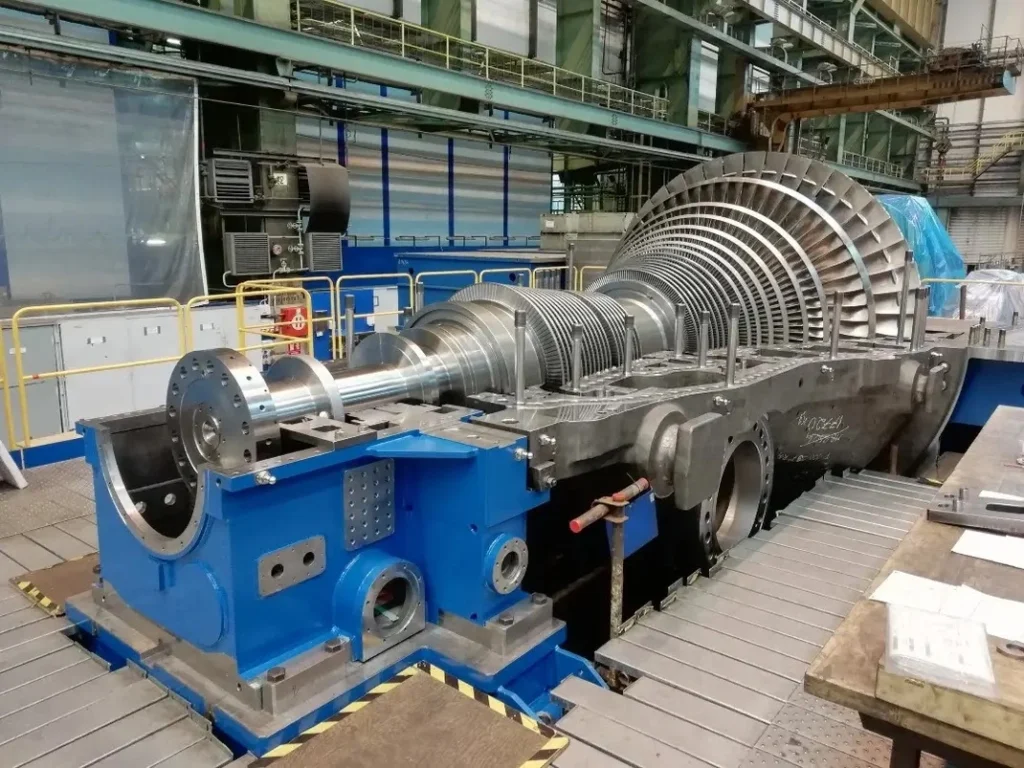
The industrial steam turbine, a testament to the convergence of mechanical engineering and industrial innovation, undergoes a comprehensive manufacturing process to emerge as a vital component in the landscape of power generation within industrial settings. This intricate device stands at the intersection of advanced technology and practical utility, designed to efficiently convert steam energy into mechanical power to drive industrial processes.
At the heart of the industrial steam turbine lies the precision-engineered turbine unit. This critical component boasts intricately designed blades mounted on a rotating shaft, strategically positioned to capture and harness the energy within high-pressure steam. The materials chosen for these components must exhibit exceptional strength and resistance to the extreme conditions of elevated temperatures and pressures prevalent in industrial applications.
Simultaneously, the generator unit undergoes meticulous construction. Engineered to transform the rotational motion of the turbine into electrical power, the generator typically features a configuration of rotor and stator components. The manufacturing process delves into intricate steps such as coil winding, assembly of magnetic elements, and a keen focus on insulation and alignment to ensure optimal electrical efficiency.
The housing or casing, an often-overlooked yet crucial element, is designed with structural robustness to withstand the rigors of industrial operations. Precision welding and advanced machining techniques are employed to meet stringent quality standards, ensuring the durability and longevity of the turbine unit under demanding conditions.
Auxiliary systems play a significant role in enhancing the overall functionality and safety of the industrial steam turbine. Cutting-edge control and monitoring units, equipped with an array of sensors, controllers, and safety mechanisms, regulate critical parameters such as steam pressure, temperature, and turbine speed. The control system’s sophisticated programming facilitates seamless operation under diverse conditions and implements protective measures in response to anomalies, ensuring both operational efficiency and safety.
Cooling systems are integral to the design, adopting methods such as air or water cooling to maintain optimal operating temperatures. These systems prevent the overheating of crucial components, contributing substantially to the overall efficiency and reliability of the industrial steam turbine.
Throughout the assembly phase, each precision-engineered component finds its place within the complex system. Quality assurance procedures, including rigorous testing and inspections, are meticulously executed to confirm the integrity of individual components and the cohesive functioning of the entire system.
Upon completion, the industrial steam turbine undergoes exhaustive testing under a variety of operational conditions. Rigorous simulations of load scenarios, thermal stress tests, and validation of control systems ensure that the turbine meets or exceeds stringent performance standards. The end result is a robust and reliable industrial steam turbine, a technological marvel poised to contribute significantly to the dynamic landscape of industrial power generation, where the conversion of steam energy into mechanical and electrical power stands as a cornerstone of efficient and sustainable operations.
Industrial Steam Turbine
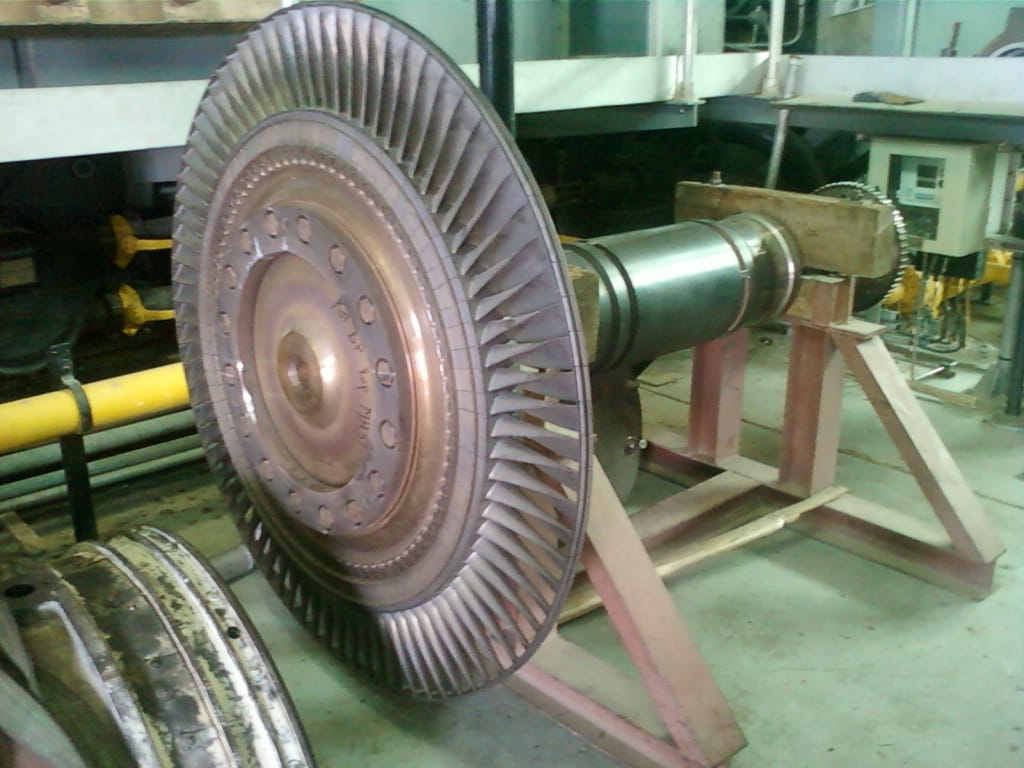
The fabrication of an industrial steam turbine is a nuanced process that seamlessly intertwines engineering precision, materials science, and industrial application. This intricate machinery serves as a linchpin in industrial power generation, designed to adeptly convert steam energy into mechanical power to propel diverse industrial processes.
Central to the industrial steam turbine is the turbine unit, a finely tuned assembly of blades mounted on a rotating shaft. These blades are strategically positioned to capture the potency of high-pressure steam, emphasizing the need for materials that exhibit robustness and resilience under the extreme conditions of elevated temperatures and pressures commonly found in industrial environments.
Simultaneously, the generator unit undergoes meticulous construction, functioning as the mechanism to translate the rotational energy of the turbine into electrical power. This section typically includes rotor and stator components, with manufacturing processes delving into intricacies such as coil winding, meticulous assembly of magnetic elements, and stringent attention to insulation and alignment, all aimed at optimizing electrical efficiency.
The housing or casing, often overlooked but crucial, is engineered for structural resilience to withstand the relentless demands of industrial operations. Precision welding and advanced machining techniques are harnessed to meet exacting quality standards, ensuring the turbine’s durability and sustained functionality in the face of challenging conditions.
Auxiliary systems play a pivotal role in augmenting the overall functionality and safety of the industrial steam turbine. Advanced control and monitoring units, furnished with an array of sensors, controllers, and safety mechanisms, take charge of regulating critical parameters like steam pressure, temperature, and turbine speed. The control system’s nuanced programming ensures seamless operation across diverse conditions, implementing protective measures in real-time to preserve both operational efficiency and safety.
Cooling systems, integrated seamlessly into the design, adopt methodologies such as air or water cooling to uphold optimal operating temperatures. These systems act as guardians against the overheating of crucial components, thereby significantly contributing to the overall efficiency and reliability of the industrial steam turbine.
During the assembly phase, each intricately crafted component finds its place in the mosaic of the larger system. Quality assurance procedures, encompassing rigorous testing and inspections, are meticulously conducted to affirm the integrity of individual components and the harmonious interplay of the entire system.
Upon completion, the industrial steam turbine undergoes exhaustive testing under a spectrum of operational conditions. Rigorous simulations, thermal stress tests, and validation of control systems constitute this phase, ensuring that the turbine aligns seamlessly with, and often exceeds, stringent performance benchmarks. The end result is a resilient and dependable industrial steam turbine—a technological marvel poised to make significant contributions to the dynamic landscape of industrial power generation, where the conversion of steam energy into both mechanical and electrical power stands as an essential driver of efficiency and sustainability.
Industrial Steam Turbine Types
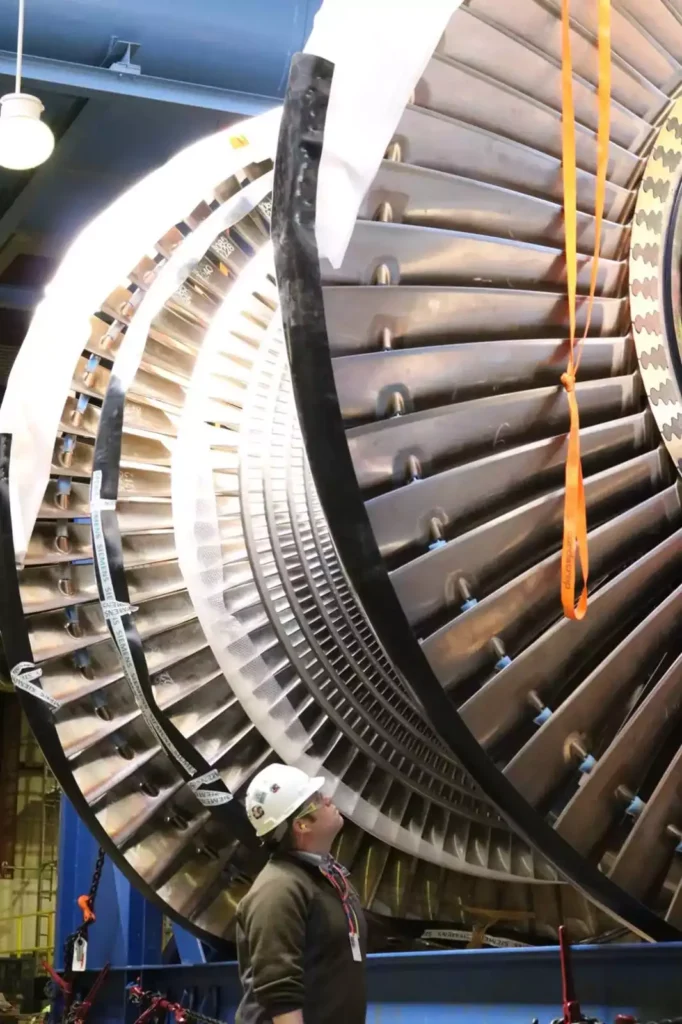
The realm of industrial steam turbines encompasses a diverse array of types, each tailored to meet specific operational requirements and industrial applications. These sophisticated machines, which form the backbone of industrial power generation, demonstrate versatility in their design and functionality.
One prevalent type is the condensing steam turbine, widely employed in power plants and large-scale industrial facilities. This turbine operates in tandem with a condenser to maximize efficiency. The high-pressure steam is expanded in the turbine, producing mechanical work, and is then condensed back into water in the condenser, facilitating the extraction of additional energy and enhancing overall efficiency.
In contrast, back-pressure steam turbines are designed for applications where the steam must be utilized at a specific pressure after passing through the turbine. These turbines find utility in industrial processes where low to moderate pressure steam is required for specific tasks, such as heating or certain manufacturing processes.
The extraction steam turbine is another noteworthy type, featuring multiple extraction points for steam at various pressure levels. This design allows the turbine to cater to diverse industrial needs by supplying steam at different pressures for various processes within a facility.
Condensing-extraction steam turbines combine the features of both condensing and extraction turbines. They efficiently extract steam at multiple points for different applications while also benefiting from the condensation process, enhancing overall energy extraction.
In the realm of industrial steam turbines, a special mention goes to the impulse and reaction turbine types. Impulse turbines utilize the kinetic energy of high-velocity steam jets to produce mechanical work. They often feature moving blades in the form of buckets or nozzles to harness the steam’s kinetic energy.
On the other hand, reaction turbines are characterized by the continuous conversion of pressure energy into mechanical work as steam flows over stationary and rotating blades. These turbines are particularly suitable for applications where a high degree of pressure drop is required.
In the domain of industrial power generation, the steam reheat turbine has gained prominence. This type involves a reheating process where steam, after partial expansion in the high-pressure turbine, is reheated before passing through the low-pressure turbine. This facilitates increased efficiency by preventing excessive moisture content in the steam.
Industrial steam turbines can also be categorized based on their specific applications, such as marine turbines for naval propulsion or mechanical drive turbines for industrial processes beyond power generation. The marine variant, for instance, must contend with dynamic operational conditions, while mechanical drive turbines are tailored to provide mechanical power for various industrial machinery.
In conclusion, the landscape of industrial steam turbines is marked by diversity, with each type meticulously crafted to fulfill distinct operational needs. Whether optimizing efficiency in power plants, providing essential steam for industrial processes, or propelling naval vessels, these turbines exemplify engineering excellence in their capacity to adapt and excel in a myriad of industrial applications.
