar steam (8 tons/hour) to dry pulp and exhausts at 2 bar for mill heating, generating 4 MW from wood waste. Sugar mills use them with bagasse—a 3 MW turbine extracts 15-bar steam for evaporators and exhausts at 5 bar, producing 2 MW during harvest. Food processing benefits—a 1 MW turbine extracts 5-bar steam for sterilization and exhausts at 2 bar for drying, generating 800 kW, often gas-fired. District heating with cogeneration employs them—a 5 MW turbine extracts 10-bar steam for industrial clients and exhausts at 3 bar for residential heating, producing 4 MW. Off-grid sites use smaller units—a 100 kW turbine extracts 5-bar steam for a farm process and exhausts at 2 bar, generating 80 kW from wood or biogas.
Efficiency reflects their dual role. Electrical efficiency ranges from 20-35%, lower than condensing turbines (35-45%) due to extraction and higher exhaust pressure. A 10 MW turbine (50 bar, 540°C) might produce 3 MW (30% efficiency), with 40% as extracted steam (15 bar, 4 MW thermal), 25% as exhaust steam (5 bar, 2.5 MW thermal), and 5-10% lost to friction, turbulence, and leaks. Total CHP efficiency reaches 70-85%, optimizing energy use—e.g., a 5 MW unit yields 1.5 MW power, 2 MW extracted steam, and 1 MW exhaust heat. Losses stem from incomplete expansion (5-20 bar exhaust vs. 0.05 bar), blade inefficiencies (fewer stages than condensing units), and leakage (5-10% with basic seals). Multi-stage designs (10-20 stages) and superheating to 540°C lift electrical efficiency to 35%, but extraction reduces steam available for power, a deliberate trade-off. Fuel quality impacts output—dry biomass (4.5 kWh/kg) outperforms wet (3 kWh/kg), with moisture above 20% cutting steam by 30% unless pre-dried, a frequent issue in biomass systems.
Historically, these turbines emerged from early 20th-century industrial demands for flexible steam. Charles Parsons’ 1884 multi-stage turbine enabled extraction concepts, with De Laval’s impulse design adding speed, adopted by factories by the 1920s for 15-25% efficiency plus heat, outpacing steam engines (5-10%). Pre-WWII plants used coal-fired units for power and process steam, scaling up by the 1930s with better steels. WWII saw them in adaptable factory setups—e.g., a 1 MW unit powered and heated a site on limited fuel. Post-war automation (1950s-60s) introduced valve controls, while the 1970s energy crisis boosted CHP use with biomass and waste heat. Modern designs enhance this—digital systems optimize extraction, and alloys handle 540°C, refining their niche.
Modern significance lies in industrial adaptability and CHP efficiency. Chemical and refining industries save $1-3 million yearly—a 10 MW turbine adjusts steam on demand, cutting costs vs. separate systems. Biomass adoption rises—a 5 MW unit burns 2 tons/hour of waste, reducing fossil fuel use in rural mills. Waste heat recovery grows—a 2 MW turbine uses 400°C exhaust, saving $500,000 annually emissions-free. District heating pairs power and heat—a 5 MW unit serves 1,000 homes and generates 4 MW. Automation ensures precision—sensors and PLCs adapt to load shifts, while coatings and modular designs improve durability and cost, though condensing turbines dominate pure power output.
Advantages include unmatched flexibility—automatic extraction adjusts steam from 20 bar to 2 bar instantly, meeting variable needs. High CHP efficiency (70-85%) maximizes energy—a 5 MW unit delivers 4 MW total output vs. 1.5 MW from a standalone generator. Simplicity—no condenser—cuts costs to $500-$1,000/kW vs. $1,500-$2,000/kW for condensing, easing maintenance. Fuel flexibility spans coal, biomass, and waste heat, fitting local resources. Reliability endures—20-30 years, 5,000-8,000 hours/year. Challenges include lower electrical efficiency (20-35%)—a 10 MW unit yields 3 MW vs. 4-5 MW condensing. Control complexity adds $50,000-$100,000 to costs. Maintenance—valves, blades, seals—runs $10,000-$50,000 yearly. Biomass emissions (50-200 mg/m³) need filters ($10,000-$50,000). Fuel variability cuts output by 20-30% if inconsistent.
Examples highlight their role. A 10 MW refinery turbine extracts 20-bar and 5-bar steam, produces 7 MW, saving $2 million yearly. A 1 MW food plant unit extracts 5-bar steam, generates 800 kW, costing $100,000 but saving $50,000 annually. A 5 MW paper mill turbine extracts 10-bar steam, exhausts at 2 bar, produces 4 MW from wood, saving $1 million.
Automatic extraction non-condensing turbines bridge power and process needs with precision, their adaptability and efficiency anchoring complex industrial energy systems in a versatile, sustainable way.
Automatic Extraction Condensing Turbine
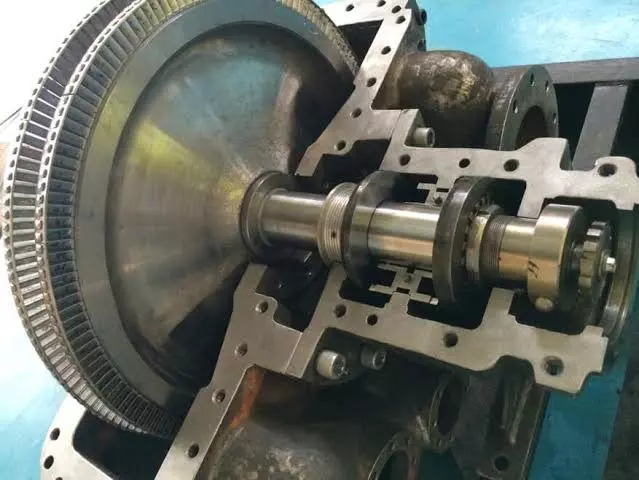
Here’s a detailed exploration of automatic extraction condensing steam turbines, presented as a long plain text. Automatic extraction condensing steam turbines are a sophisticated hybrid of steam turbine designs, combining the ability to extract steam at one or more intermediate pressures for industrial processes or heating with the capability to exhaust the remaining steam into a vacuum, typically 0.05 to 0.1 bar, to maximize electrical power generation. These turbines are engineered to provide both mechanical power—usually driving a generator—and controlled steam output, with automatic systems adjusting extraction flows to meet fluctuating demands, making them highly versatile. They are commonly used in large industrial facilities like refineries, chemical plants, and power plants with CHP (combined heat and power) needs, with capacities ranging from a few megawatts to hundreds of megawatts. Their dual focus on high electrical efficiency and process steam delivery sets them apart from simpler condensing or non-condensing turbines. Let’s dive into their mechanics, design features, applications, operational details, historical context, modern relevance, advantages, and challenges.