Impulse Steam Turbines
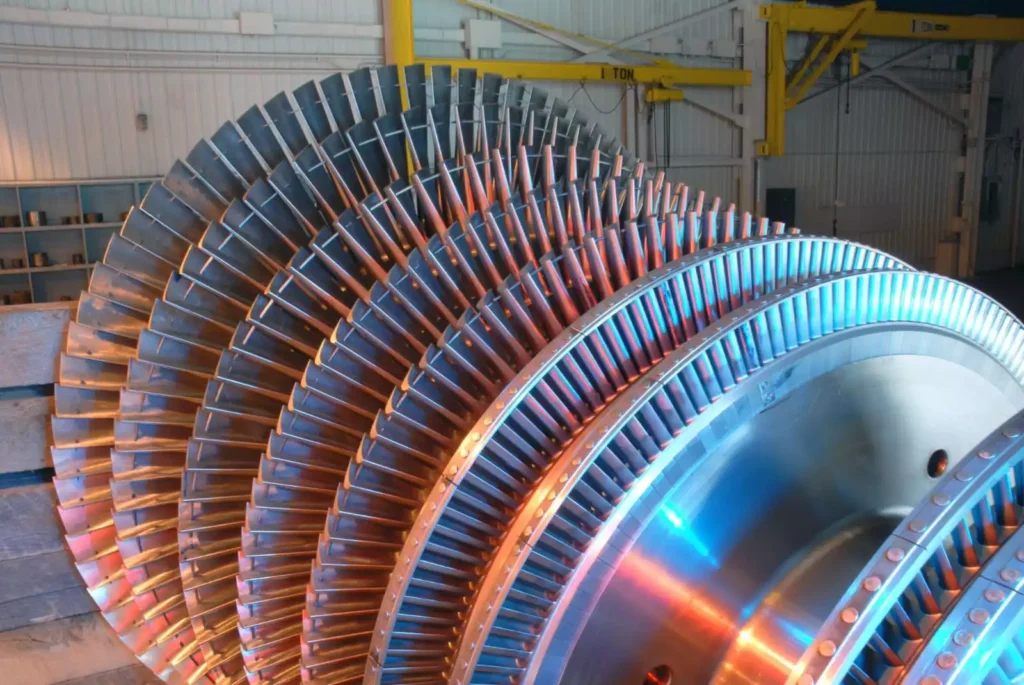
Impulse turbines stand as a distinctive and influential category within the realm of steam turbines, characterized by their unique approach to harnessing the kinetic energy of high-velocity steam to generate mechanical power. These turbines play a vital role in various industrial and power generation applications, embodying a design philosophy that capitalizes on the principles of fluid dynamics.
At the core of impulse turbines is the principle of converting the potential energy of steam into kinetic energy. This is achieved through the expansion of high-pressure steam through a set of nozzles. As the steam exits the nozzles at high velocity, it strikes a series of moving blades, commonly in the form of buckets, with remarkable force. This impact results in a transfer of kinetic energy from the steam to the blades, causing them to move and creating mechanical work.
One of the key distinguishing features of impulse turbines lies in the fact that the pressure of the steam remains constant throughout the expansion process. The kinetic energy imparted to the blades is solely a consequence of the steam’s velocity. Consequently, impulse turbines are particularly well-suited for applications where a single, high-velocity jet of steam can be efficiently utilized.
Within the realm of impulse turbines, there are variations in design, with the Curtis and Rateau turbines being notable examples. The Curtis turbine typically employs multiple stages of alternating fixed and moving blades. This arrangement allows for the gradual extraction of energy from the steam, optimizing efficiency. On the other hand, the Rateau turbine features a single set of rotating blades within a series of fixed nozzles, simplifying the design and making it suitable for specific industrial applications.
Impulse turbines find applications in diverse settings, ranging from hydroelectric power plants to certain configurations of steam-driven power generation. Their adaptability is underscored by their capacity to handle variable load conditions effectively. In hydroelectric power generation, for instance, the high-pressure water jet serves as the driving force, mirroring the steam’s role in steam-driven impulse turbines.
Despite their effectiveness in specific scenarios, impulse turbines are not without limitations. Their reliance on high-velocity steam necessitates the availability of steam at high pressures, which may pose challenges in certain applications. Additionally, the design complexity associated with multiple stages of blades, as seen in Curtis turbines, can impact manufacturing and maintenance considerations.
In essence, impulse turbines epitomize the synergy between fluid dynamics and mechanical engineering, showcasing a design philosophy that has been instrumental in harnessing the kinetic energy of steam for power generation. From the majestic cascades of hydroelectric power plants to industrial settings demanding precise mechanical work, impulse turbines stand as a testament to innovation in the quest for efficient and sustainable energy solutions.
Reaction Steam Turbines
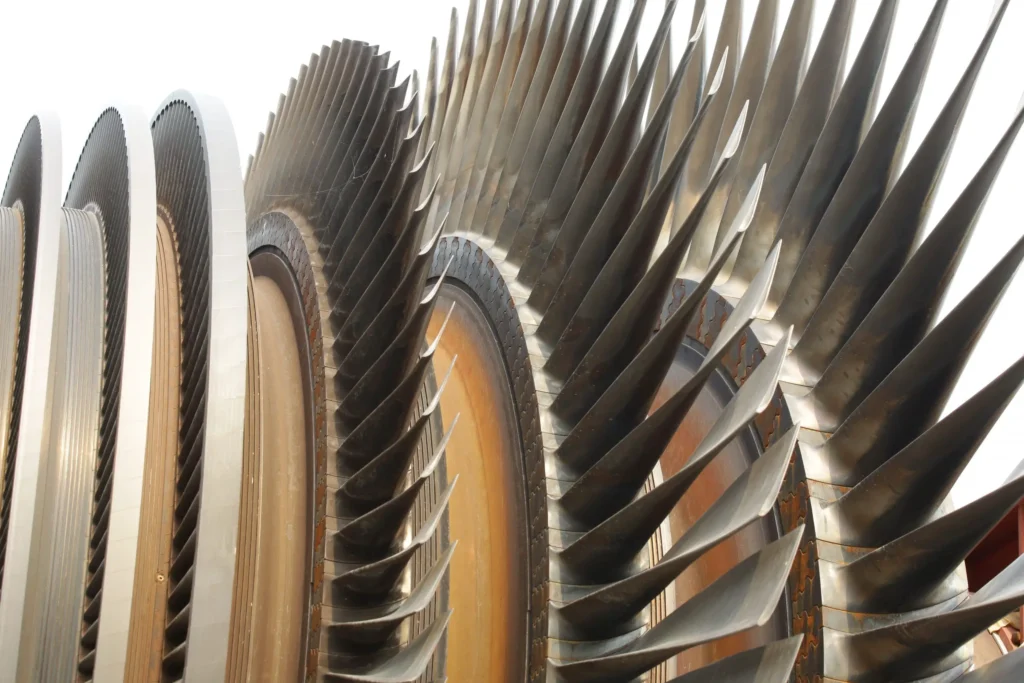
Reaction steam turbines, a cornerstone in the landscape of power generation and industrial applications, represent a sophisticated and efficient means of converting the potential energy of steam into mechanical work. Unlike impulse turbines that harness the kinetic energy of high-velocity steam jets, reaction turbines operate on the principle of transforming both kinetic and pressure energy as steam flows over a series of stationary and rotating blades.
At the heart of a reaction steam turbine lies the intricate interplay between pressure and velocity. Steam enters the turbine at high pressure, and as it flows over the stationary blades, it undergoes an expansion that results in both pressure and velocity changes. The rotating blades, also known as rotor blades, are shaped to allow steam to continuously expand and increase in velocity, leading to a conversion of pressure energy into kinetic energy.
One of the distinguishing features of reaction turbines is the fact that the pressure of the steam decreases progressively as it traverses through the turbine stages. This contrasts with impulse turbines, where the pressure remains constant throughout the expansion process. The gradual pressure drop in reaction turbines enables them to efficiently extract energy from the steam over multiple stages, enhancing overall efficiency.
The design of reaction turbines encompasses both axial and radial configurations. Axial flow turbines feature steam flowing parallel to the axis of rotation, facilitating a streamlined and compact design. In contrast, radial flow turbines direct steam outward from the center of rotation, often in a radial or diagonal direction. The choice between axial and radial configurations depends on factors such as application requirements and engineering considerations.
Reaction steam turbines find widespread application in power plants, where their efficiency and versatility contribute significantly to electricity generation. These turbines are commonly employed in combined-cycle power plants, where they work in conjunction with gas turbines to maximize overall plant efficiency.
In industrial settings, reaction turbines play a pivotal role in driving mechanical equipment, such as compressors and pumps. Their adaptability to varying steam conditions and load fluctuations makes them suitable for applications where a constant and reliable source of mechanical power is essential.
While reaction turbines exhibit notable advantages in terms of efficiency and adaptability, they are not without challenges. The design complexity associated with the intricate interplay of pressure and velocity demands precision engineering. Additionally, the potential for erosion and wear on the blades due to the dynamic nature of steam flow necessitates careful material selection and maintenance practices.
In conclusion, reaction steam turbines stand as exemplars of engineering ingenuity, seamlessly converting the energy within steam into valuable mechanical work. From power generation to industrial applications, their versatility and efficiency make them integral components in the dynamic landscape of energy conversion, contributing to the sustainable and reliable generation of power.
Parts of the Industrial Steam Turbine
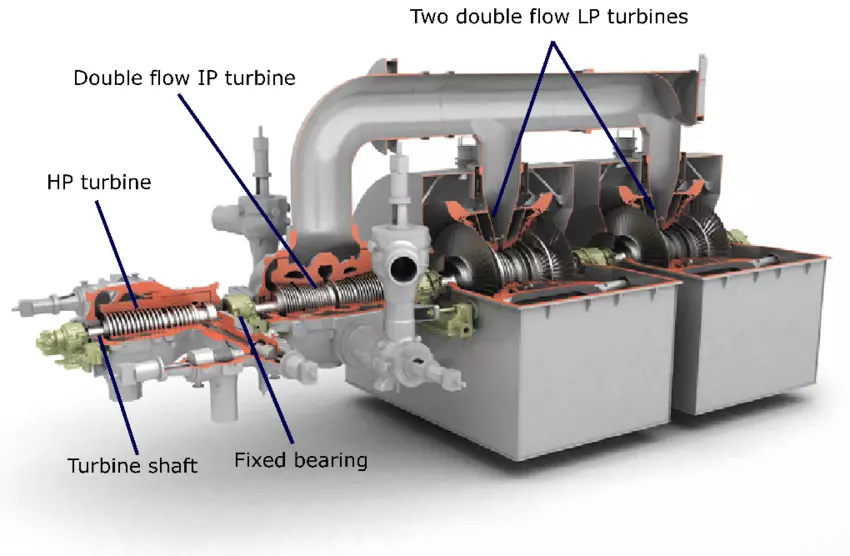
The industrial steam turbine, a complex and robust machine at the heart of power generation in industrial settings, comprises various integral parts that work in concert to convert steam energy into mechanical power. Understanding the key components of an industrial steam turbine illuminates the intricate interplay of engineering precision and thermodynamic principles.
1. Rotor and Blades: The rotor is a central component of the steam turbine, consisting of a shaft with a series of blades attached to it. These blades play a crucial role in extracting energy from the steam as it flows over them. Depending on the type of turbine, the blades may be impulse blades, where steam jets impinge on them to produce kinetic energy, or reaction blades, where steam expands and changes direction, producing both kinetic and pressure energy.
2. Stator: Complementing the rotor, the stator is a stationary component within the steam turbine. It houses the stationary blades, also known as nozzles or guide vanes, which direct the flow of steam onto the moving rotor blades. The stator serves to efficiently guide and control the steam as it passes through the turbine stages.
3. Casing: The casing, or housing, encapsulates the rotor and stator components. It serves multiple functions, providing structural support, containing steam within the turbine, and housing various internal components. The casing is often designed to withstand high temperatures and pressures associated with steam operation.
4. Governor and Control System: The governor is a crucial part of the control system, regulating the speed of the turbine by adjusting the flow of steam. Modern industrial steam turbines feature advanced control systems that include sensors, controllers, and safety mechanisms. These systems ensure precise regulation of parameters such as steam pressure and temperature, optimizing the turbine’s performance under varying conditions.
5. Bearings and Seals: Bearings support the rotating shaft and rotor, allowing smooth and controlled movement. Proper lubrication and cooling mechanisms are essential for bearing longevity. Seals are critical for maintaining the integrity of the casing, preventing steam leakage, and ensuring efficient turbine operation.
6. Steam Inlet and Exhaust: The steam inlet is where high-pressure steam enters the turbine, and its flow is directed over the blades. After passing through the turbine stages, the steam exits through the exhaust, having released its energy. Efficient steam inlet and exhaust systems are vital for maximizing the turbine’s energy conversion efficiency.
7. Condenser (in some applications): In certain industrial steam turbine configurations, a condenser may be present. The condenser facilitates the conversion of steam back into water, extracting additional energy and improving overall efficiency. This is common in power plants and other applications where a higher level of efficiency is desired.
8. Cooling Systems: Industrial steam turbines often incorporate cooling systems to maintain optimal operating temperatures. This may involve air-cooling or water-cooling mechanisms to prevent overheating of critical components, ensuring long-term reliability.
Understanding the intricate details of these components provides insights into the functionality and efficiency of industrial steam turbines. Each part plays a vital role in the overall system, contributing to the turbine’s ability to efficiently convert steam energy into mechanical power for a wide range of industrial applications.
Applications of Industrial Steam Turbines
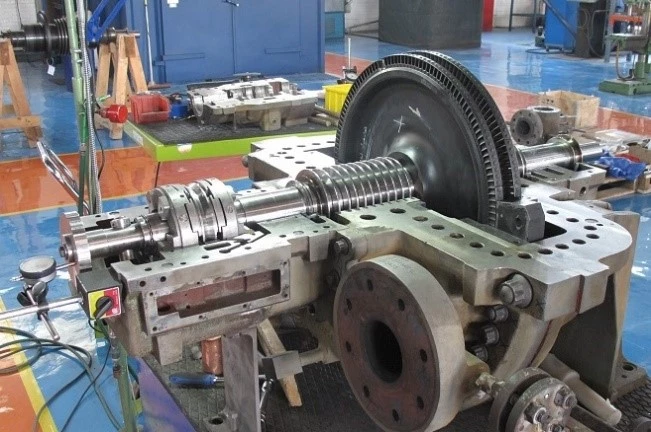
Industrial steam turbines find a broad spectrum of applications across diverse sectors, showcasing their versatility and efficiency in converting steam energy into valuable mechanical and electrical power. These robust machines play a pivotal role in powering industrial processes, enhancing energy efficiency, and contributing to the overall sustainability of various applications.
1. Power Generation: One of the primary applications of industrial steam turbines is in power generation. Steam turbines are integral components of power plants, where they convert the thermal energy of steam into mechanical energy, subsequently driving generators to produce electrical power. Both fossil fuel and nuclear power plants commonly utilize steam turbines to generate electricity on a large scale.
2. Combined Heat and Power (CHP) Plants: Industrial steam turbines are key components in Combined Heat and Power (CHP) plants, also known as cogeneration plants. In these facilities, steam turbines not only generate electricity but also harness the waste heat produced during the process. This heat is utilized for various industrial purposes, such as heating buildings, industrial processes, or providing steam for additional power generation.
3. Petrochemical Industry: The petrochemical industry extensively employs industrial steam turbines in various processes. Steam turbines drive compressors, pumps, and other machinery essential for refining crude oil, producing petrochemicals, and supporting overall plant operations. These turbines contribute to the reliability and efficiency of critical processes within the petrochemical sector.
4. Manufacturing and Industrial Processes: Industrial steam turbines play a vital role in powering machinery and processes in manufacturing industries. They are used for driving equipment such as fans, blowers, compressors, and pumps, contributing to the efficiency of manufacturing operations. Steam turbines are also employed in paper mills, textile factories, and other industrial settings where a continuous and reliable power source is required.
5. District Heating Systems: District heating systems utilize industrial steam turbines to generate both electricity and steam for heating purposes. These systems supply thermal energy to residential and commercial buildings, improving energy efficiency by co-generating electricity and heat from a single source.
6. Marine Propulsion: Steam turbines have been historically employed in marine propulsion systems, driving the propellers of ships and naval vessels. While newer propulsion technologies have emerged, steam turbines continue to be used in certain marine applications due to their reliability and ability to generate high power outputs.
7. Sugar and Bioenergy Industry: In the sugar and bioenergy industry, industrial steam turbines are utilized in sugar mills and bioethanol production plants. They drive machinery for sugar extraction and processing, as well as contribute to the generation of electricity from biomass sources.
8. Renewable Energy: Industrial steam turbines also find applications in renewable energy systems, particularly in geothermal power plants. Steam produced from geothermal sources is used to drive steam turbines, generating clean and sustainable electricity.
9. Mechanical Drive Applications: Steam turbines serve as prime movers for mechanical drive applications in various industries. They power mechanical equipment such as compressors, fans, and pumps, providing essential rotational energy for diverse industrial processes.
In summary, industrial steam turbines are foundational to the operation of numerous industrial sectors, ranging from traditional power generation to emerging applications in renewable energy. Their adaptability, efficiency, and capacity to generate both mechanical and electrical power make them indispensable components across a diverse array of industrial processes, contributing significantly to global energy infrastructure and sustainable development.
Industrial Steam Turbine
1. Engineering and Design:
- Optimizing steam inlet design for efficiency and performance.
- Material selection and challenges in high-pressure, high-temperature environments.
- Advanced control systems and their impact on turbine operation.
- Novel blade designs for improved energy extraction.
2. Applications and Impact:
- The role of steam turbines in specific industries (e.g., power generation, marine propulsion, chemical processing).
- Economic and environmental considerations of using steam turbines compared to other power sources.
- The future of steam turbines: integration with renewable energy sources, carbon capture technologies, etc.
3. Historical and Social Context:
- The evolution of steam turbine technology over time.
- The impact of steam turbines on industrial development and societal progress.
- The social and environmental implications of relying on steam turbines for power generation.
Steam Inlet
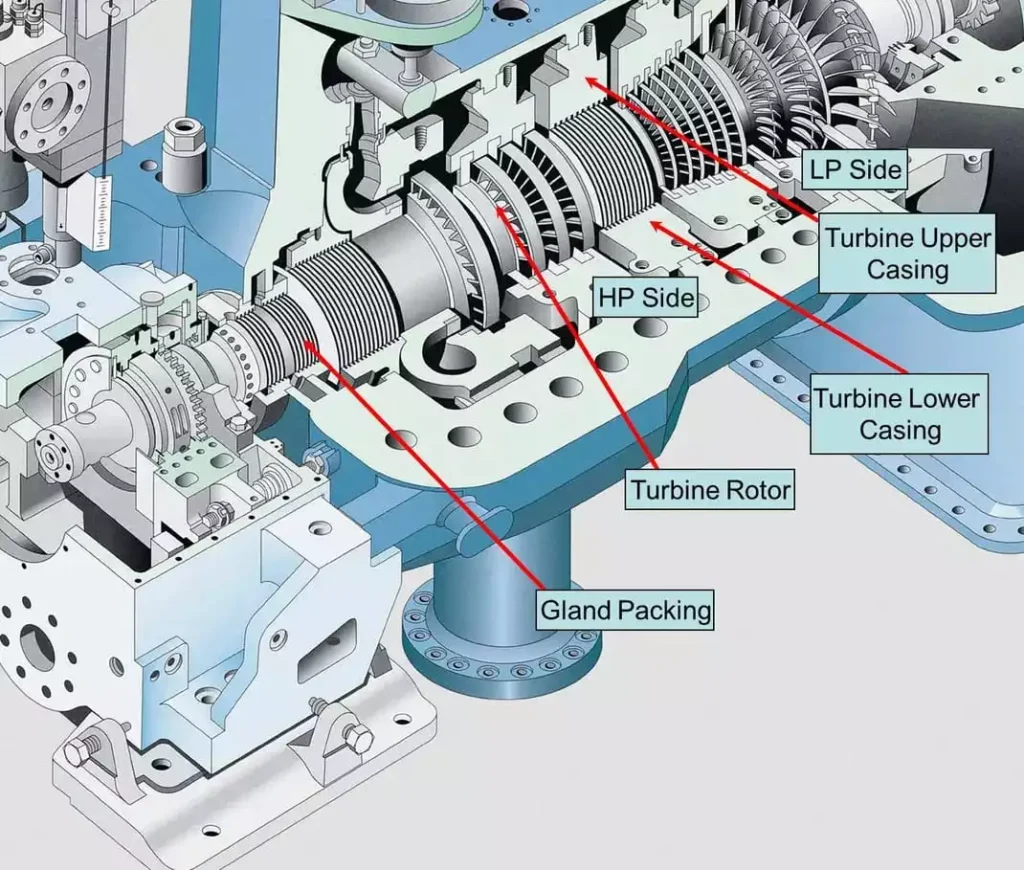
The steam inlet in an industrial turbine is more than just a simple pipe. It’s the crucial artery, the throbbing heart, the silent conductor of an energy symphony. To dive into the academic realm of this fascinating component, let’s explore several potential essay angles:
1. Engineering and Design:
- Optimizing Flow Efficiency: Analyze computational fluid dynamics simulations to explore how nozzle and channel shapes influence steam flow, pressure drop, and energy extraction. Discuss the use of advanced materials and coatings to reduce friction and wear.
- Advanced Materials and Stress Analysis: Research the challenges of high-pressure, high-temperature environments. Analyze material selection processes, considering creep, fatigue, and corrosion resistance. Explore novel materials like nickel superalloys and ceramic composites.
- Control Systems and Automation: Investigate the role of feedback loops and control valves in regulating steam flow and maximizing turbine performance. Discuss potential challenges like sensor accuracy and actuator response times.
- Novel Blade Design: Compare axial, radial, and mixed-flow designs. Analyze the impact of blade geometry, surface roughness, and cooling systems on efficiency and power output.
2. Applications and Impact:
- Power Generation Efficiency: Analyze the role of steam inlet design in minimizing energy losses and maximizing power output in specific power plant configurations (e.g., combined cycle, supercritical). Compare steam turbines with other power sources like gas turbines or renewables.
- Industrial Applications and Integration: Research the use of steam turbines in diverse industries like oil and gas, chemical processing, or papermaking. Analyze how steam inlet design adapts to specific process requirements and flow characteristics.
- Environmental Considerations: Evaluate the environmental impact of steam turbines compared to other power sources. Discuss potential mitigation strategies like carbon capture and storage, and explore the role of steam inlets in integrating with renewable energy sources.
3. Historical and Social Context:
- Evolution of Steam Inlet Design: Trace the historical development of steam inlet technology, highlighting key innovations and their impact on efficiency and performance.
- Industrial Revolution and Steam Power: Analyze the role of steam turbines as catalysts for industrial growth and societal progress. Discuss the social and economic implications of widespread steam power adoption.
- Future of Steam Inlets: Explore the potential for further optimization and integration with emerging technologies like advanced materials, additive manufacturing, and smart sensors. Discuss the role of steam turbines in a sustainable energy future.
Nozzle Ring
The nozzle ring, nestled within the steam inlet of an industrial turbine, plays a captivating role in this technological ballet. It’s an unsung hero, quietly shaping and directing the potent steam, transforming its raw energy into precise propulsion for the turbine blades. Let’s explore some compelling angles for an academic essay delving into this fascinating component:
1. Engineering and Design:
- Flow Optimization: Analyze the impact of nozzle geometry (angle, curvature, throat area) on steam flow velocity and energy extraction. Discuss the use of computational fluid dynamics simulations to optimize nozzle design for different operating conditions.
- Material Challenges: Investigate the harsh environment of high pressure, high temperature steam. Research material selection considerations for wear resistance, fatigue strength, and thermal stability. Explore cutting-edge materials like high-performance ceramics and composites.
- Manufacturing and Quality Control: Analyze the intricate manufacturing processes involved in producing nozzle rings, considering factors like machining precision, surface finish, and non-destructive testing methods.
- Advanced Control Systems: Investigate the potential for integrating smart sensors and actuators into nozzle rings to actively adjust steam flow for real-time optimization and dynamic response.
2. Applications and Impact:
- Performance Enhancement: Analyze the impact of nozzle ring design on overall turbine efficiency and power output. Compare different nozzle configurations for specific applications (e.g., variable-geometry nozzles for rapid load changes).
- Industrial Applications and Adaptability: Research the role of nozzle rings in diverse industrial settings like power generation, marine propulsion, chemical processing, and oil and gas production. Discuss how nozzle design adapts to different process requirements and steam conditions.
- Cost Considerations and Maintenance: Analyze the lifecycle costs of nozzle rings, including initial investment, maintenance needs, and potential replacement cycles. Discuss advanced coating technologies and wear mitigation strategies to extend lifespan.
3. Historical and Social Context:
- Evolution of Nozzle Ring Design: Trace the historical development of nozzle ring technology, highlighting key innovations and their impact on turbine efficiency and performance. Discuss the role of pioneering engineers and advancements in materials science.
- Industrial Revolution and Steam Power: Analyze the contribution of nozzle rings to the growth and efficiency of steam-powered machinery during the Industrial Revolution. Discuss the impact on societal progress and technological advancements.
- Future of Nozzle Rings: Explore the potential for further optimization of nozzle ring design through additive manufacturing, advanced materials, and integration with artificial intelligence for real-time control and predictive maintenance.
Rotor and Blades
1. Engineering and Design:
- Blade Design Optimization: Analyze the impact of blade geometry (airfoil shape, twist angle, tip clearance) on aerodynamic efficiency, pressure drop, and stress distribution. Discuss the use of computational fluid dynamics to optimize blade design for specific operating conditions.
- Material Challenges: Investigate the harsh environment of high-speed rotation, high temperature steam, and potential erosion. Research material selection considerations for fatigue strength, creep resistance, and corrosion resistance. Explore advanced materials like titanium alloys and fiber-reinforced composites.
- Manufacturing and Quality Control: Analyze the intricate manufacturing processes involved in producing turbine blades, considering factors like precision casting, forging, machining, and non-destructive testing methods.
- Advanced Cooling Systems: Discuss the importance of blade cooling for maintaining efficiency and preventing thermal failure. Explore internal and external cooling techniques like film cooling, impingement cooling, andtranspiration cooling.
2. Applications and Impact:
- Efficiency and Power Output: Analyze the impact of rotor and blade design on overall turbine efficiency and power generation. Compare different blade configurations for specific applications (e.g., impulse vs. reaction blades, single-stage vs. multi-stage turbines).
- Industrial Applications and Adaptability: Research the role of rotor and blade design in diverse industrial settings like power generation, marine propulsion, chemical processing, and oil and gas production. Discuss how blade design adapts to different process requirements and steam conditions.
- Maintenance and Repair: Analyze the challenges of blade erosion, corrosion, and fatigue damage. Discuss preventive maintenance strategies and advanced repair techniques like blade coating and laser cladding.
3. Historical and Social Context:
- Evolution of Rotor and Blade Design: Trace the historical development of rotor and blade technology, highlighting key innovations and their impact on turbine efficiency and performance. Discuss the role of pioneering engineers like Charles Parsons and Aegidius Ellinghaus.
- Industrial Revolution and Steam Power: Analyze the contribution of advanced rotor and blade design to the growth and efficiency of steam-powered machinery during the Industrial Revolution. Discuss the impact on societal progress and technological advancements.
- Future of Rotors and Blades: Explore the potential for further optimization of rotor and blade design through additive manufacturing, advanced materials, and integrated sensors for real-time monitoring and predictive maintenance.
Stator and Casing
1. Engineering and Design:
- Flow Optimization: Analyze the design of the stator vanes and internal channels to ensure smooth and efficient steam flow while minimizing pressure drop and turbulence. Discuss the use of computational fluid dynamics simulations to optimize internal geometry for different operating conditions.
- Material Challenges: Investigate the high-pressure, high-temperature environment and the need for robust materials resistant to creep, fatigue, and thermal expansion. Explore advanced materials like high-strength steels and nickel alloys.
- Structural Integrity: Analyze the complex stress distribution within the stator and casing due to high pressure and rotational forces. Discuss design considerations for maintaining structural integrity and preventing potential failures.
- Sealing and Leakage Control: Investigate the critical role of seals and gaskets in minimizing steam leakage between the stator and rotor. Discuss advanced sealing technologies and materials to ensure optimal efficiency and prevent energy losses.
2. Applications and Impact:
- Safety and Reliability: Analyze the contribution of the stator and casing to the overall safety and reliability of the turbine. Discuss design features like pressure relief valves, containment systems, and vibration damping mechanisms.
- Maintenance and Repair: Investigate the challenges of inspecting and maintaining the internal components of the stator and casing. Discuss non-destructive testing methods and strategies for minimizing downtime during maintenance.
- Integration with Other Turbine Components: Analyze the role of the stator and casing in interacting with other turbine components like the steam inlet, rotor and blades, and exhaust outlet. Discuss how design optimizations impact overall performance and efficiency.
3. Historical and Social Context:
- Evolution of Stator and Casing Design: Trace the historical development of stator and casing technology, highlighting key innovations and their impact on turbine safety, reliability, and efficiency. Discuss the challenges faced by early engineers and the role of materials science advancements.
- Industrial Revolution and Steam Power: Analyze the contribution of improved stator and casing design to the widespread adoption of steam-powered machinery during the Industrial Revolution. Discuss the safety considerations and advancements that enabled large-scale power generation.
- Future of Stators and Casings: Explore the potential for further optimization of stator and casing design through additive manufacturing, advanced materials, and integrated sensors for real-time monitoring and condition-based maintenance.
Exhaust Outlet
1. Engineering and Design:
- Flow Optimization: Analyze the design of the exhaust outlet to minimize pressure drop and ensure smooth flow of spent steam without impacting upstream performance. Discuss the use of diffusers and flow straighteners to optimize flow characteristics and energy recovery.
- Noise and Vibration Control: Investigate the potential for noise generation and vibration transmission through the exhaust outlet. Discuss design features like silencers, baffles, and vibration dampers to minimize environmental impact and protect surrounding equipment.
- Material Challenges: Consider the high-temperature environment and potential erosion from residual steam particles. Explore material selection considerations for heat resistance, wear resistance, and corrosion resistance.
- Integration with Other Systems: Analyze the role of the exhaust outlet in connecting the turbine to downstream systems like condensers, waste heat recovery units, or pollution control equipment. Discuss design considerations for compatibility and optimal energy utilization.
2. Applications and Impact:
- Efficiency and Power Generation: Analyze the impact of exhaust outlet design on overall turbine efficiency by minimizing energy losses through backpressure. Discuss strategies for waste heat recovery from the exhaust steam for additional power generation or industrial processes.
- Environmental Considerations: Investigate the contribution of the exhaust outlet to emissions control and noise pollution mitigation. Discuss design features and potential retrofitting options to comply with environmental regulations and minimize environmental impact.
- Industrial Applications and Adaptability: Research the role of exhaust outlet design in diverse industrial settings like power generation, marine propulsion, chemical processing, and papermaking. Discuss how design adapts to different process requirements and downstream equipment configurations.
3. Historical and Social Context:
- Evolution of Exhaust Outlet Design: Trace the historical development of exhaust outlet technology, highlighting key innovations and their impact on turbine efficiency, environmental compatibility, and noise reduction. Discuss the challenges faced by early engineers and the role of advancements in materials science and fluid dynamics.
- Industrial Revolution and Steam Power: Analyze the contribution of improved exhaust outlet design to the widespread adoption of steam-powered machinery during the Industrial Revolution. Discuss the environmental concerns and social pressures that drove engineering advancements in this area.