The mechanics of an automatic extraction condensing steam turbine are based on the Rankine cycle, adapted to balance maximum power extraction with controlled steam delivery. A boiler generates steam by combusting fuels like coal, natural gas, oil, or biomass (e.g., wood chips, bagasse), or using heat from nuclear reactors, waste heat recovery (e.g., 400-600°C industrial exhaust), or solar thermal systems, producing steam at pressures of 50-300 bar and temperatures of 400-600°C, with advanced units reaching 700°C in supercritical designs. This steam enters the turbine, flowing over blades on a rotor—a forged steel shaft weighing tons in large units or kilograms in smaller ones. As the steam expands—say, from 100 bar to an extraction point at 15 bar, then to a final exhaust at 0.05 bar—it accelerates to speeds of 500-700 meters per second, driving the blades and spinning the rotor at speeds like 3,000 RPM for 50 Hz grids, 3,600 RPM for 60 Hz, or up to 10,000 RPM in smaller setups. The rotor powers a generator for electricity or drives machinery via a shaft, often with a gearbox—e.g., reducing 10,000 RPM to 1,500 RPM. Steam is extracted at intermediate stages—e.g., 15 bar for a process, 3 bar for heating—through ports with automatic valves, while the remaining steam exhausts into a condenser, cooling back to water at 30-50°C under vacuum conditions using water from rivers or cooling towers, or air in dry regions. This vacuum maximizes the pressure drop and power output, while automated controls—PLCs or DCS—adjust extraction flows in real time, responding to changes in steam or power demand, ensuring stability and efficiency.
The design of these turbines is complex, integrating multi-stage expansion with extraction and condensing features. They feature 10-50 stages, divided into high-pressure (HP) sections before the first extraction, intermediate sections for extraction points, and low-pressure (LP) sections leading to the condenser. HP stages handle inlet steam—e.g., 100 bar, 600°C—with small blades (5-20 cm) made of nickel-based superalloys like Inconel or Rene 41, resistant to extreme conditions, coated with chromium or ceramics for durability. Extraction stages manage mid-range pressures—e.g., 15 bar or 5 bar—with blades (10-30 cm) sized for flow, while LP stages process the final drop to 0.05 bar with large blades (up to 2 meters), typically titanium or stainless steel to resist wet steam erosion at tip speeds of 500-700 m/s. Impulse designs dominate HP stages—steam jets strike buckets—while reaction designs prevail in LP stages, with combination turbines blending both for efficiency. Extraction ports, fitted with throttling valves or nozzles, divert steam to external piping, controlled by servo-motors tied to an automation system that adjusts based on pressure, temperature, or flow signals. Rotors, weighing 10-100 tons in large units, are balanced for high speeds, while casings—forged steel or alloys—contain up to 300 bar, with labyrinth seals minimizing leakage across stages, critical due to the steep pressure drop to vacuum. Condensers—shell-and-tube or surface types—use 1-2 liters of cooling water per kWh or air fans, maintaining the vacuum and rejecting 50-60% of heat as waste.
Automatic extraction condensing steam turbine generators pair the turbine with a synchronous generator, scaled to the system’s capacity. A 50 MW unit might produce 13.8 kV AC for grid transmission, while a 5 MW unit generates 400 V AC for industrial use, synchronized at 50 or 60 Hz. Generators feature rotor poles spinning in stator windings, cooled by hydrogen (large units) or air (smaller ones), with voltage regulators adjusting output as extraction alters power. Boilers, producing 10-1,000 tons/hour, use fuels like coal (30 MJ/kg), gas (50 MJ/kg), or biomass (10-20 MJ/kg), or heat from nuclear or solar sources. Cooling systems are extensive—water towers process millions of liters daily, air-cooled condensers suit arid areas at a 5-10% efficiency cost. Automation is key—sensors monitor extraction points, exhaust vacuum, and rotor speed, feeding data to a PLC or DCS that adjusts valves in milliseconds, optimizing power and steam under variable loads, with costs from $1-2 million for a 5 MW unit to $50-100 million for a 50-100 MW system.
Applications focus on large industrial and power generation settings with dual needs. Refineries use them extensively—a 50 MW turbine extracts 20-bar steam (20 tons/hour) for cracking and 5-bar steam (10 tons/hour) for distillation, generating 40 MW, fueled by gas or byproduct fuels. Chemical plants rely on them—a 20 MW turbine extracts 15-bar steam (15 tons/hour) for reactors and 3-bar steam (5 tons/hour) for heating, producing 15 MW, often using waste heat. Pulp and paper mills adopt them—a 10 MW turbine extracts 10-bar steam (10 tons/hour) to dry pulp, generating 8 MW from wood waste, with exhaust to a condenser. Power plants with CHP use them—a 100 MW turbine extracts 15-bar steam for industrial clients and generates 80 MW for the grid, fueled by coal or gas. Food processing benefits—a 5 MW turbine extracts 5-bar steam for sterilization, producing 4 MW, often gas-fired. Biomass plants employ them—a 20 MW turbine extracts 10-bar steam for factory use and generates 15 MW from wood, supporting rural grids. Their ability to serve both power and steam makes them versatile, though less common in pure power plants than straight condensing turbines.
Efficiency balances power and extraction. Electrical efficiency is 30-40%, lower than pure condensing turbines (35-45%) due to steam diverted for extraction. A 20 MW turbine (100 bar, 540°C) might produce 8 MW (40% efficiency without extraction), but with 15-bar and 5-bar extraction, it yields 6 MW (30% efficiency), with 40% as extracted steam (8 MW thermal) and 20-25% lost to condenser heat and friction. Total CHP efficiency reaches 70-80%, leveraging steam use—e.g., a 10 MW unit yields 3 MW power and 6 MW thermal. Losses include condenser heat (50-60% without extraction), blade turbulence (2-5%), and moisture in LP stages (5-10% unless superheated to 540°C). Reheat—returning steam to 540°C at 20-50 bar—boosts efficiency by 4-5%, common in large units. Fuel quality affects output—coal outperforms wet biomass, with 20%+ moisture cutting steam by 30%.
Historically, these turbines evolved from early 20th-century multi-stage designs. Parsons’ 1884 turbine enabled extraction, scaled up by the 1930s for industrial power and steam, surpassing steam engines (5-10% efficiency) with 20-30%. WWII used them in flexible plants, while post-war automation (1950s-60s) added control precision. The 1970s energy crisis drove CHP adoption, with modern designs using digital systems and alloys for efficiency.
Modern relevance ties to industrial versatility. Refineries and chemical plants save $2-5 million yearly—a 20 MW turbine meets variable steam and power needs cost-effectively. Biomass and waste heat use grows—a 10 MW unit burns 2 tons/hour of wood or uses 400°C exhaust, cutting emissions. Power plants with CHP benefit—a 50 MW unit powers grids and supplies steam. Automation optimizes performance—PLCs adjust extraction instantly—while coatings handle 600°C, though pure condensing turbines lead in grid-scale power.