- Future of Exhaust Outlets: Explore the potential for further optimization of exhaust outlet design through computational fluid dynamics simulations, advanced materials like heat-resistant composites, and integration with intelligent control systems for adaptive flow management and noise reduction.
Power Generation
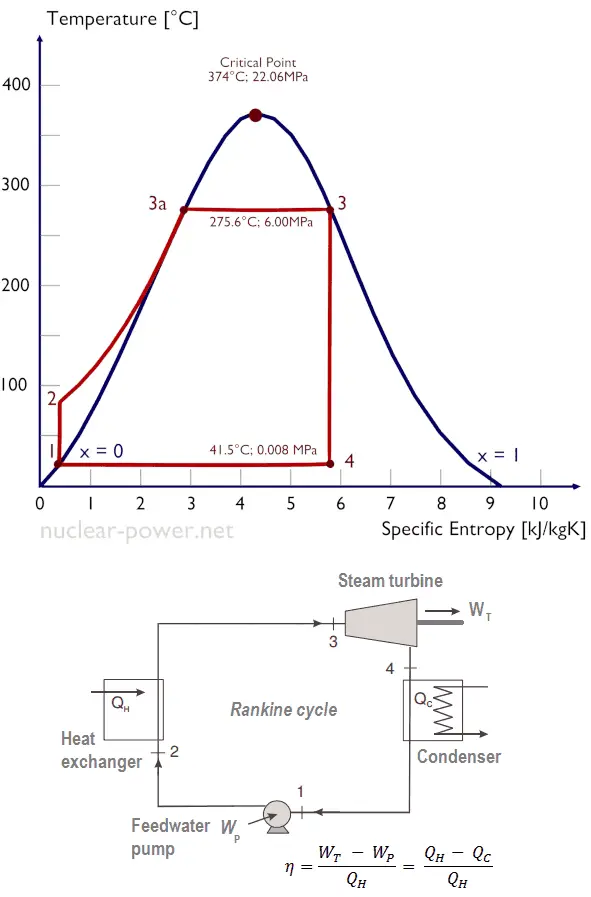
1. Technological Advancements and Efficiency:
- Emerging technologies: Dive into the world of renewable energy sources like solar, wind, geothermal, and ocean currents, analyzing their integration with traditional methods like hydroelectric and nuclear power.
- Material science and engineering: Explore the role of advanced materials like high-temperature superconductors, nanocomposites, and energy-harvesting materials in boosting efficiency and reducing environmental impact.
- Distributed generation and microgrids: Investigate the shift towards decentralized power systems, analyzing their potential for improving grid resilience, reducing transmission losses, and empowering local communities.
- Artificial intelligence and optimization: Discuss the implementation of AI in power plant control systems, forecasting energy demand, and optimizing energy distribution for greater efficiency and reliability.
2. Sustainability and Environmental Considerations:
- Decarbonization strategies: Analyze the various pathways towards achieving net-zero carbon emissions in the power sector, including carbon capture and storage, renewable energy integration, and energy efficiency initiatives.
- Environmental impact assessment: Delve into the environmental impacts of different power generation technologies, considering factors like air and water pollution, land use, and ecological balance.
- Life cycle analysis: Conduct a comprehensive life cycle analysis of various power generation options, encompassing resource extraction, infrastructure development, energy production, and waste management.
- Energy justice and equity: Explore the social and economic implications of different power generation choices, ensuring equitable access to clean energy and mitigating negative impacts on vulnerable communities.
3. Economic and Policy Dimensions:
- Energy market restructuring: Analyze the changing landscape of energy markets, considering the rise of renewables, distributed generation, and smart grids, and their impact on traditional power generation models.
- Energy policy and regulation: Discuss the role of government policies and regulations in shaping the future of power generation, including incentives for renewable energy, carbon pricing schemes, and grid modernization initiatives.
- Investment and financing: Investigate the financial aspects of different power generation options, considering their capital costs, operating expenses, ROI potential, and risk factors for investors.
- Geopolitics and energy security: Examine the intersection of global politics and energy security, analyzing how dependence on specific energy sources can impact international relations and regional stability.
Marine Propulsion
1. Technological Advancements and Efficiency:
- Beyond the propeller: Dive deeper than the traditional screw propeller, exploring innovative propulsion technologies like hydrofoils, water jets, and even wind-assisted sails. Analyze their efficiency, maneuverability, and environmental impact compared to conventional methods.
- Fuel options and decarbonization: Examine the shift away from fossil fuels in marine propulsion. Explore the potential of alternative fuels like hydrogen, ammonia, and biofuels, analyzing their infrastructure needs, cost-effectiveness, and emission reduction potential.
- Electric and hybrid propulsion: Investigate the rising tide of electric and hybrid-powered vessels. Unpack the challenges of battery range and charging infrastructure, while exploring the benefits of emissions reduction and noise reduction.
- Automation and intelligent systems: Discuss the role of AI and smart control systems in optimizing fuel consumption, route planning, and collision avoidance for safer and more efficient maritime operations.
2. Sustainability and Environmental Considerations:
- Ballast water management: Analyze the risks and regulations surrounding ballast water discharge, exploring eco-friendly treatment methods to prevent the spread of invasive species and protect marine ecosystems.
- Noise pollution and underwater soundscapes: Investigate the impact of underwater noise from propellers and engines on marine life, particularly communication and migration patterns. Discuss mitigation strategies and quieter propulsion technologies.
- Oil spills and pollution prevention: Analyze the devastating consequences of oil spills and explore technologies and regulations to prevent accidents and ensure responsible waste disposal at sea.
- Sustainable fisheries and ocean conservation: Discuss the role of marine propulsion in sustainable fishing practices, minimizing harmful impacts on fish stocks and ensuring responsible resource management.
3. Economic and Policy Dimensions:
- International maritime regulations: Examine the complex web of international regulations governing maritime emissions, fuel standards, and safety protocols. Analyze the challenges of enforcement and the role of flag states in ensuring compliance.
- Port infrastructure and shore power: Discuss the need for adequate shore power facilities and charging infrastructure to support cleaner ship operations while docked, reducing air and noise pollution in port cities.
- Economic viability and subsidies: Analyze the financial incentives and subsidies needed to encourage adoption of cleaner and more efficient marine propulsion technologies in the current economic landscape.
- Geopolitical competition and strategic interests: Explore the intersection of maritime trade, national security, and control over key shipping routes, analyzing how advancements in propulsion technology can impact geopolitical dynamics.
Industrial Applications
1. Efficiency and Productivity Enhancements:
- Power generation and cogeneration: Analyze how steam turbines contribute to efficient electricity production in various industries, including oil and gas, chemical processing, and papermaking. Explore cogeneration options to maximize energy utilization and minimize waste.
- Process Optimization and Control: Investigate how steam turbines drive pumps, compressors, and other critical equipment in diverse industrial processes. Analyze the use of variable-speed controls and advanced automation for optimal process efficiency and production capacity.
- Waste Heat Recovery and Energy Saving: Dive into the world of waste heat recovery systems, leveraging steam turbines to utilize residual heat from industrial processes for additional power generation or other energy-intensive tasks. Discuss the economic and environmental benefits of these closed-loop systems.
- Maintenance and Reliability Optimizations: Explore how predictive maintenance tools and sensor-based monitoring of steam turbines contribute to extended equipment life and minimized downtime. Analyze the potential of AI and machine learning for data-driven maintenance strategies.
2. Environmental Considerations and Sustainability:
- Decarbonization Strategies: Investigate the role of steam turbines in integrating renewable energy sources like biomass or geothermal power into industrial processes. Analyze the potential for carbon capture and storage technologies to mitigate emissions from steam turbine operations.
- Circular Economy and Resource Recovery: Explore how steam turbines can power equipment for recycling and waste treatment, contributing to a closed-loop circular economy within industries. Analyze the environmental and economic benefits of resource recovery and material reuse.
- Water Management and Conservation: Discuss the challenges of water usage in industrial steam turbine systems. Analyze water treatment technologies and closed-loop cooling systems to minimize freshwater consumption and wastewater discharge.
- Life Cycle Assessment and Environmental Impact: Conduct a comprehensive life cycle assessment of steam turbine use in specific industries, considering resource extraction, infrastructure development, energy production, and waste management. Evaluate the overall environmental footprint and potential mitigation strategies.
3. Industry-Specific Applications and Challenges:
- Chemical Processing: Analyze the diverse roles of steam turbines in chemical production, from driving compressors and mixers to providing process heat and generating electricity. Discuss specific applications and challenges within different chemical sub-sectors.
- Oil and Gas Exploration and Production: Dive into the crucial role of steam turbines in offshore platforms and onshore facilities for oil and gas extraction, processing, and transportation. Discuss safety considerations and specific equipment configurations.
- Food and Beverage Industry: Explore the use of steam turbines for sterilization, cleaning, and other energy-intensive processes in food and beverage manufacturing. Analyze the need for food-grade steam hygiene and quality control.
- Textile and Paper Production: Investigate the applications of steam turbines in fiber processing, drying, and papermaking. Discuss specific challenges related to moisture control and efficient heat transfer within these industries.
Industrial steam turbines are integral to modern energy systems, converting heat energy into mechanical work to generate electricity or drive machinery. These turbines have evolved over a century of development, from early designs that powered factories and ships to highly advanced systems that drive today’s power plants and industrial processes.
Early steam turbines laid the groundwork for the rapid industrialization of the 20th century. Today, steam turbines are widely used in power generation, petrochemicals, pulp and paper, and even food processing industries. This document explores their operation, types, applications, and future developments, reflecting their continuing role in global industrial energy systems.
Basic Principles of Steam Turbines
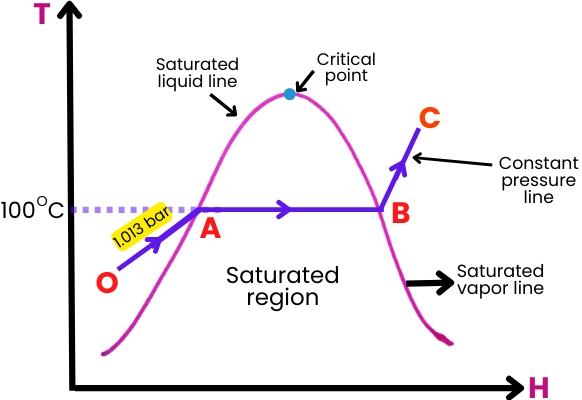
At their core, steam turbines operate by harnessing the thermal energy in steam to perform mechanical work. The process begins by superheating water in a boiler, which transforms into steam. This high-pressure steam is directed through a series of turbine blades, causing the rotor to spin, converting thermal energy into mechanical energy.
Key topics:
- Thermodynamics: Discuss the Rankine cycle, which is the basic thermodynamic cycle governing steam turbine operations.
- Mechanical to Electrical Energy: How generators convert mechanical rotation into electricity.
- Saturated vs. Superheated Steam: How different steam states affect efficiency and output.
Types of Industrial Steam Turbines
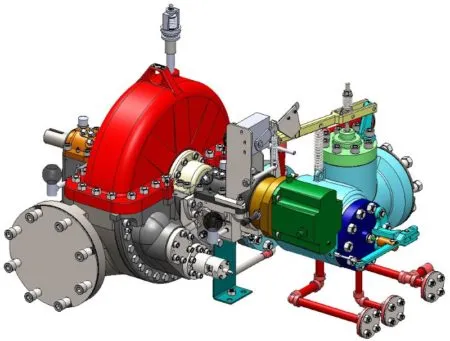
Industrial steam turbines come in various types based on application and design. This section will outline the main categories:
- Condensing Turbines: Commonly used in power generation, these turbines release exhaust steam at low pressures to maximize energy extraction.
- Back-pressure (Non-condensing) Turbines: Typically used in cogeneration applications, these turbines exhaust steam at higher pressures for additional industrial processes like heating.
- Impulse vs. Reaction Turbines: Impulse turbines use high-pressure steam jets to impact blades, while reaction turbines work by steam expansion along the blade surface.
Design and Construction of Steam Turbines
Steam turbines consist of several critical components designed for efficiency and durability:
- Rotor and Blades: Discuss the importance of blade design in maximizing energy transfer, the use of high-strength materials, and anti-corrosion coatings.
- Casing and Nozzles: The role of high-pressure steam in efficient turbine operation.
- Blade Materials: High-temperature alloys and innovations in metallurgy.
This section will also touch on challenges like managing vibration, noise, and blade fatigue, as well as technological advances in blade cooling techniques and aerodynamic designs.
Operation and Performance
Operating steam turbines efficiently requires careful monitoring and control of various parameters, including steam pressure, temperature, and flow rate. This section will describe typical start-up and shutdown procedures, as well as strategies for performance optimization through steam quality management and operational adjustments.
Key topics:
- Operational Efficiency: Analyzing key metrics like thermal efficiency, mechanical losses, and parasitic losses.
- Common Operational Issues: Addressing corrosion, erosion, and steam quality.
- Maintenance Best Practices: Routine inspections, preventive maintenance schedules, and component replacement strategies.
Applications in Power Generation
Steam turbines are the backbone of thermal power plants, whether fossil fuel-based, nuclear, or renewable. This section will cover the various types of power plants where steam turbines are used, focusing on their role in:
- Coal, Gas, and Oil-fired Power Plants: Discuss the Rankine cycle’s role in fossil-fuel power generation.
- Nuclear Power: How steam turbines function in nuclear plants, particularly in pressurized and boiling water reactors.
- Renewable Energy Integration: The role of steam turbines in biomass and geothermal power generation.
- Combined-cycle Power Plants: A comparison with gas turbines in modern power systems.
Industrial Applications Beyond Power Generation
While power generation is the most common application, steam turbines are also widely used in various industrial processes:
- Cogeneration and District Heating: How industrial plants use steam turbines to produce both power and heat.
- Petrochemical Industry: The role of turbines in refining processes.
- Pulp and Paper Manufacturing: Steam turbines for combined heat and power in paper mills.
- Desalination: The integration of steam turbines in large-scale desalination plants.
Efficiency and Performance Improvements
Advances in steam turbine design are continuously pushing the boundaries of efficiency. Some areas of improvement include:
- Blade Design and Aerodynamics: How modern blade profiles maximize efficiency.
- Digitalization: The role of sensors and AI in monitoring performance and predicting maintenance needs.
- Retrofitting Older Systems: Updating older turbines with modern technology for better performance.
Challenges and Limitations
Industrial steam turbines face challenges related to:
- Material Limitations: Corrosion, erosion, and fouling are major concerns.
- Regulatory Pressures: Compliance with emissions standards and efficiency benchmarks.
- Competitors: How steam turbines compare with other technologies like gas and wind turbines.
Future Trends and Innovations
The future of industrial steam turbines will be shaped by technological advancements and global energy trends:
- Hybrid Systems: Combining steam and gas turbines for enhanced efficiency.
- Hydrogen Integration: The potential of hydrogen-powered steam systems.
- AI and IoT: Predictive maintenance and real-time performance monitoring for better operational efficiency.
Conclusion
The conclusion will summarize the key points covered in the document, highlighting the continuing relevance of industrial steam turbines in both traditional and modern energy landscapes. It will also touch on the potential future developments and innovations that could reshape the role of steam turbines in global energy systems.
Industrial Steam Turbines
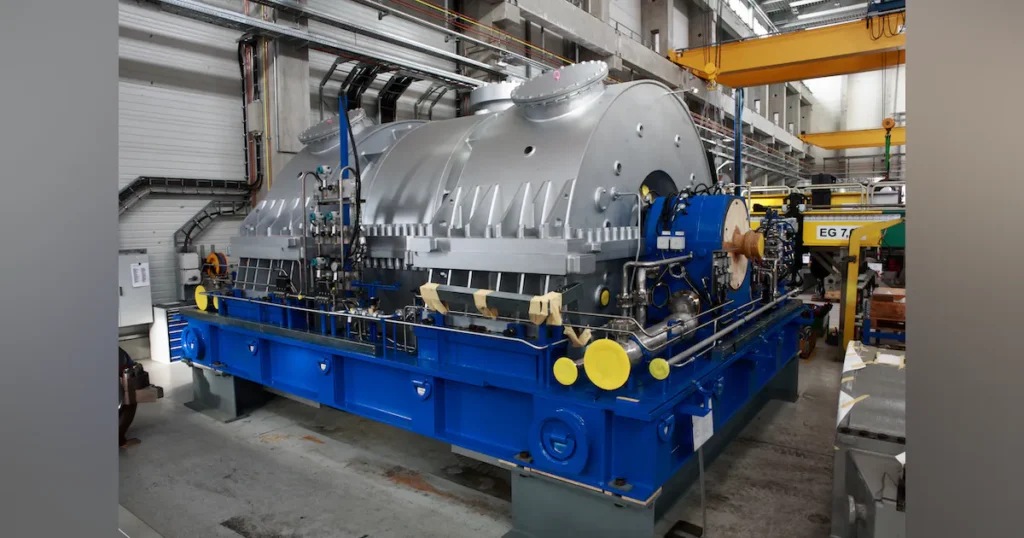
Industrial steam turbines are fundamental components of power generation and industrial processes worldwide, playing a critical role in converting heat energy into mechanical work and electricity. From small-scale applications in manufacturing plants to massive power generation facilities, steam turbines remain one of the most efficient and reliable technologies for energy conversion. These turbines function by utilizing high-pressure steam produced by heating water in boilers. The steam passes through a series of blades, causing the turbine to rotate and generate mechanical work. This process, dating back more than a century, remains crucial in the modern industrial landscape.
Historically, steam turbines were developed in the late 19th century as an evolution of earlier steam engines, which were significantly less efficient and more cumbersome. The invention of the steam turbine is often credited to Sir Charles Parsons, who introduced the first practical design in 1884. His design revolutionized marine propulsion, power generation, and many industrial processes, ushering in an era of rapid industrialization. As the industrial revolution progressed, steam turbines became more advanced and widely adopted in power plants, oil refineries, chemical processing plants, and other sectors.
Today, steam turbines are key components in a broad range of energy systems, from fossil fuel-based power plants to renewable energy setups. In power generation, they are primarily used in thermal plants, where coal, gas, or nuclear reactions heat water to create steam. In industrial applications, steam turbines drive machinery, compress gases, or generate electricity as part of cogeneration systems. These turbines can range in size from small units producing a few megawatts to giant turbines capable of generating several hundred megawatts, depending on the application.
Modern steam turbine technology has advanced to achieve high efficiencies through innovative materials, precision engineering, and digital monitoring systems. The versatility of steam turbines allows them to be integrated into diverse energy systems, including combined-cycle power plants, where they work in tandem with gas turbines, and geothermal or biomass plants, where they harness renewable steam sources.
With increasing global energy demands and the transition toward cleaner and more efficient energy sources, steam turbines continue to evolve. Efficiency improvements, integration with renewable energy technologies, and advancements in digitalization, such as predictive maintenance and AI-driven monitoring systems, are shaping the future of steam turbines. They also play a vital role in decarbonization efforts, particularly when coupled with carbon capture technologies or integrated into hybrid energy systems.
In conclusion, industrial steam turbines are integral to the global energy infrastructure, providing reliable, efficient, and scalable power generation solutions. From their historical origins to modern-day innovations, they remain a cornerstone of both traditional and emerging energy systems, ensuring their relevance well into the future.
Basic Principles of Steam Turbines
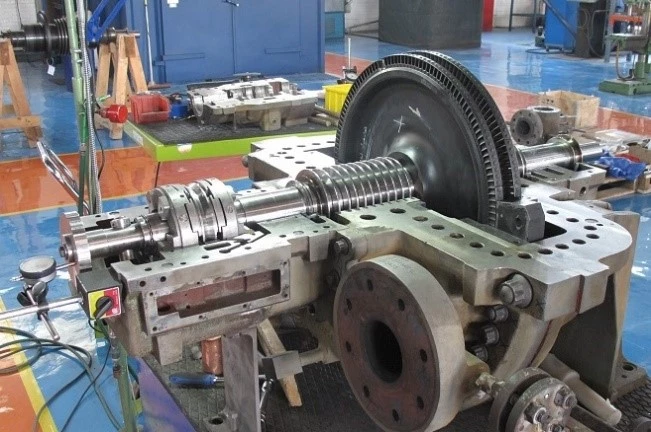
Industrial steam turbines operate based on well-established thermodynamic principles, converting thermal energy from steam into mechanical work. This section will discuss the key principles, energy conversion process, steam properties, and the basic components that make up a steam turbine.
Thermodynamics of Steam Power
Steam turbines rely on the Rankine cycle, a thermodynamic process that describes how heat energy is converted into mechanical work in a closed-loop system. The cycle consists of four main stages:
- Heat Addition (Boiler): Water is heated in a boiler until it becomes steam, either saturated or superheated, depending on the application. This phase is crucial for generating the high-pressure steam needed to drive the turbine.
- Expansion (Turbine): The high-pressure steam is directed onto turbine blades, causing them to rotate. As the steam expands through the turbine stages, it loses pressure and temperature, transferring its energy to the rotor. This expansion is the core process where thermal energy is converted into mechanical energy.
- Condensation (Condenser): After passing through the turbine, the low-pressure steam enters a condenser, where it is cooled and returned to liquid form. This process helps maintain efficiency by creating a pressure difference that drives steam flow through the turbine.
- Pressurization (Pump): The condensed water is pumped back to the boiler under high pressure, completing the cycle.
The Rankine cycle is the most commonly used thermodynamic process in industrial steam turbines, particularly in power generation. Its efficiency depends on factors such as the temperature and pressure of the steam entering the turbine, the effectiveness of the condenser, and the efficiency of the turbine blades.
Conversion of Thermal Energy to Mechanical Energy
The primary purpose of a steam turbine is to convert thermal energy from steam into mechanical work. This energy conversion occurs when high-pressure steam enters the turbine and interacts with the blades. Steam turbines use either impulse or reaction principles to achieve this conversion, depending on their design.
- Impulse Principle: In impulse turbines, high-pressure steam is directed through nozzles, which convert the steam’s thermal energy into kinetic energy. The resulting high-speed steam jets strike the turbine blades, causing them to rotate. The pressure remains constant as the steam flows through the turbine stages, while its velocity decreases. Impulse turbines are commonly used in applications where high-pressure steam is available, such as in power plants.
- Reaction Principle: In reaction turbines, steam expands continuously as it passes over the turbine blades, which are designed to act as nozzles. The pressure drop occurs directly within the blade passages, and both the velocity and pressure of the steam decrease as it progresses through the turbine. Reaction turbines are typically used in applications with lower pressure or when maximizing efficiency at lower steam velocities is essential.
Both impulse and reaction turbines are often combined in multi-stage configurations to optimize energy conversion across different steam pressure and velocity ranges.
Types of Steam: Saturated vs. Superheated
The properties of the steam used in a turbine significantly impact its performance and efficiency. Two key types of steam are commonly used in industrial steam turbines:
- Saturated Steam: Saturated steam exists at a temperature corresponding to the boiling point of water at a given pressure. It contains a mixture of water vapor and liquid, making it ideal for applications where heat recovery is required, such as in cogeneration systems. However, because saturated steam contains water droplets, it can cause erosion and reduce the efficiency of turbine blades over time.
- Superheated Steam: Superheated steam is produced by heating saturated steam beyond its boiling point, at which point it becomes completely dry and free of water droplets. Superheated steam is preferred for most power generation applications because it allows for greater efficiency. The absence of moisture reduces the risk of blade erosion, and its higher temperature provides more energy for conversion into mechanical work.
The choice between saturated and superheated steam depends on the specific application, with superheated steam offering advantages in high-efficiency power generation systems.
Basic Components of a Steam Turbine
Steam turbines consist of several key components, each of which plays a crucial role in the energy conversion process:
- Rotor: The rotor is the central rotating part of the turbine, onto which the blades are mounted. As the steam flows through the turbine, it causes the rotor to spin, converting thermal energy into mechanical work. The rotor is typically supported by bearings and connected to a generator or mechanical system to perform useful work.
- Blades: Turbine blades are the primary components that interact with the steam. They are precisely engineered to extract maximum energy from the high-pressure steam. Blades in impulse turbines are shaped to change the direction of the steam jets, while reaction turbine blades are designed to create pressure drops as the steam passes over them. Blade materials must withstand high temperatures and stresses, and they are often made of heat-resistant alloys or coated with protective materials to prevent erosion.
- Casing: The casing encloses the turbine and contains the steam as it flows through the turbine stages. It is designed to withstand high pressures and temperatures. Casings are typically made from materials that can tolerate the thermal expansion and mechanical stresses caused by the steam.
- Nozzles: In impulse turbines, nozzles play a critical role in converting the thermal energy of the steam into kinetic energy. These nozzles direct high-speed steam jets onto the blades, ensuring efficient energy transfer.
- Bearings: Bearings support the rotor and allow it to spin smoothly. They must be capable of handling the high-speed rotation and the weight of the turbine assembly while minimizing friction and wear.
- Condenser: In condensing turbines, the condenser is responsible for cooling and condensing the exhaust steam back into water. This process reduces the steam’s pressure, enhancing the efficiency of the turbine by maintaining a large pressure differential across the turbine stages.
Together, these components form a highly efficient machine capable of converting the thermal energy in steam into mechanical work, which can then be used for power generation or other industrial processes.
Types of Industrial Steam Turbines
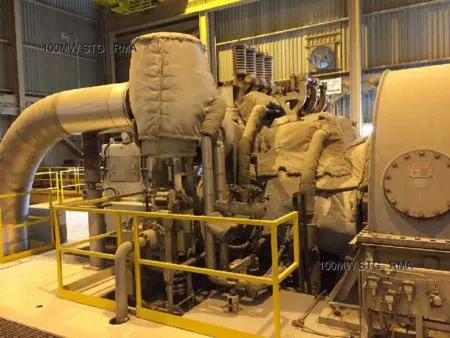
Industrial steam turbines are designed to meet various energy conversion needs across different sectors. Depending on the application, steam conditions, and desired output, steam turbines can be classified into several types. The most common types include condensing turbines, non-condensing (back-pressure) turbines, and extraction turbines. Additionally, turbines can be categorized based on their working principles into impulse turbines and reaction turbines. Understanding these types and their functions is key to selecting the appropriate turbine for a given application.
Condensing Turbines
Condensing steam turbines are one of the most widely used types in industrial power generation, particularly in large-scale power plants. These turbines operate by condensing the exhaust steam after it passes through the turbine, allowing for maximum energy extraction. The condensing process significantly lowers the pressure of the exhaust steam, thereby increasing the pressure differential between the steam entering and leaving the turbine. This increased pressure difference allows the turbine to extract as much energy as possible from the steam.
- Mechanism: In a condensing turbine, steam is introduced at a high pressure and temperature into the turbine. As the steam passes through multiple stages of the turbine, it expands and loses pressure and temperature, causing the rotor to spin. After completing the energy conversion process, the low-pressure exhaust steam is directed into a condenser, where it is cooled and converted back into liquid water. The condensed water is then pumped back into the boiler to repeat the cycle.
- Applications: Condensing turbines are primarily used in large-scale thermal power plants, including coal, natural gas, and nuclear power stations. They are designed to generate electricity efficiently by maximizing energy extraction from the steam. In these plants, the condenser is often connected to a cooling tower or a body of water to remove the heat generated during the condensation process.
- Advantages:
- High efficiency due to the large pressure drop across the turbine.
- Ideal for applications focused solely on power generation.
- Disadvantages:
- Condensers require significant amounts of cooling water, which may not be available in all locations.
- The overall system is more complex, requiring additional equipment such as condensers and cooling towers.
Non-condensing (Back-pressure) Turbines
Non-condensing, or back-pressure turbines, are used in applications where the steam is needed for industrial processes after passing through the turbine. Unlike condensing turbines, these turbines do not exhaust steam at low pressures. Instead, the exhaust steam is used at a higher pressure for additional purposes, such as heating or driving industrial processes.
- Mechanism: In a back-pressure turbine, high-pressure steam enters the turbine and expands, causing the rotor to rotate and perform mechanical work. However, instead of being condensed, the exhaust steam exits the turbine at a relatively high pressure. This steam can then be used for other processes, such as heating buildings, drying products, or running auxiliary equipment in industrial plants.
- Applications: Back-pressure turbines are commonly used in cogeneration systems, where both electricity and heat are produced simultaneously. Industrial plants, such as chemical factories, pulp and paper mills, and refineries, often use back-pressure turbines to generate electricity while using the exhaust steam for heating or other processes. This makes them highly efficient for applications that require both power and steam.
- Advantages:
- Highly efficient in cogeneration applications since both the electricity and steam are utilized.
- Lower capital costs compared to condensing turbines, as condensers and cooling systems are not needed.
- Ideal for applications where steam is required for processes beyond power generation.
- Disadvantages:
- Less efficient for purely power generation purposes, as not all energy is extracted from the steam.
- Limited to locations or industries where the exhaust steam can be effectively utilized.
Extraction and Regenerative Turbines
Extraction turbines are a hybrid design that allows steam to be extracted at one or more intermediate points during its expansion process within the turbine. This design enables the turbine to supply steam at different pressures for industrial processes, while still generating electricity. The remaining steam continues to expand and is either exhausted as in a back-pressure turbine or condensed as in a condensing turbine.
- Mechanism: In extraction turbines, steam is partially expanded in the turbine to generate power. At one or more stages of the turbine, a portion of the steam is “extracted” and redirected for use in other processes. The remaining steam continues through the turbine for further energy extraction. By controlling the amount and pressure of the extracted steam, the turbine can meet varying steam demands while continuing to generate electricity.
- Applications: Extraction turbines are frequently used in cogeneration and combined heat and power (CHP) plants, where steam is needed for both electricity generation and industrial applications. Industries such as chemical processing, refineries, and paper mills benefit from this type of turbine, as they can use the extracted steam for heating or other processes while producing electricity.
- Advantages:
- Flexible operation: Steam can be extracted at different pressures to meet various process requirements.
- Efficient use of steam for both power generation and industrial processes.
- Disadvantages:
- More complex control systems are needed to manage steam extraction and maintain turbine efficiency.
- The design is more complicated compared to non-extraction turbines, leading to higher initial costs.