Advantages include flexibility—automatic extraction adjusts steam from 15 bar to 5 bar on demand. High electrical efficiency (30-40%) beats non-condensing (20-35%), with CHP at 70-80%. Large capacity (up to 100 MW) suits big loads. Fuel flexibility spans coal, gas, biomass, and waste heat. Challenges are complexity—condensers and controls raise costs to $1,500-$2,000/kW vs. $500-$1,000/kW for non-condensing, with $50,000-$200,000 yearly maintenance. Water use (1-2 liters/kWh) strains dry areas. Heat rejection (20-25%) limits thermal reuse vs. non-condensing systems.
Examples show their impact. A 20 MW refinery turbine extracts 20-bar and 5-bar steam, generates 15 MW, saving $3 million yearly. A 5 MW chemical unit extracts 10-bar steam, produces 4 MW, costing $1 million but saving $500,000 annually. A 50 MW power plant turbine extracts 15-bar steam, generates 40 MW, powering 20,000 homes.
Automatic extraction condensing turbines excel where power and steam demands intersect, their adaptability and efficiency making them a powerhouse in industrial and CHP applications.
Here’s a continued exploration of automatic extraction condensing steam turbines, presented as a detailed plain text that builds on the previous discussion with deeper insights and expanded perspectives. Automatic extraction condensing steam turbines are advanced systems that integrate the ability to extract steam at one or more intermediate pressures for industrial or heating purposes with the capability to exhaust the remaining steam into a vacuum, typically 0.05 to 0.1 bar, to maximize electrical power generation. These turbines are designed to deliver mechanical power—most often driving a generator—while providing controlled steam output, with sophisticated automatic control systems dynamically adjusting extraction flows to meet fluctuating process or power demands. They are widely deployed in large industrial complexes such as refineries, chemical plants, and power stations with combined heat and power (CHP) requirements, offering capacities from a few megawatts to hundreds of megawatts. Their hybrid design balances high electrical efficiency with flexible steam delivery, making them a versatile choice for complex energy needs. Let’s delve further into their mechanics, design intricacies, applications, operational specifics, historical evolution, modern significance, advantages, and challenges.
The mechanics of an automatic extraction condensing steam turbine are grounded in the Rankine cycle, optimized to extract maximum energy from steam while accommodating intermediate extraction points. A boiler generates steam by combusting fuels like coal, natural gas, oil, or biomass (e.g., wood pellets, rice husks, or sugarcane bagasse), or harnessing heat from nuclear fission, waste heat recovery (e.g., 400-600°C exhaust from kilns or engines), or concentrated solar power, producing steam at pressures of 50-300 bar and temperatures of 400-600°C, with supercritical or ultra-supercritical units reaching 700°C. This high-pressure steam enters the turbine, flowing over blades mounted on a rotor—a forged steel shaft that can weigh from a few kilograms in smaller units to 10-100 tons in large-scale systems. As the steam expands—say, from 100 bar to an extraction point at 15 bar, then to another at 5 bar, and finally to 0.05 bar—it accelerates to speeds of 500-700 meters per second, driving the blades and spinning the rotor at speeds such as 3,000 RPM for 50 Hz electrical grids, 3,600 RPM for 60 Hz, or up to 10,000 RPM in smaller, high-speed designs. The rotor powers a generator to produce electricity or drives machinery like pumps or compressors through a shaft, often with a gearbox to adjust speed—e.g., stepping down 10,000 RPM to 1,500 RPM for industrial equipment. Steam is extracted at intermediate stages—e.g., 15 bar for a chemical reactor, 5 bar for preheating boiler feedwater—via ports equipped with automatic valves, while the remaining steam exhausts into a condenser, where it cools back to water at 30-50°C under vacuum conditions using cooling water from rivers, oceans, or towers, or air in arid regions. This vacuum maximizes the pressure differential and power output, while the automation system—typically a programmable logic controller (PLC) or distributed control system (DCS)—uses sensors to monitor and adjust extraction flows in real time, ensuring a stable balance between power generation and steam supply as demands shift.
The design of automatic extraction condensing steam turbines is intricate, combining multi-stage expansion, extraction capabilities, and condensing technology. They feature 10-50 stages, organized into high-pressure (HP) sections before the first extraction, intermediate sections for each extraction point, and low-pressure (LP) sections leading to the condenser. HP stages manage the initial steam conditions—e.g., 100 bar, 600°C—with small blades (5-20 cm) crafted from nickel-based superalloys like Inconel 718 or Nimonic, designed to withstand extreme heat and pressure, often coated with chromium carbide or ceramic layers to resist creep, corrosion, and oxidation. Extraction stages handle mid-range pressures—e.g., 15 bar or 5 bar—with blades (10-30 cm) sized for controlled flow, while LP stages process the final expansion to 0.05 bar with massive blades (up to 1.5-2 meters), made of titanium or stainless steel to endure wet steam erosion at tip speeds of 500-700 m/s. Impulse designs are prevalent in HP stages—steam accelerates through nozzles into jets that strike bucket-shaped blades—while reaction designs dominate LP stages, where steam expands across moving blades, with combination turbines blending both approaches for optimal efficiency across the pressure gradient. Extraction ports, equipped with throttling valves or adjustable nozzles, divert steam to external piping, controlled by servo-motors linked to the automation system, which responds to real-time signals from pressure, temperature, or flow sensors. Rotors, weighing tons in large units, are precision-balanced to minimize vibration, while casings—forged from high-strength steel or alloys—contain pressures up to 300 bar, with labyrinth seals or brush seals reducing leakage across stages, a critical feature given the steep drop to vacuum. Condensers—typically shell-and-tube or surface designs—use 1-2 liters of cooling water per kWh or air fans to maintain the vacuum, rejecting 50-60% of heat as waste, supported by cooling towers that
Back Pressure Steam Turbine
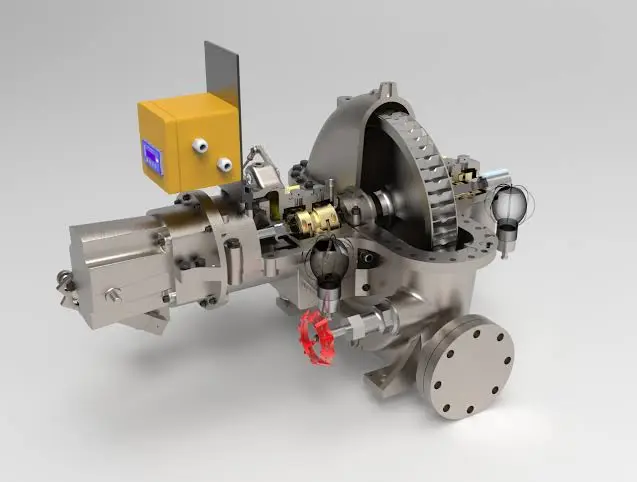
Here’s a detailed exploration of back-pressure steam turbines, presented as a long plain text. Back-pressure steam turbines, also known as non-condensing steam turbines, are a type of steam turbine where the exhaust steam exits at a pressure higher than atmospheric, typically between 2 and 20 bar, rather than being condensed into a vacuum as in condensing turbines. These turbines are designed to produce mechanical power—usually to drive a generator for electricity or to power machinery—while simultaneously supplying usable exhaust steam for industrial processes, heating, or other thermal applications. They are widely utilized in industrial settings such as pulp and paper mills, chemical plants, sugar mills, and combined heat and power (CHP) systems, with power outputs ranging from a few kilowatts to tens of megawatts. Back-pressure steam turbines are valued for their simplicity, high total energy efficiency in CHP applications, and ability to make dual use of steam as both a power source and a heat source. Let’s dive into their mechanics, design features, applications, operational details, historical context, modern relevance, advantages, and challenges.