Impulse vs. Reaction Turbines
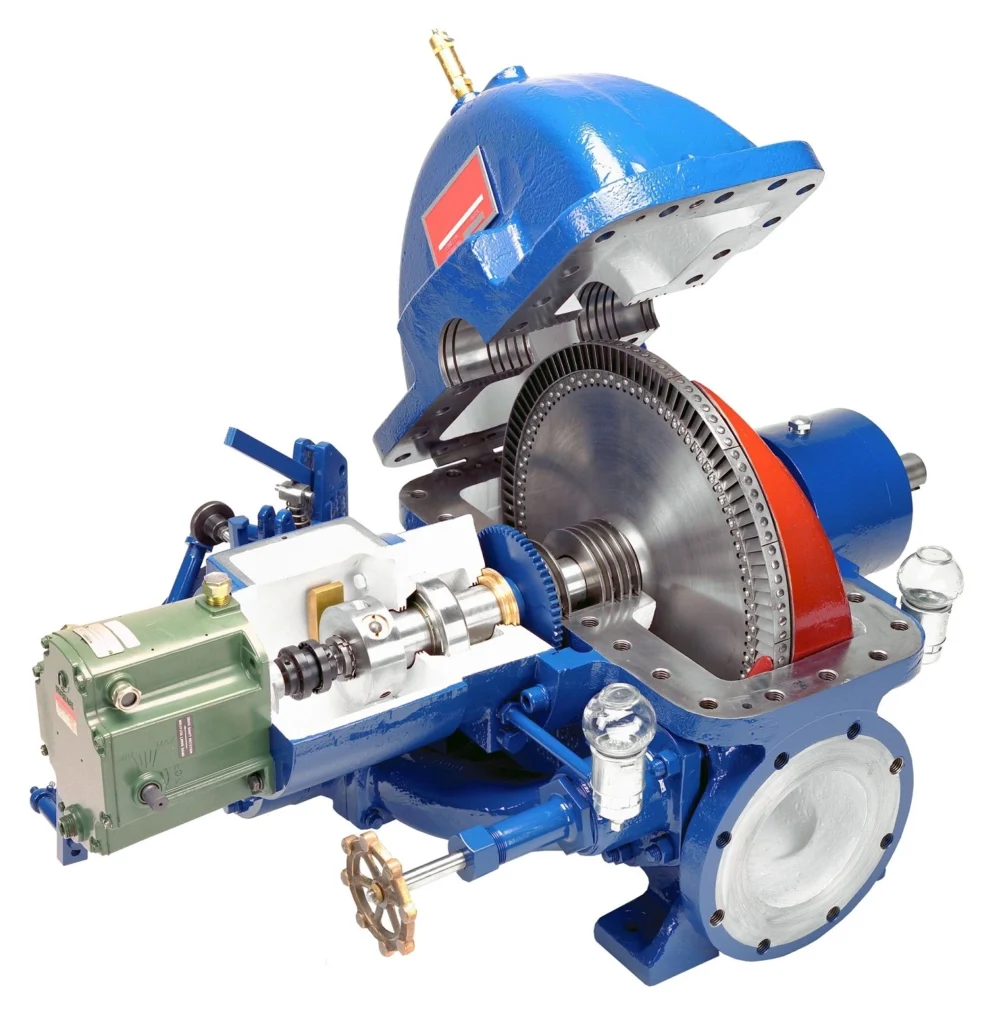
Steam turbines are also categorized based on the mechanism they use to convert the steam’s energy into mechanical work. The two main types are impulse turbines and reaction turbines.
- Impulse Turbines: In an impulse turbine, steam is directed through nozzles that convert the steam’s pressure energy into high-velocity jets. These jets then strike the blades of the turbine, causing the rotor to spin. The pressure of the steam remains constant as it passes through the blades, while its velocity decreases. Impulse turbines are often used in applications with high-pressure steam and are known for their simplicity and durability.
- Applications: Impulse turbines are used in high-pressure stages of steam power plants and in marine propulsion systems.
- Advantages:
- Simple design with fewer stages, which reduces mechanical complexity.
- High durability and resistance to damage from steam impurities.
- Disadvantages:
- Less efficient in converting energy at low pressures, leading to a need for multi-stage setups in many applications.
- Reaction Turbines: In a reaction turbine, the steam expands as it passes over the blades, causing a continuous pressure drop. Both the moving and stationary blades act as nozzles, with the pressure energy being converted into both velocity and mechanical work. Reaction turbines are commonly used in lower-pressure applications and provide higher efficiency in these conditions.
- Applications: Reaction turbines are often used in lower-pressure stages of power plants, as well as in applications where steam pressure is lower to begin with.
- Advantages:
- Higher efficiency at lower steam pressures and velocities.
- Smoother operation due to gradual energy transfer.
- Disadvantages:
- More complex design with more stages, leading to higher manufacturing and maintenance costs.
- Increased susceptibility to damage from steam impurities, requiring better steam quality.
Combination of Impulse and Reaction Turbines
In practice, many industrial steam turbines use a combination of both impulse and reaction designs to optimize efficiency across different pressure and temperature stages. For example, the high-pressure stages may use impulse turbines, while the lower-pressure stages use reaction turbines, creating a highly efficient energy conversion system.
Design and Construction of Steam Turbines
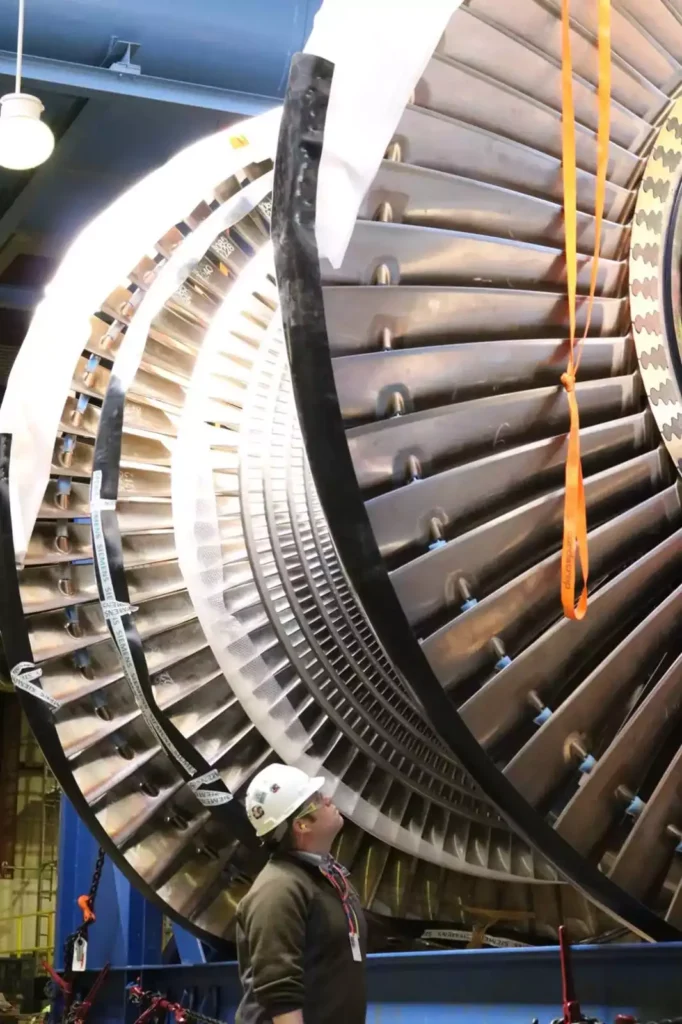
Steam turbine design is a complex engineering discipline aimed at maximizing the efficiency and reliability of the machine. A typical industrial steam turbine consists of several critical components, each designed to withstand high temperatures, pressures, and mechanical stresses. This section will focus on the design of major components such as the rotor, blades, casing, and nozzles, as well as the materials used and recent technological advancements.
Major Components of a Steam Turbine
- Rotor
- The rotor is the heart of the steam turbine. It is a long shaft onto which turbine blades are mounted and is responsible for transmitting the mechanical energy generated by the rotating blades. The rotor is directly connected to the generator or mechanical system that the turbine drives.
- Rotors are usually made from high-strength steel alloys that can withstand the centrifugal forces created by high-speed rotation. They must also be resistant to thermal expansion and contraction as temperatures fluctuate during operation.
- Blades
- The blades are arguably the most critical components of a steam turbine, as they are responsible for converting the energy from the steam into mechanical rotation. Turbine blades must be carefully designed to handle the high-pressure and high-temperature steam that passes over them.
- Blade Profile and Shape: Blade shape and aerodynamic profile play a significant role in the turbine’s efficiency. Engineers use computational fluid dynamics (CFD) to model steam flow over the blades and optimize their design for maximum energy extraction.
- Materials: Steam turbine blades are typically made from specialized alloys, often based on nickel or chromium, that can endure high temperatures (up to 600°C) and pressures. In some advanced designs, blades are coated with ceramic or thermal barrier coatings to enhance their durability and resistance to corrosion and erosion.
- Fixed and Moving Blades: Turbines typically consist of alternating rows of fixed and moving blades. The fixed blades (stators) redirect the steam flow, while the moving blades (rotors) extract energy from the steam. This alternating arrangement ensures efficient energy transfer from the steam to the turbine rotor.
- Casing
- The casing encloses the turbine and directs steam through the different stages of the turbine. It must withstand both the internal pressure of the steam and the mechanical stresses generated by the rotating rotor and blades.
- Casing materials are usually high-strength steels or cast iron, designed to handle the thermal expansion caused by high operating temperatures. The casing is often lined with insulation to minimize heat loss and maintain operational efficiency.
- Nozzles
- In impulse turbines, nozzles play a crucial role by converting the steam’s thermal energy into kinetic energy. These nozzles are positioned to direct high-velocity steam jets onto the turbine blades. In reaction turbines, nozzles are incorporated into the blade design itself, allowing the steam to expand continuously as it passes through the turbine.
- The design of nozzles involves precision engineering to ensure that steam flow is directed efficiently at the blades, minimizing energy losses.
- Bearings
- Bearings support the rotor and ensure smooth rotation with minimal friction. Since the rotor operates at high speeds, bearings must be carefully designed to handle both radial and axial forces while minimizing vibration. Bearings are typically made from wear-resistant materials such as specialized steel or ceramic composites.
- Turbines may use oil-lubricated bearings or more advanced magnetic bearings, which reduce friction and wear, leading to improved longevity and performance.
- Seals
- Seals are used to prevent steam from escaping at the points where the rotor passes through the casing. This is critical for maintaining efficiency and minimizing energy losses. Seals are designed to withstand high temperatures and pressures, ensuring that steam remains within the turbine’s operating system.
- Common seal designs include labyrinth seals, which consist of a series of ridges and grooves that reduce steam leakage by creating multiple points of resistance to steam flow.
Materials Used in Turbine Construction
The choice of materials is essential in turbine construction, as the components must endure extreme temperatures, high pressures, and corrosive environments. Engineers typically use materials that have a high strength-to-weight ratio, can withstand thermal stress, and resist corrosion and erosion from the steam. Some common materials include:
- Steel Alloys: High-strength steel alloys are commonly used for components such as the rotor and casing. These alloys are designed to handle high pressures and resist fatigue caused by the continuous rotational forces during operation.
- Nickel-based Alloys: Turbine blades and other critical components exposed to high temperatures are often made from nickel-based superalloys. These materials are ideal for maintaining their strength at elevated temperatures, offering excellent resistance to oxidation and creep (the slow deformation of materials under stress).
- Chromium-based Alloys: Chromium-based alloys are also used in turbine blades and other high-temperature areas. These alloys offer excellent corrosion resistance, especially in environments where steam contains impurities or chemicals.
- Ceramic Coatings: Advanced steam turbines may use ceramic coatings on turbine blades to provide additional thermal protection and reduce wear. These coatings help extend the lifespan of the blades, particularly in environments where erosion and corrosion are significant concerns.
Design Considerations for Efficiency and Durability
Steam turbine design focuses on maximizing efficiency while ensuring durability and reliability. Key design considerations include:
- Aerodynamics of Blades
- The aerodynamic profile of turbine blades is critical for efficiency. Engineers optimize blade shapes to minimize energy losses due to friction and turbulence as steam flows over them. Modern turbines use highly specialized blade profiles that ensure smooth, laminar flow to maximize energy transfer from the steam to the rotor.
- Multistage Turbines
- Most industrial steam turbines are multistage systems, where steam passes through several stages of blades before exiting the turbine. In each stage, the steam expands, losing pressure and temperature, and transferring energy to the blades. Multistage designs allow for more efficient energy extraction, particularly when dealing with high-pressure steam.
- Cooling Systems
- In high-temperature applications, blade cooling is essential to maintain efficiency and prevent damage. Some turbines use internal cooling systems that circulate air or coolant through the blades, reducing the temperature and extending their operational life. Blade cooling is particularly important in turbines using superheated steam.
- Vibration and Noise Management
- High-speed turbines are prone to vibration, which can lead to mechanical wear and damage over time. Modern turbine designs incorporate advanced bearing systems and vibration-damping technologies to minimize these effects. Noise reduction is also an important design consideration, especially for turbines operating in urban areas or near sensitive environments.
Advances in Turbine Blade Technology
In recent years, significant advancements in turbine blade technology have led to improvements in both efficiency and durability:
- 3D Printed Blades: Additive manufacturing (3D printing) has enabled the production of more complex and precise blade designs, improving aerodynamic performance and reducing manufacturing costs. 3D-printed blades can be optimized for specific flow patterns, leading to higher efficiency and reduced material waste.
- Advanced Blade Coatings: New thermal barrier coatings and erosion-resistant materials are being developed to protect turbine blades from high temperatures and harsh environments. These coatings reduce wear and corrosion, extending the operational life of the turbine.
- Smart Blades: Some modern turbines incorporate sensors within the blades to monitor temperature, pressure, and vibration in real-time. These “smart blades” provide valuable data that can be used to optimize turbine performance and predict maintenance needs before issues arise.
Operation and Performance of Steam Turbines
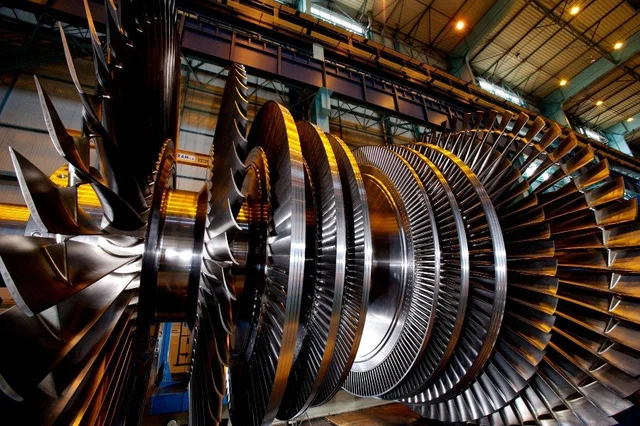
The efficient operation of a steam turbine requires careful management of steam conditions, performance monitoring, and proper maintenance. Steam turbines are designed to operate reliably for extended periods under varying loads and conditions. This section will explore the procedures for starting and stopping turbines, operational efficiency metrics, common issues encountered during operation, and strategies to optimize performance and minimize downtime.
Start-up and Shutdown Procedures
Operating a steam turbine involves specific start-up and shutdown procedures to ensure the turbine functions safely and efficiently. These procedures are designed to manage thermal stress on the turbine’s components, particularly the rotor and blades, which can experience significant temperature changes during these phases.
- Start-up Procedure:
- Pre-Checks: Before starting the turbine, a series of checks are performed to ensure that all systems are operational. This includes checking the lubrication system, ensuring the steam supply is at the correct pressure and temperature, and verifying that all safety interlocks are in place.
- Warming Up: The turbine must be gradually warmed up to avoid thermal shock. Steam is slowly introduced at a reduced pressure and temperature to gradually heat the rotor, blades, and casing. This step is crucial to avoid damage due to rapid temperature changes, which can cause thermal expansion and material fatigue.
- Synchronization: Once the turbine reaches operating speed, it must be synchronized with the electrical grid (in the case of power generation) or the mechanical system it is driving. Synchronization ensures that the turbine’s output matches the grid’s frequency or the system’s load requirements.
- Shutdown Procedure:
- Load Reduction: Before shutting down the turbine, its load is gradually reduced. This allows the system to cool down and prevents excessive mechanical stress on the components.
- Steam Shut-off: After the load is reduced, the steam supply is shut off, and the turbine is allowed to coast down to a stop. The rotor continues to spin for some time after the steam is cut off, so the lubrication system must remain active until the turbine comes to a complete stop.
- Cooling Down: The turbine must be allowed to cool down gradually to avoid thermal stress. This cooling period helps prevent damage to the rotor and blades, which could occur if the turbine is exposed to rapid temperature changes.
Operational Efficiency Metrics
Several key metrics are used to assess the operational efficiency of a steam turbine. These metrics help operators monitor performance and make adjustments to optimize energy conversion and reduce losses.
- Thermal Efficiency:
- Thermal efficiency refers to how effectively a turbine converts the thermal energy of steam into mechanical energy. It is calculated as the ratio of the useful mechanical energy output to the total thermal energy input from the steam. High thermal efficiency is critical in minimizing fuel consumption and reducing operating costs, particularly in power generation applications.
- Factors that affect thermal efficiency include steam pressure, temperature, and quality (i.e., whether the steam is saturated or superheated).
- Heat Rate:
- The heat rate is another key metric used in power generation. It is defined as the amount of energy (typically in British Thermal Units, or BTUs) required to produce one kilowatt-hour (kWh) of electricity. A lower heat rate indicates better turbine performance, as less energy is needed to generate the same amount of electricity.
- Heat rate can be influenced by the turbine’s design, operating conditions, and the quality of the steam. Operators continually monitor heat rate to ensure that the turbine is running efficiently.
- Mechanical Losses:
- Mechanical losses occur due to friction in the bearings, seals, and other moving parts of the turbine. These losses reduce the amount of mechanical energy available for electricity generation or driving industrial equipment. High-quality lubrication systems and well-maintained bearings help minimize these losses.
- Parasitic Losses:
- Parasitic losses are the energy consumed by auxiliary systems that are necessary for the turbine’s operation, such as the lubrication system, pumps, and cooling systems. These losses reduce the overall efficiency of the power plant or industrial process, so minimizing parasitic losses is an important aspect of turbine operation.
Common Issues During Operation
Steam turbines are generally reliable machines, but they can experience operational issues that impact performance and longevity. Some of the most common issues include:
- Corrosion:
- Corrosion can occur when steam contains impurities such as oxygen or other chemicals. Over time, corrosion can weaken turbine components, particularly the blades and casing, leading to reduced efficiency and potential failure.
- To mitigate corrosion, steam is typically treated to remove impurities before it enters the turbine. Additionally, anti-corrosion coatings can be applied to turbine blades and other vulnerable parts.
- Erosion:
- Erosion is caused by solid particles or water droplets in the steam impinging on the turbine blades at high velocity. This can lead to the gradual wearing away of the blades, reducing their aerodynamic efficiency and increasing the risk of failure.
- Erosion is especially common in turbines using saturated steam, which may contain water droplets. Using superheated steam or installing erosion-resistant coatings on the blades can reduce the impact of erosion.
- Steam Quality:
- The quality of steam has a significant impact on turbine performance. Poor-quality steam, which contains water droplets or solid particles, can cause both erosion and corrosion, reducing the turbine’s efficiency and lifespan.
- Maintaining high-quality steam through proper boiler operation, water treatment, and steam conditioning systems is critical to minimizing these risks.
- Vibration and Fatigue:
- Vibration is a common issue in high-speed turbines and can lead to mechanical fatigue over time. Vibration can be caused by imbalances in the rotor, misalignment of bearings, or flow disturbances in the steam path.
- Regular monitoring of vibration levels and the use of advanced bearing systems can help prevent excessive wear and avoid mechanical failures.
Performance Monitoring and Optimization Strategies
To ensure optimal performance, modern steam turbines are equipped with sensors and monitoring systems that provide real-time data on key operating parameters. These systems allow operators to make adjustments and prevent potential issues before they lead to costly downtime.
- Real-Time Monitoring:
- Sensors installed throughout the turbine measure parameters such as steam pressure, temperature, rotor speed, and vibration. This data is analyzed by a control system that automatically adjusts operating conditions to maintain optimal performance.
- Operators can use this data to identify trends and predict potential problems, allowing for proactive maintenance and reducing the risk of unplanned outages.
- Digitalization and Predictive Maintenance:
- Digitalization is playing an increasingly important role in turbine operation and maintenance. Predictive maintenance systems use data from sensors and advanced analytics, including artificial intelligence (AI), to predict when a component is likely to fail. This allows operators to schedule maintenance during planned downtime, reducing the likelihood of unexpected failures.
- AI-driven systems can also help optimize performance by analyzing data trends and recommending adjustments to steam conditions, load distribution, or operating speeds.
- Regular Maintenance:
- Routine maintenance is essential for keeping steam turbines operating efficiently. Regular inspections of the rotor, blades, bearings, and seals can help identify wear and tear before it leads to significant performance issues.
- Typical maintenance activities include lubricating bearings, inspecting seals for leaks, checking steam quality, and monitoring vibration levels.
Applications in Power Generation
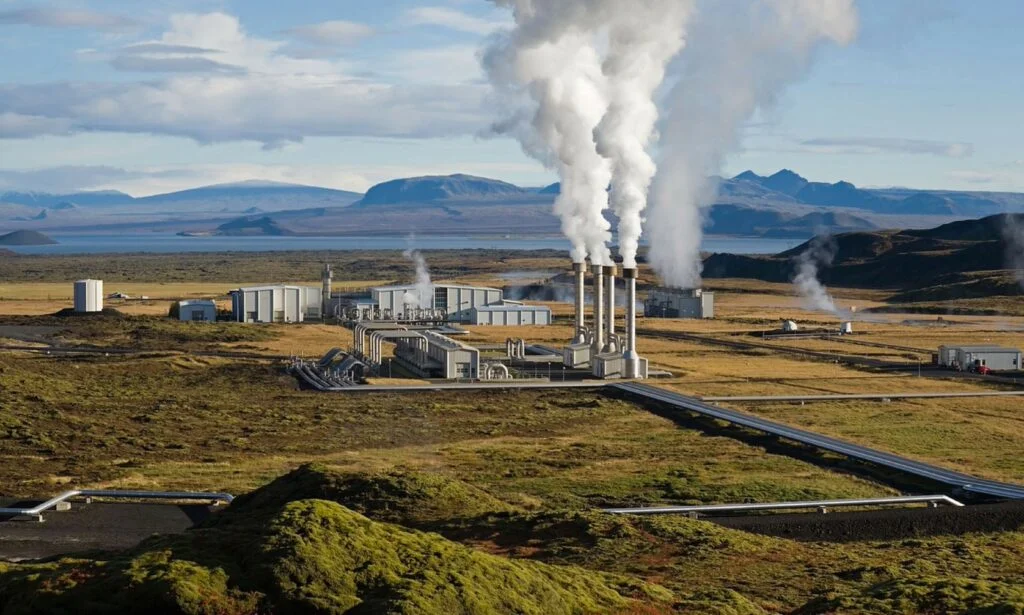
Steam turbines are an essential component of the global power generation industry. Their ability to efficiently convert thermal energy into mechanical and electrical energy makes them a cornerstone of energy production in various types of power plants, from fossil-fuel-based systems to nuclear and renewable energy facilities. This section explores the role of steam turbines in power generation across different fuel sources and technologies, emphasizing their importance in both traditional and modern energy systems.
Steam Turbines in Fossil-Fuel Power Plants
Fossil-fuel power plants—such as those that use coal, natural gas, and oil—are among the most common applications of steam turbines. In these plants, steam turbines are employed to convert the thermal energy generated by burning fossil fuels into electrical energy. Despite the global shift toward cleaner energy sources, fossil-fuel power plants continue to generate a significant portion of the world’s electricity, particularly in regions where access to renewable energy is limited.
- Coal-fired Power Plants:
- In a traditional coal-fired power plant, coal is burned in a boiler to generate heat. This heat is used to convert water into high-pressure steam, which is then directed into the steam turbine. As the steam expands through the turbine, it causes the rotor to spin, generating mechanical energy that is then converted into electricity by a generator.
- Role of Steam Turbines: Steam turbines in coal-fired plants are typically large, multi-stage machines designed to handle high-pressure, superheated steam. Their efficiency is critical to the plant’s overall performance, as coal combustion produces large amounts of thermal energy that must be efficiently converted into electricity.
- Environmental Concerns: Coal-fired power plants are associated with significant environmental issues, including greenhouse gas emissions and air pollution. While steam turbines themselves do not produce emissions, they are integral to the process of converting the energy produced by coal combustion into electricity. Many modern coal plants incorporate pollution control technologies, such as carbon capture and storage (CCS), to mitigate their environmental impact.
- Natural Gas-fired Power Plants:
- Natural gas is used in two main types of power plants: simple-cycle gas plants and combined-cycle gas plants. In a simple-cycle plant, natural gas is burned in a combustion chamber, and the high-temperature exhaust gases are used to spin a gas turbine. In a combined-cycle plant, the exhaust heat from the gas turbine is used to generate steam, which is then directed into a steam turbine to produce additional electricity. This combination increases the overall efficiency of the power plant.
- Role of Steam Turbines in Combined-Cycle Plants: In combined-cycle gas plants, steam turbines play a crucial role in utilizing the waste heat from the gas turbine, making the overall system more efficient. This process can increase the plant’s efficiency from around 30-40% (in simple-cycle operation) to more than 60% in combined-cycle mode.
- Environmental Benefits: Compared to coal, natural gas produces fewer emissions of carbon dioxide (CO₂), sulfur dioxide (SO₂), and particulate matter. Combined-cycle plants, in particular, are considered one of the most efficient and environmentally friendly fossil-fuel-based power generation technologies.
- Oil-fired Power Plants:
- Oil-fired power plants operate similarly to coal-fired plants, with oil being burned in a boiler to produce steam, which drives the steam turbine. These plants are less common today due to the higher cost of oil compared to coal and natural gas, as well as environmental concerns.
- Applications: Oil-fired plants are often used in regions where natural gas and coal are less accessible, or as backup power sources in places like remote islands or industrial facilities.
Role of Steam Turbines in Nuclear Power Plants
Nuclear power plants rely on steam turbines to convert the thermal energy produced by nuclear fission into electricity. These plants are designed to operate continuously at high output levels, making steam turbines an essential component of their overall efficiency and reliability.
- Pressurized Water Reactors (PWRs):
- In pressurized water reactors (PWRs), nuclear fission takes place in the reactor core, generating heat that is used to produce high-pressure steam. The steam is then routed to the steam turbine, where it expands and spins the turbine rotor, generating mechanical energy that is converted into electricity.
- Steam Turbines in PWRs: In PWR systems, the steam that drives the turbine is typically superheated, allowing for greater efficiency in energy conversion. The steam turbine is designed to handle the specific conditions of nuclear power plants, including high reliability and resistance to radiation.
- Boiling Water Reactors (BWRs):
- In a boiling water reactor (BWR), water is heated directly by the nuclear reactor to produce steam, which is sent directly to the steam turbine. BWRs use the steam generated within the reactor itself, eliminating the need for a separate steam generator.
- Challenges in BWRs: Since the steam that drives the turbine comes directly from the reactor, the turbine must be designed to handle potential contamination from radioactive materials. Special materials and design considerations are required to ensure safe and reliable operation.
- Advantages of Nuclear Steam Turbines:
- High Capacity: Steam turbines used in nuclear power plants are often among the largest in the world, with the ability to generate several hundred megawatts of electricity. Their size and capacity are crucial for meeting the base-load power requirements of many countries.
- Environmental Benefits: Nuclear power is a low-carbon energy source, making it an attractive option for reducing greenhouse gas emissions. Steam turbines play a vital role in the efficiency of nuclear plants, ensuring that the thermal energy produced by nuclear reactions is effectively converted into electricity.
Steam Turbines in Renewable Energy: Biomass and Geothermal Plants
In addition to fossil fuels and nuclear power, steam turbines are also used in renewable energy applications, particularly in biomass and geothermal power plants. These renewable energy sources offer a cleaner alternative to traditional power generation, and steam turbines help maximize their efficiency.
- Biomass Power Plants:
- Biomass power plants use organic materials such as wood, agricultural waste, or dedicated energy crops to generate heat. The combustion of biomass produces steam, which is then directed into a steam turbine to generate electricity.
- Advantages of Steam Turbines in Biomass Plants:
- Biomass is considered carbon-neutral, as the CO₂ released during combustion is offset by the CO₂ absorbed during the growth of the biomass. This makes biomass plants an environmentally friendly alternative to fossil-fuel plants.
- Steam turbines in biomass plants are similar to those used in fossil-fuel plants, with modifications to accommodate the lower energy content of biomass fuel compared to coal or gas.
- Geothermal Power Plants:
- Geothermal power plants harness the heat stored beneath the Earth’s surface to generate steam, which drives steam turbines to produce electricity. In these plants, geothermal reservoirs provide naturally occurring steam or hot water, which is converted into mechanical energy by the turbine.
- Types of Geothermal Plants:
- Dry Steam Plants: These plants use steam directly from geothermal wells to drive the turbine.
- Flash Steam Plants: In flash steam plants, hot water from geothermal reservoirs is depressurized to produce steam, which is then used in the turbine.
- Binary Cycle Plants: In binary cycle plants, geothermal water heats a secondary fluid with a lower boiling point, which vaporizes and drives the turbine.
- Advantages of Geothermal Steam Turbines:
- Geothermal power is a highly reliable and continuous energy source, as geothermal heat is available 24/7. Steam turbines in geothermal plants operate with high reliability and can be integrated with binary systems for greater efficiency.
Combined-Cycle Power Plants: Integration of Steam and Gas Turbines
Combined-cycle power plants are one of the most efficient forms of power generation, utilizing both gas turbines and steam turbines to maximize energy output. In these plants, the exhaust heat from a gas turbine is used to generate steam, which is then directed into a steam turbine to produce additional electricity. This dual-turbine approach significantly increases the plant’s overall efficiency, often exceeding 60%.
- Operation: In a combined-cycle plant, natural gas is burned in a combustion chamber, driving a gas turbine. The high-temperature exhaust gases are used to heat water in a heat recovery steam generator (HRSG), producing steam. The steam is then sent to a steam turbine, where it generates additional electricity.
- Advantages of Combined-Cycle Plants:
- Higher Efficiency: By utilizing both gas and steam turbines, combined-cycle plants achieve much higher efficiency than traditional single-cycle power plants.
- Lower Emissions: Combined-cycle plants produce fewer emissions compared to coal or oil plants due to their higher efficiency and the cleaner nature of natural gas as a fuel.
- Flexibility: These plants can respond quickly to changes in electricity demand, making them ideal for balancing grid load, especially in regions with a high share of intermittent renewable energy sources like wind or solar.
Economic and Environmental Considerations
Steam turbines are central to the economic and environmental performance of power plants. Their efficiency directly influences the cost of electricity generation and the plant’s environmental footprint.
- Efficiency and Cost:
- Higher efficiency turbines reduce fuel consumption, which lowers operating costs and reduces the plant’s overall environmental impact. For example, improving the thermal efficiency of a coal-fired power plant by just a few percentage points can lead to significant reductions in fuel use and greenhouse gas emissions.
- Environmental Impact:
- The environmental impact of steam turbines depends largely on the energy source they are paired with. In fossil-fuel plants, steam turbines play a role in emissions management through the efficient conversion of thermal energy into electricity. In nuclear and renewable plants, they help reduce greenhouse gas emissions by providing a reliable, low-carbon energy source.