The mechanics of a back-pressure steam turbine are based on the Rankine cycle, adapted to prioritize both mechanical power and usable exhaust steam over maximizing electrical efficiency alone. A boiler generates steam by burning fuels such as coal, natural gas, oil, or biomass (e.g., wood chips, bagasse, or rice husks), or by capturing waste heat from industrial processes—imagine exhaust from a furnace at 400-600°C or a gas engine at 300-400°C. Steam enters the turbine at pressures typically ranging from 10 to 100 bar and temperatures from 200 to 540°C, depending on the system’s scale and fuel source. This steam flows over blades mounted on a rotor—a forged steel shaft that can weigh from a few kilograms in small units to several tons in larger ones. As the steam expands—dropping from, say, 50 bar to an exhaust pressure of 5 bar—it accelerates to speeds of 300-500 meters per second, pushing the blades and spinning the rotor at speeds like 3,000 RPM for 50 Hz electrical grids, 3,600 RPM for 60 Hz, or up to 10,000 RPM in high-speed industrial designs. The rotor drives a generator to produce electricity or powers mechanical equipment such as pumps, compressors, fans, or mills through a shaft, often with a gearbox to adjust speed—for example, stepping down 10,000 RPM to 1,500 RPM for a pump. Unlike condensing turbines, which exhaust into a vacuum (0.05-0.1 bar) to extract maximum energy, back-pressure turbines release steam at a higher pressure—2 to 20 bar—determined by downstream thermal needs, such as heating a building, drying materials in a factory, or supplying steam for chemical reactions. This exhaust steam retains significant thermal energy, often 50-70% of the input, making back-pressure turbines ideal for CHP systems where the goal is to maximize total energy utilization rather than electrical output alone.
The design of back-pressure steam turbines reflects their dual-purpose role and simpler construction compared to condensing systems. They can be single-stage for small applications—say, a 50 kW unit with one set of blades extracting energy in a single pass—or multi-stage with 5 to 20 stages for larger outputs like 10 MW, allowing progressive energy extraction across multiple blade rows. Impulse designs are common, especially in smaller units—steam accelerates through nozzles into high-velocity jets that strike curved, bucket-shaped blades, spinning the rotor at high speeds like 10,000-20,000 RPM, a design rooted in Gustaf de Laval’s 1880s impulse turbine. Reaction designs, drawing from Charles Parsons’ multi-stage concept, are more typical in larger back-pressure turbines—steam expands across alternating fixed stator and moving rotor blades, spinning at 3,000-6,000 RPM for better efficiency and compatibility with standard generators. Combination designs may integrate impulse and reaction stages—impulse for the high-pressure entry (e.g., 50 bar) and reaction for the lower-pressure exhaust (e.g., 10 bar)—optimizing energy extraction in mid-sized systems. Blades vary in size—5-20 cm in high-pressure sections, up to 50 cm in low-pressure stages—made of stainless steel for durability or nickel-based alloys like Inconel for heat resistance up to 540°C, often coated with chromium or ceramic to resist corrosion and erosion from wet steam. Rotors, forged steel, are precision-balanced to handle high speeds without vibration, while casings—cast steel or alloys—contain pressures up to 100 bar, with seals like labyrinths or packing glands minimizing leakage, though sealing is less critical here than in condensing turbines since the exhaust pressure is higher than atmospheric, reducing the pressure differential that drives leaks.
Back-pressure steam turbine generators couple the turbine with an alternator or dynamo to produce electricity, scaled to the system’s needs. A 1 MW unit might generate 400 V AC for industrial machinery, while a 50 kW unit produces 230 V AC for a small facility, synchronized at 50 or 60 Hz for grid or local use. Generators are typically synchronous—rotor poles spin within stator windings—cooled by air or water to dissipate heat from 50-70% energy losses, with voltage adjustable via regulators to match loads. Boilers, producing 10 to 100 tons/hour of steam, integrate with diverse fuels—natural gas offers clean combustion with minimal emissions, biomass leverages local waste like sawdust or agricultural residues, and waste heat taps free energy from processes like steelmaking or cement production. Unlike condensing turbines, back-pressure systems eliminate the need for a condenser and associated cooling infrastructure—exhaust steam is piped directly to heat exchangers, process equipment, or heating systems, simplifying installation and maintenance compared to the complex cooling towers and vacuum pumps of condensing setups. Control systems range from manual valves and mechanical governors in basic configurations to digital controls with sensors in modern units, adjusting steam flow to balance power and heat output, with costs ranging from $10,000 for a 50 kW unit to $1-2 million for a 10 MW system, depending on size and sophistication.
Applications of back-pressure steam turbines are concentrated in industries and settings where both electrical power and thermal energy are required, making them a natural fit for CHP operations. Pulp and paper mills are a prime example—a 5 MW turbine burns wood waste from sawmill operations, producing 4 MW of electricity to run machinery like grinders and pumps, and 10 tons/hour of 5-bar steam to dry paper pulp, capitalizing on abundant biomass to offset grid costs by $1-2 million annually. Chemical plants use them for process steam—a 10 MW turbine generates 8 MW of power and 20 tons/hour of 15-bar steam for reactors, distillation columns, or heating vats, often fueled by natural gas for its consistent heat output. Sugar mills rely heavily on them with bagasse—a 3 MW turbine powers milling equipment and delivers 8 tons/hour of 10-bar steam to evaporate cane juice, using cane waste to achieve near-self-sufficiency during harvest season, reducing reliance on external energy sources. Textile factories employ smaller units—a 500 kW turbine produces 400 kW and 2 tons/hour of 3-bar steam for dyeing fabrics or heating dye vats, often coal-fired in regions where coal is cheap and accessible. District heating systems in colder climates adopt them—a 2 MW turbine generates 1.5 MW of electricity and heats a small town with 5-bar steam piped to radiators, burning local biomass like wood pellets for sustainability and cost savings. Small cogeneration plants use them for grid support—a 20 MW turbine supplies 15 MW to the grid and steam to nearby factories, fueled by oil or gas in urban settings where fuel supply is reliable. Off-grid applications benefit as well—a 50 kW turbine in a remote farm burns wood, powering tools and heating livestock barns with 2-bar steam, providing energy independence where extending the grid would cost tens of thousands of dollars per kilometer.
Efficiency in back-pressure steam turbines depends on their operational priorities, with a focus on total energy utilization rather than electrical output alone. Electrical efficiency is typically lower than that of condensing turbines—ranging from 20-30%—because the steam expansion stops at a higher exhaust pressure, leaving much of its energy as usable heat rather than converting it fully to mechanical work. A 5 MW turbine burning 20 tons/hour of steam (50 bar, 540°C) might produce 1.5 MW of electricity (25% efficiency), with 70% of the energy exiting as 10-bar steam (3.5 MW thermal equivalent), and 5-10% lost to friction, blade turbulence, and leakage. In CHP mode, total efficiency—combining power and heat—reaches 70-85%, far surpassing the 35-45% electrical efficiency of condensing systems, making them highly efficient when both outputs are utilized. For instance, a 3 MW turbine might convert 25% to electricity (750 kW), 55% to 5-bar steam (1.65 MW thermal), and lose 20% to waste heat—a stark contrast to condensing turbines, which reject 50-60% of energy as unusable heat. Losses in back-pressure turbines come from incomplete expansion (exhaust at 5-20 bar vs. 0.05 bar in condensing units), blade inefficiencies due to fewer stages compared to large turbines, and leakage (5-10% with basic seals). Multi-stage designs with 5-20 stages or superheating steam to 540°C can boost electrical efficiency to 30-35%, but lowering the exhaust pressure (e.g., to 2 bar) increases power output at the expense of usable heat, a trade-off that CHP systems carefully manage to meet thermal demands. Fuel quality significantly impacts performance—dry biomass at 4.5 kWh/kg outperforms wet biomass at 3 kWh/kg, with moisture levels above 20% reducing steam output by 30% if not pre-dried, a common challenge in biomass-heavy applications like sugar mills or sawmills.
The historical development of back-pressure steam turbines traces back to the early days of steam power, evolving from the need to utilize steam efficiently in industrial settings. Charles Parsons’ 1884 multi-stage turbine and Gustaf de Laval’s impulse design provided the foundational technology, but by the early 20th century, industries adopted back-pressure turbines for their ability to supply both power and heat, outpacing less efficient steam engines (5-10% efficiency) with turbines offering 15-25% electrical efficiency plus usable steam. Pre-World War II factories used coal-fired units to drive machinery and heat workspaces, scaling to megawatts by the 1930s as metallurgy improved—chromium steels allowed higher temperatures and pressures, increasing durability and performance. During WWII, back-pressure turbines powered small plants or military facilities—e.g., a 1 MW unit ran a factory and warmed it using rationed fuel, proving their resilience in resource-scarce conditions. Post-war, condensing turbines took over large-scale power generation due to their higher electrical efficiency, but the 1970s energy crisis revived back-pressure designs for CHP applications, particularly with the rise of biomass and waste heat as alternative fuels amid soaring oil prices. Modern units enhance this legacy—digital automation optimizes steam use, and advanced materials like nickel alloys withstand temperatures up to 540°C, though they remain a specialized tool compared to the widespread use of condensing systems in utility-scale power plants.
The modern significance of back-pressure steam turbines lies in their role in energy efficiency and sustainability, particularly in industrial and decentralized energy systems. Industrial CHP applications thrive with these turbines—a 10 MW turbine saves a factory $2-3 million annually by reusing exhaust steam for processes like drying or heating, far more cost-effective than separate power generation (30-40% efficiency) and heating systems (50-60% fuel loss), which can double operational costs. Biomass utilization is on the rise—a 5 MW turbine burns 2 tons/hour of wood waste, reducing fossil fuel dependence in rural industries like sawmills or paper plants, aligning with carbon reduction goals such as those set by international agreements like the Paris Accord. Waste heat recovery expands their reach—a 2 MW turbine uses 400°C exhaust from a steel furnace, generating power and steam without additional fuel, cutting emissions and costs by $500,000 yearly in industries with high heat output. District heating systems in Europe and North America rely on them—a 3 MW turbine heats 500 homes and powers local loads with 5-bar steam, often fueled by gas or biomass for reliability and sustainability, providing a cost-effective alternative to separate electric and heating grids. Decentralized power applications grow too—a 50-500 kW turbine serves off-grid communities or farms where grid access costs exceed $10,000 per kilometer, offering a self-sufficient energy solution. Innovations bolster their role—digital controls with real-time sensors balance power and heat output, advanced coatings handle higher temperatures, and modular designs reduce installation costs, though condensing turbines dominate in scenarios focused solely on large-scale electricity production.
The advantages of back-pressure steam turbines are numerous and context-specific, making them a compelling choice for certain applications. Their simplicity—no need for a condenser or cooling towers—reduces initial costs to $500-$1,000 per kW, compared to $1,500-$2,000/kW for condensing systems, and eliminates the maintenance burden of complex cooling infrastructure like vacuum pumps or cooling water treatment systems. High CHP efficiency (70-85%) maximizes energy utilization—a 5 MW turbine delivers 4 MW of combined electrical and thermal output, outperforming standalone generators or boilers in total energy efficiency, especially when exhaust steam is fully utilized. Fuel flexibility accommodates a wide range of local resources—wood, agricultural waste, gas, or waste heat work effectively, making them viable in diverse settings like forested regions, agricultural zones, or industrial hubs with excess heat. Their compact size fits constrained spaces—a 1 MW unit requires a 5×10-meter footprint versus 10×20 meters for a condensing setup, easing installation in factories or small plants where space is at a premium. Reliability is robust—units can run for 20-30 years with regular maintenance, operating 5,000-8,000 hours/year in harsh conditions like dusty mills or humid climates, with fewer components prone to failure compared to condensing systems. Sustainability aligns with green initiatives—biomass-driven units achieve near carbon neutrality when paired with regrowth cycles, and waste heat recovery minimizes fuel use, supporting eco-friendly operations in industries aiming to reduce their carbon footprint.
Challenges, however, limit their broader adoption and must be carefully considered. Their lower electrical efficiency (20-30%) restricts power output compared to condensing turbines—a 5 MW turbine yields 1.5 MW of electricity versus 2 MW from a condensing turbine with the same steam input, requiring more fuel (e.g., 2 tons/hour of wood versus 1.5 tons) to achieve equivalent electrical generation, which can increase operational costs if heat isn’t fully utilized. The trade-off between exhaust pressure and power constrains design flexibility—higher exhaust pressures (10-20 bar) preserve more heat for thermal use but reduce electrical yield, while lower pressures (2-5 bar) boost power at the cost of thermal utility, requiring precise calibration to meet specific site needs. Maintenance needs—blade wear from wet steam, seal leakage, and boiler scaling—cost $10,000-$50,000 annually for a 5 MW unit, with downtime interrupting power or heat supply, a significant concern in continuous operations like chemical plants or paper mills. Emissions from biomass fuels—particulates at 50-200 mg/m³ and CO—require filters or scrubbers costing $10,000-$50,000 to meet stringent regulations like the U.S. EPA’s 25 mg/m³ limit or the EU’s 20 mg/m³ standard, adding expense and complexity to biomass-driven systems. Initial costs, while lower than condensing systems, remain significant—$500,000 for a 1 MW unit can’t compete with solar’s $1-2/W for small-scale power generation, making them less attractive for pure electricity needs. Fuel logistics pose additional risks—wet biomass (e.g., 50% moisture) or inconsistent waste heat reduces steam quality, cutting output by 20-30% if not managed with drying systems or heat buffers, a practical challenge in regions with variable fuel availability.
Examples showcase their practical impact across various scales and industries. A 1 MW back-pressure turbine in a textile plant burns coal, producing 800 kW of electricity and 3 tons/hour of 5-bar steam for dyeing processes, saving $200,000 annually on energy bills with a $400,000 setup cost, paying back in two years. A 10 MW turbine in a paper mill uses wood waste, generating 8 MW of power and 20 tons/hour of 10-bar steam for drying pulp, reducing operational costs by $2 million yearly against a $2 million investment, leveraging free biomass from mill operations. A 50 kW off-grid turbine in a rural farm burns logs, powering tools with 40 kW and heating a barn with 2-bar steam, costing $10,000 to install but saving $5,000 annually over diesel generators, ideal where fuelwood is abundant and grid access is impractical.
Back-pressure steam turbines excel in environments where power and heat are both valuable, offering a streamlined, efficient solution for industries, small plants, and off-grid sites. Their evolution—from early 20th-century industrial applications to modern CHP systems—reflects a focus on resource efficiency and adaptability, ensuring they remain a relevant and practical choice in a world increasingly balancing energy needs with sustainability goals. Their ability to turn steam into a dual-purpose resource keeps them a vital tool in the energy landscape, particularly where local fuels or waste heat can be harnessed effectively.
Here’s a continued exploration of back-pressure steam turbines, presented as a detailed plain text that builds on the previous discussion with deeper insights and expanded perspectives. Back-pressure steam turbines, also referred to as non-condensing steam turbines, are engineered to generate mechanical power—typically for electricity production or driving machinery—while exhausting steam at a pressure above atmospheric levels, usually between 2 and 20 bar, making the exhaust steam usable for industrial processes, heating, or other thermal applications. Unlike condensing turbines, which exhaust into a vacuum to maximize electrical output, back-pressure turbines prioritize a balance between power generation and thermal energy delivery, making them ideal for combined heat and power (CHP) systems and industrial settings like sugar mills, pulp and paper plants, and chemical facilities. With capacities ranging from a few kilowatts to tens of megawatts, they offer simplicity, high total energy efficiency when paired with heat use, and versatility with various fuel sources. Let’s delve further into their mechanics, design intricacies, applications, operational specifics, historical evolution, modern significance, advantages, and challenges.
The mechanics of a back-pressure steam turbine follow the Rankine cycle, tailored to provide both mechanical work and usable exhaust steam rather than focusing solely on electrical efficiency. A boiler produces steam by combusting fuels such as coal, natural gas, oil, or biomass (e.g., wood pellets, sawdust, or agricultural residues like corn stalks), or by capturing waste heat from sources like industrial exhausts at 400-600°C or engine tailpipes at 300-400°C. Steam enters the turbine at pressures typically between 10 and 100 bar and temperatures from 200 to 540°C, depending on the system’s size and fuel quality. This steam flows over blades mounted on a rotor—a forged steel shaft that ranges from a few kilograms in small units to several tons in larger ones. As the steam expands—say, from 50 bar to an exhaust pressure of 5 bar—it accelerates to speeds of 300-500 meters per second, driving the blades and spinning the rotor at speeds like 3,000 RPM for 50 Hz grids, 3,600 RPM for 60 Hz, or up to 10,000 RPM in high-speed configurations. The rotor powers a generator to produce electricity or drives machinery such as pumps, fans, compressors, or mills via a shaft, often with a gearbox to adjust speed—for example, reducing 10,000 RPM to 1,500 RPM for a pump. Unlike condensing turbines, which exhaust into a vacuum (0.05-0.1 bar) to extract maximum energy, back-pressure turbines release steam at a higher pressure—2 to 20 bar—set by downstream thermal requirements, such as heating a factory, drying lumber, or supplying steam for chemical distillation. This exhaust steam retains substantial thermal energy, often 50-70% of the input, making back-pressure turbines highly effective in CHP setups where the total energy utilization of both power and heat is the primary objective.
The design of back-pressure steam turbines is practical, emphasizing dual-purpose energy production and reduced complexity compared to condensing systems. They can be single-stage for small-scale use—such as a 50 kW unit with one blade set extracting energy in a single pass—or multi-stage with 5 to 20 stages for larger capacities like 10 MW, allowing gradual energy extraction across multiple blade rows. Impulse designs are prevalent in smaller units—steam accelerates through nozzles into high-velocity jets that strike curved, bucket-shaped blades, spinning the rotor at high speeds like 10,000-20,000 RPM, echoing Gustaf de Laval’s 1880s impulse turbine. Reaction designs, inspired by Charles Parsons’ multi-stage approach, are more common in larger back-pressure turbines—steam expands across alternating fixed stator and moving rotor blades, spinning at 3,000-6,000 RPM for improved efficiency and compatibility with standard generators. Combination designs may blend impulse and reaction stages—impulse for the high-pressure entry (e.g., 50 bar) and reaction for the lower-pressure exhaust (e.g., 10 bar)—optimizing performance in mid-sized systems. Blades are sized for the pressure range—5-20 cm in high-pressure sections, up to 50 cm in low-pressure stages—crafted from stainless steel for cost-effectiveness and corrosion resistance or nickel-based alloys like Inconel for heat tolerance up to 540°C, often coated with chromium or ceramic to combat erosion from wet steam. Rotors, forged steel, are precision-balanced to handle high speeds without vibration, while casings—cast steel or alloys—contain pressures up to 100 bar, with seals like labyrinths or packing glands minimizing leakage, though sealing is less critical here than in condensing turbines due to the higher exhaust pressure reducing the pressure differential that drives leaks.
Back-pressure steam turbine generators pair the turbine with a synchronous generator or alternator to produce electricity, tailored to the system’s scale. A 1 MW unit might generate 400 V AC for industrial machinery, while a 50 kW unit produces 230 V AC for smaller loads, synchronized at 50 or 60 Hz for grid or local use. Generators feature rotor poles spinning within stator windings, cooled by air in smaller units or water in larger ones to dissipate heat from 50-70% energy losses, with voltage regulators adjusting output to match demand. Boilers, producing 10 to 100 tons/hour of steam, integrate with a variety of fuels—natural gas provides clean, efficient combustion, biomass utilizes local waste like wood chips or straw, and waste heat taps free energy from processes such as glass manufacturing or cement kilns. Unlike condensing turbines, back-pressure systems skip the condenser and cooling towers—exhaust steam is piped directly to heat exchangers, process equipment, or heating systems, simplifying the setup and reducing the footprint and maintenance compared to the extensive cooling infrastructure of condensing turbines. Control systems vary—manual valves and mechanical governors suffice in basic setups, while modern units use digital controls with sensors to adjust steam flow, optimizing the balance between power and heat output, with costs ranging from $10,000 for a 50 kW unit to $1-2 million for a 10 MW system, depending on capacity and features.
Applications of back-pressure steam turbines are centered in industries and settings where both electrical power and thermal energy are in demand, making them a cornerstone of CHP operations. Pulp and paper mills frequently use them—a 5 MW turbine burns wood waste from sawmill operations, producing 4 MW of electricity to power grinders and conveyors, and 10 tons/hour of 5-bar steam to dry paper pulp, leveraging abundant biomass to save $1-2 million annually on grid electricity and heating costs. Chemical plants rely on them for process steam—a 10 MW turbine generates 8 MW of power and 20 tons/hour of 15-bar steam for reactors, distillation units, or heating, often fueled by natural gas for its reliability and low emissions. Sugar mills depend on them with bagasse—a 3 MW turbine powers milling equipment and supplies 8 tons/hour of 10-bar steam to evaporate cane juice, using cane waste to achieve near energy self-sufficiency during harvest season, minimizing reliance on external fuel sources. Textile factories use smaller units—a 500 kW turbine produces 400 kW and 2 tons/hour of 3-bar steam for dyeing or heating dye vats, often coal-fired in regions with affordable coal supplies. District heating systems in colder climates adopt them—a 2 MW turbine generates 1.5 MW of electricity and heats a small town with 5-bar steam piped to radiators, burning local biomass like wood pellets for cost-effective sustainability. Small cogeneration plants use them for grid support—a 20 MW turbine supplies 15 MW to the grid and steam to nearby factories, fueled by oil or gas in urban areas with reliable fuel access. Off-grid applications benefit too—a 50 kW turbine in a remote farm burns wood, powering tools and heating barns with 2-bar steam, offering energy independence where grid extension costs are prohibitive.
Efficiency in back-pressure steam turbines hinges on their dual-purpose operation, with a focus on total energy utilization rather than electrical output alone. Electrical efficiency is typically 20-30%, lower than condensing turbines (35-45%) because the steam expansion stops at a higher exhaust pressure, preserving energy for thermal use rather than converting it fully to mechanical work. A 5 MW turbine burning 20 tons/hour of steam (50 bar, 540°C) might produce 1.5 MW of electricity (25% efficiency), with 70% of the energy exiting as 10-bar steam (3.5 MW thermal equivalent), and 5-10% lost to friction, blade turbulence, and leakage. In CHP mode, total efficiency—combining power and heat—reaches 70-85%, significantly outperforming the 35-45% electrical efficiency of condensing systems when exhaust steam is fully utilized. For example, a 3 MW turbine might convert 25% to electricity (750 kW), 55% to 5-bar steam (1.65 MW thermal), and lose 20% to waste heat—a stark contrast to condensing turbines, which discard 50-60% of energy as unusable heat. Losses in back-pressure turbines arise from incomplete expansion (exhaust at 5-20 bar vs. 0.05 bar in condensing units), blade inefficiencies due to fewer stages than large condensing turbines, and leakage (5-10% with basic seals). Multi-stage designs with 5-20 stages or superheating steam to 540°C can increase electrical efficiency to 30-35%, but lowering the exhaust pressure (e.g., to 2 bar) boosts power at the expense of usable heat, a trade-off that CHP systems calibrate to match thermal demands. Fuel quality plays a critical role—dry biomass at 4.5 kWh/kg outperforms wet biomass at 3 kWh/kg, with moisture levels above 20% reducing steam output by 30% if not pre-dried, a frequent challenge in biomass-driven systems like sugar mills or rural installations.
The historical evolution of back-pressure steam turbines traces back to the early 20th century, driven by industrial needs for efficient steam use. Charles Parsons’ 1884 multi-stage turbine and Gustaf de Laval’s impulse design laid the groundwork, but by the 1920s, industries adopted back-pressure turbines for their ability to supply both power and heat, surpassing steam engines (5-10% efficiency) with turbines offering 15-25% electrical efficiency plus usable steam. Pre-World War II factories used coal-fired units to drive machinery and heat workspaces, scaling to megawatts by the 1930s as metallurgy advanced—chromium steels enabled higher temperatures and pressures, improving durability and output. During WWII, back-pressure turbines powered small plants or military facilities—e.g., a 1 MW unit ran a factory and heated it on limited fuel, showcasing their adaptability. Post-war, condensing turbines dominated large-scale power generation due to their higher electrical efficiency, but the 1970s energy crisis revived back-pressure designs for CHP, particularly with biomass and waste heat as oil prices soared, prompting a shift toward energy conservation. Modern units build on this legacy—digital automation enhances steam management, and materials like nickel alloys withstand 540°C, refining their role in industrial and sustainable energy systems, though they remain niche compared to condensing turbines in utility-scale applications.
The modern significance of back-pressure steam turbines lies in their efficiency and adaptability in industrial and decentralized energy contexts. Industrial CHP systems benefit greatly—a 10 MW turbine saves a factory $2-3 million annually by reusing exhaust steam for processes like drying or heating, far more economical than separate power generation (30-40% efficiency) and heating systems (50-60% fuel loss), which can inflate costs by 50-100%. Biomass utilization grows—a 5 MW turbine burns 2 tons/hour of wood waste, reducing fossil fuel reliance in rural industries like sawmills or paper plants, aligning with carbon neutrality goals and policies like the EU’s Green Deal. Waste heat recovery expands their scope—a 2 MW turbine uses 400°C exhaust from a glass furnace, generating power and steam without additional fuel, cutting emissions and costs by $500,000 yearly in heat-intensive sectors. District heating in colder regions leans on them—a 3 MW turbine heats 500 homes and powers local loads with 5-bar steam, often fueled by gas or biomass for reliability and eco-friendliness, offering a cost-effective alternative to separate grids. Decentralized power applications rise too—a 50-500 kW turbine serves off-grid communities or farms where grid extension costs exceed $10,000 per kilometer, providing self-sufficient energy. Innovations enhance their utility—digital controls with real-time sensors optimize power and heat balance, advanced coatings handle higher temperatures, and modular designs cut installation costs, though condensing turbines lead in pure power generation scenarios.
The advantages of back-pressure steam turbines are compelling for their specific applications. Their simplicity—no condenser or cooling towers—lowers initial costs to $500-$1,000 per kW versus $1,500-$2,000/kW for condensing systems, and reduces maintenance by avoiding complex cooling infrastructure like vacuum pumps or water treatment plants. High CHP efficiency (70-85%) maximizes energy use—a 5 MW turbine delivers 4 MW of combined electrical and thermal output, outperforming standalone generators or boilers when exhaust steam is fully utilized. Fuel flexibility accommodates diverse resources—wood, agricultural waste, gas, or waste heat work seamlessly, making them viable in forested areas, farming regions, or industrial zones with excess heat. Their compact size suits tight spaces—a 1 MW unit requires a 5×10-meter footprint versus 10×20 meters for a condensing setup, easing installation in factories or small plants. Reliability is strong—units run 20-30 years with regular maintenance, operating 5,000-8,000 hours/year in tough conditions like dusty mills or humid climates, with fewer failure-prone components than condensing systems. Sustainability aligns with green trends—biomass units offset CO2 with regrowth, and waste heat recovery cuts fuel use, appealing to eco-conscious operations.
Challenges limit their broader adoption. Lower electrical efficiency (20-30%) caps power output—a 5 MW turbine yields 1.5 MW versus 2 MW from a condensing turbine with the same input, needing more fuel (e.g., 2 tons/hour of wood vs. 1.5 tons) for equivalent electricity, raising costs if heat isn’t fully used. Exhaust pressure trade-offs constrain design—higher pressures (10-20 bar) preserve heat but reduce power, while lower pressures (2-5 bar) boost power at heat’s expense, requiring precise tuning. Maintenance—blade wear, seal leaks, boiler scaling—costs $10,000-$50,000 yearly for a 5 MW unit, with downtime disrupting output, a concern in continuous processes. Biomass emissions (50-200 mg/m³ particulates) need filters ($10,000-$50,000) to meet limits like the EU’s 20 mg/m³, adding cost. Initial costs—$500,000 for 1 MW—lag solar ($1-2/W) for small-scale power. Fuel inconsistency—wet biomass or variable heat—cuts output by 20-30% without mitigation.
Examples highlight their value. A 1 MW textile turbine burns coal, produces 800 kW and 3 tons/hour of 5-bar steam, saving $200,000 yearly. A 10 MW paper mill turbine uses wood waste, generates 8 MW and 20 tons/hour of 10-bar steam, saving $2 million. A 50 kW farm turbine burns logs, powers 40 kW and heats with 2-bar steam, saving $5,000 annually.
Back-pressure steam turbines shine where power and heat converge, their efficiency and adaptability making them vital for industries and CHP, blending steam’s legacy with modern energy needs.
EMS Power Machines
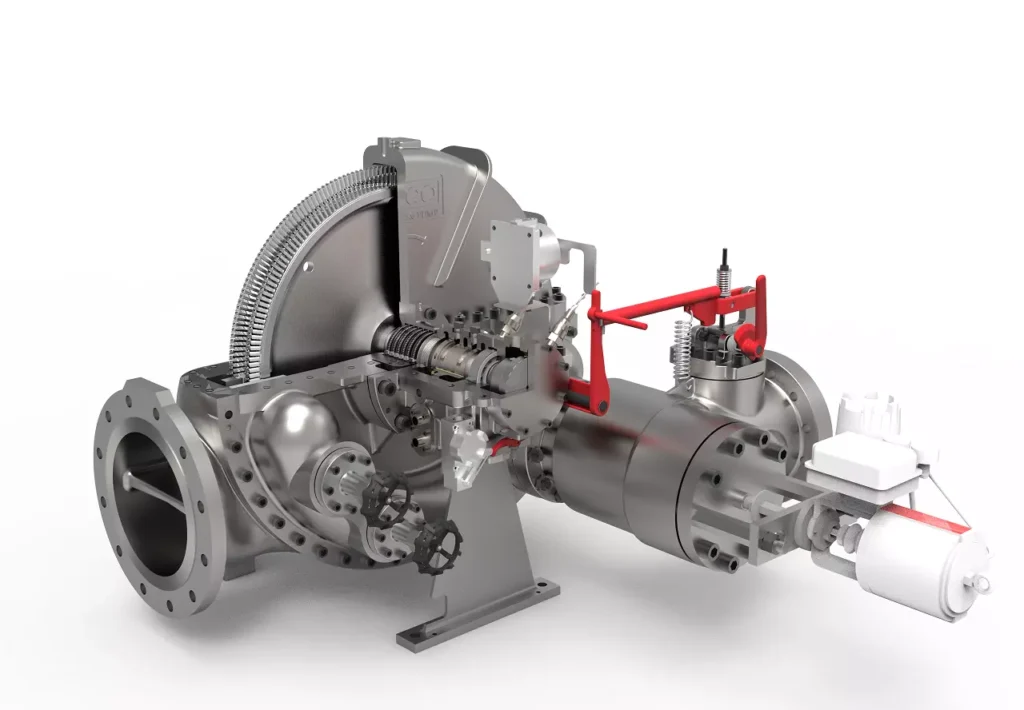
We design, manufacture and assembly Power Machines such as – diesel generators, electric motors, vibration motors, pumps, steam engines and steam turbines
EMS Power Machines is a global power engineering company, one of the five world leaders in the industry in terms of installed equipment. The companies included in the company have been operating in the energy market for more than 60 years.
EMS Power Machines manufactures steam turbines, gas turbines, hydroelectric turbines, generators, and other power equipment for thermal, nuclear, and hydroelectric power plants, as well as for various industries, transport, and marine energy.
EMS Power Machines is a major player in the global power industry, and its equipment is used in power plants all over the world. The company has a strong track record of innovation, and it is constantly developing new and improved technologies.
Here are some examples of Power Machines’ products and services:
- Steam turbines for thermal and nuclear power plants
- Gas turbines for combined cycle power plants and industrial applications
- Hydroelectric turbines for hydroelectric power plants
- Generators for all types of power plants
- Boilers for thermal power plants
- Condensers for thermal power plants
- Reheaters for thermal power plants
- Air preheaters for thermal power plants
- Feedwater pumps for thermal power plants
- Control systems for power plants
- Maintenance and repair services for power plants
EMS Power Machines is committed to providing its customers with high-quality products and services. The company has a strong reputation for reliability and innovation. Power Machines is a leading provider of power equipment and services, and it plays a vital role in the global power industry.
EMS Power Machines, which began in 1961 as a small factory of electric motors, has become a leading global supplier of electronic products for different segments. The search for excellence has resulted in the diversification of the business, adding to the electric motors products which provide from power generation to more efficient means of use.