Industrial Applications Beyond Power Generation
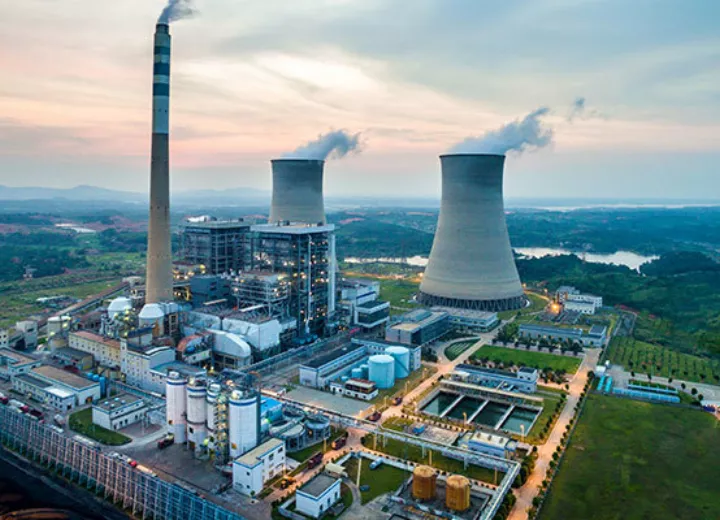
While steam turbines are commonly associated with power generation, their use extends far beyond electricity production. Steam turbines play a vital role in various industrial applications, where they provide both mechanical power and heat for industrial processes. In many industries, steam turbines are integrated into systems that produce both electricity and useful thermal energy in what is known as cogeneration or combined heat and power (CHP) systems. This section explores the critical role of steam turbines in industrial sectors such as petrochemical refining, pulp and paper manufacturing, food processing, and desalination.
Cogeneration and District Heating
Cogeneration, also known as combined heat and power (CHP), is a highly efficient system that generates both electricity and useful heat from the same energy source. Steam turbines are often at the heart of cogeneration systems, where they not only produce electricity but also provide thermal energy for industrial processes or heating applications. This dual-purpose use of energy improves overall efficiency and reduces waste.
- Cogeneration Systems:
- In a typical cogeneration system, steam is generated in a boiler, and a portion of the steam is used to drive a steam turbine and generate electricity. The remaining steam, instead of being condensed and wasted, is extracted at an intermediate pressure and used for industrial processes, heating, or cooling.
- Advantages:
- Higher Efficiency: By utilizing the waste heat from electricity generation, cogeneration systems can achieve efficiencies of 70-90%, compared to the 30-40% efficiency of conventional power plants.
- Cost Savings: Cogeneration reduces fuel costs by producing both electricity and heat from a single energy source.
- Environmental Benefits: Since cogeneration uses less fuel to produce the same amount of energy, it results in lower emissions of carbon dioxide (CO₂) and other pollutants.
- District Heating:
- Steam turbines are also used in district heating systems, where the heat produced during electricity generation is used to supply hot water or steam for heating buildings in urban areas. This is particularly common in cold climates, where district heating systems provide an efficient way to heat homes, offices, and industrial facilities.
- Applications: District heating systems are widespread in countries like Denmark, Sweden, and Russia, where they contribute to significant energy savings and reduce the need for individual heating systems in each building.
Steam Turbines in the Petrochemical and Refining Industries
The petrochemical and refining industries are major users of steam turbines, where they are employed to drive mechanical equipment, such as compressors, pumps, and fans, as well as to generate electricity as part of cogeneration systems.
- Mechanical Drive Applications:
- In many petrochemical plants and refineries, steam turbines are used to provide mechanical power for large compressors and pumps. These machines are essential for processes such as refining crude oil, compressing natural gas, and producing chemicals.
- Advantages:
- Reliability: Steam turbines provide continuous, reliable mechanical power, making them ideal for processes that must operate 24/7 without interruption.
- Fuel Flexibility: Steam turbines in these industries can use the heat generated from waste products or by-products, such as process gas, to produce steam, enhancing overall efficiency.
- Cogeneration in Refineries:
- Refineries often use cogeneration systems to produce both electricity and steam for their internal processes. For example, steam is used for distillation processes, cracking, and other heat-intensive operations, while electricity generated by the turbines powers the plant’s equipment.
- Example: In a typical refinery, steam turbines may drive large compressors that help separate and refine different hydrocarbon fractions from crude oil.
Pulp and Paper Manufacturing
The pulp and paper industry is another major user of steam turbines, where they are integrated into combined heat and power systems to provide the electricity and steam required for paper production. This industry relies heavily on steam for drying paper products and for mechanical processes such as pulping.
- Cogeneration in Pulp and Paper Mills:
- Pulp and paper mills use cogeneration to generate electricity and heat from the same steam. The steam is used to drive turbines for power generation, while the remaining steam is directed into industrial processes such as drying, cooking, and heating.
- Process: Biomass or waste wood from the paper production process is often used as fuel to generate steam, making this a highly sustainable approach. The steam drives a turbine to generate electricity, and the residual steam is used for drying paper and other manufacturing steps.
- Efficiency: Steam turbines help paper mills achieve high energy efficiency by making use of both electricity and heat. This reduces the need for external energy sources, lowering costs and minimizing the mill’s carbon footprint.
- Mechanical Drive:
- Steam turbines in pulp and paper plants are also used to drive mechanical equipment such as pumps, fans, and compressors, which are essential for the production process.
Steam Turbines in the Food Processing Industry
The food processing industry uses steam turbines in various ways, primarily to drive mechanical equipment and provide heat for cooking, sterilization, and drying processes. The ability to generate both electricity and steam from a single energy source makes steam turbines ideal for food processing plants.
- Sterilization and Cooking:
- Steam is used extensively in food processing for sterilizing equipment, pasteurizing products, and cooking food items in industrial-scale operations. The steam needed for these processes can be produced as a by-product of electricity generation in a cogeneration system.
- Example: A food processing plant may use a steam turbine to generate electricity while utilizing the waste steam for cooking and sterilization processes, optimizing fuel usage.
- Energy Efficiency:
- Similar to other industries, the food processing industry benefits from the high energy efficiency of cogeneration systems. By capturing the heat produced during electricity generation, food processors can significantly reduce their energy costs and lower their environmental impact.
Desalination Plants
Steam turbines are also used in desalination plants, which convert seawater into fresh water. Desalination is an energy-intensive process, and steam turbines provide an efficient solution for both electricity generation and mechanical drive applications within these plants.
- Thermal Desalination Processes:
- Thermal desalination plants, such as multi-stage flash (MSF) desalination, rely on steam to heat seawater and evaporate it. The steam is produced by a boiler or recovered from a cogeneration system, and the heat is used to evaporate seawater, leaving behind salt and other impurities.
- Role of Steam Turbines: Steam turbines in desalination plants are used to generate electricity and drive the pumps and compressors that are essential for the desalination process. By integrating steam turbines into the plant’s energy system, desalination facilities can achieve higher efficiencies and reduce operating costs.
- Cogeneration and Desalination:
- Many desalination plants use cogeneration systems, where the steam turbine generates both electricity and the heat needed for the desalination process. This reduces the overall energy consumption of the plant and makes desalination more economically viable, especially in regions with limited freshwater resources.
Other Industrial Applications
Steam turbines are also used in various other industries, including:
- Chemical Manufacturing:
- In chemical manufacturing plants, steam turbines provide mechanical power and process heat for chemical reactions, distillation, and other heat-intensive processes. Cogeneration is often used to improve energy efficiency in chemical plants.
- Steel and Cement Production:
- Steel and cement plants use steam turbines to generate electricity and drive mechanical equipment. Waste heat from the production process is often recovered to generate steam, making these plants more energy-efficient.
Efficiency and Performance Improvements
Improving the efficiency and performance of steam turbines has been a focal point of research and development in the energy and industrial sectors. Increased efficiency means extracting more useful energy from the same amount of fuel, reducing operating costs, and minimizing environmental impact. Technological advancements, materials innovation, and better operational practices have all contributed to significant improvements in the performance of steam turbines. This section explores the various methods and technologies that have enhanced turbine efficiency, including advanced blade design, digitalization, turbine cooling, steam quality optimization, and retrofitting older turbines.
Advanced Blade and Turbine Design
The design of turbine blades is critical to the overall efficiency of a steam turbine. Blades are responsible for converting steam energy into mechanical work, and their shape, material, and aerodynamic properties significantly influence turbine performance. Recent advances in blade design and materials science have led to more efficient turbines that can operate at higher temperatures and pressures.
- Aerodynamic Blade Profiles:
- Modern steam turbines use advanced blade profiles that are optimized for the smooth, laminar flow of steam. Engineers use computational fluid dynamics (CFD) simulations to design blade shapes that minimize turbulence and energy loss as steam passes through the turbine stages.
- Three-dimensional (3D) Blades: In traditional turbine designs, blades were mostly two-dimensional. However, modern turbines employ 3D blades that have complex curvature and variable angles of attack. These 3D blades improve steam flow control, reducing secondary losses (caused by steam spilling over the edges of blades) and improving the efficiency of energy transfer.
- Longer and More Efficient Blades:
- Advances in materials and manufacturing technologies have allowed for the creation of longer turbine blades that can operate at higher temperatures and rotational speeds. Longer blades increase the surface area for steam interaction, allowing for greater energy extraction per stage.
- Last-stage Blades: In low-pressure turbine sections, the last-stage blades are particularly important for maximizing efficiency. Longer, highly efficient last-stage blades help capture more energy from the exhaust steam, reducing energy losses and improving overall turbine performance.
- Advanced Materials:
- The development of high-temperature, corrosion-resistant materials has also contributed to improvements in turbine efficiency. Steam turbine blades are now commonly made from nickel-based superalloys or titanium alloys, which can withstand extreme temperatures and high levels of stress without degrading.
- Protective Coatings: Blade coatings, such as ceramic thermal barrier coatings (TBCs) and erosion-resistant coatings, provide additional protection against the harsh environment inside the turbine. These coatings reduce wear and extend the operational life of the blades, maintaining high performance over longer periods.
Turbine Cooling and Steam Quality Optimization
High temperatures improve the efficiency of steam turbines, as higher thermal energy in the steam allows for more effective energy conversion. However, these temperatures can also place enormous stress on turbine components. Cooling technologies and steam quality management play an essential role in maintaining efficiency while preventing damage to the turbine.
- Internal Blade Cooling:
- In high-efficiency turbines, especially those using superheated steam, blade cooling systems are essential for preventing thermal damage. Internal cooling systems circulate coolant (often air or steam) through channels within the turbine blades, reducing the temperature of the blade surfaces while allowing the turbine to operate at higher steam temperatures.
- Innovations in Cooling Technology: Advances in cooling technology, including the use of more efficient cooling channels and coatings, have enabled steam turbines to handle increasingly higher inlet steam temperatures. This helps improve the thermal efficiency of the turbine while ensuring long-term reliability.
- Optimizing Steam Quality:
- The quality of the steam used in a turbine significantly impacts its efficiency and longevity. Superheated steam is preferred in power generation applications due to its higher energy content and the absence of moisture, which can cause blade erosion.
- Moisture Control: Moisture in the steam can reduce efficiency by increasing energy losses through condensation and causing damage to the turbine blades. Advanced steam separators and reheaters are used in modern turbines to ensure that only dry, high-quality steam enters the turbine stages.
- Reheat Cycles: Some turbines employ a reheat cycle, where steam is partially expanded in the turbine, then sent back to the boiler to be reheated before continuing through the turbine. Reheating increases the steam’s temperature and energy content, leading to greater efficiency.
Role of Digitalization and AI in Performance Monitoring
The digitalization of steam turbine systems has enabled operators to continuously monitor turbine performance, optimize operations in real-time, and predict maintenance needs. Digital tools, sensors, and advanced analytics are revolutionizing the way turbines are managed, leading to significant improvements in both efficiency and reliability.
- Real-Time Monitoring and Data Analytics:
- Modern steam turbines are equipped with a range of sensors that measure key parameters such as temperature, pressure, rotor speed, vibration, and steam flow. These sensors feed data into advanced analytics systems, which provide operators with real-time insights into turbine performance.
- Predictive Analytics: By analyzing historical data and trends, predictive analytics systems can identify potential performance issues before they lead to failures. This allows operators to schedule maintenance during planned downtime, reducing the risk of unplanned outages and optimizing turbine efficiency.
- AI-driven Performance Optimization:
- Artificial intelligence (AI) systems are increasingly being used to optimize turbine operations. AI algorithms can analyze large amounts of performance data, identify inefficiencies, and recommend adjustments to operating conditions, such as steam pressure, load distribution, or turbine speed.
- Digital Twins: One emerging technology is the use of digital twins—virtual models of steam turbines that simulate real-world operating conditions. Digital twins allow engineers to test different scenarios, optimize turbine designs, and predict how the turbine will respond to varying operating conditions, all without disrupting the actual system.
- Maintenance and Condition Monitoring:
- Predictive maintenance systems use digital tools and AI to monitor the condition of critical turbine components, such as blades, bearings, and seals. These systems can detect signs of wear, corrosion, or misalignment, enabling timely maintenance and preventing efficiency losses due to degraded components.
- Vibration Monitoring: Vibration is a common issue in high-speed turbines, and excessive vibration can lead to mechanical fatigue and failure. Digital monitoring systems continuously track vibration levels, allowing operators to take corrective actions before serious damage occurs.
Efficiency vs. Cost: Economic Trade-offs
While increasing efficiency is a major goal for turbine operators, there are often economic trade-offs between achieving maximum efficiency and managing costs. Turbine upgrades, retrofits, and advanced materials can significantly improve performance, but they also come with associated costs. Operators must balance the investment in new technologies with the long-term savings in fuel costs and maintenance.
- Upgrading to Higher-efficiency Designs:
- Upgrading older turbines with more efficient blades, advanced materials, and digital monitoring systems can yield substantial efficiency gains. However, these upgrades require significant capital investment, and operators must carefully evaluate the return on investment (ROI) before proceeding.
- Cost-benefit Analysis: A thorough cost-benefit analysis is essential when considering upgrades or retrofits. The long-term benefits of improved efficiency, reduced fuel consumption, and extended equipment life must be weighed against the upfront costs of new components and installation.
- Balancing Efficiency and Reliability:
- In some cases, maximizing efficiency may not be the best approach if it compromises reliability or increases the risk of component failure. For example, pushing turbines to operate at their maximum thermal limits may result in higher efficiency, but it could also reduce the lifespan of critical components such as blades and seals.
- Optimized Operating Points: Many turbine operators use digital tools to find the optimal operating point, where the turbine achieves the best balance between efficiency, reliability, and operational costs.
Retrofitting and Upgrading Older Turbines
As the global energy industry evolves, many operators are retrofitting older steam turbines with modern technology to improve performance and extend their operational life. Retrofitting is a cost-effective way to enhance turbine efficiency without completely replacing existing systems.
- Retrofitting for Efficiency Gains:
- Common retrofit solutions include upgrading blades to more aerodynamic designs, installing more efficient bearings, and integrating modern control systems. These upgrades can improve the turbine’s heat rate, reduce parasitic losses, and increase overall energy output.
- Steam Path Upgrades: Retrofitting the steam path, which includes the nozzles, blades, and seals, can significantly reduce energy losses and improve the turbine’s capacity to handle higher pressures and temperatures.
- Integration with Digital Monitoring Systems:
- Older turbines can also be retrofitted with digital monitoring and control systems, enabling real-time performance tracking and predictive maintenance capabilities. These systems improve operational efficiency by identifying inefficiencies and preventing costly downtime.
Basic Principles of Industrial Steam Turbines
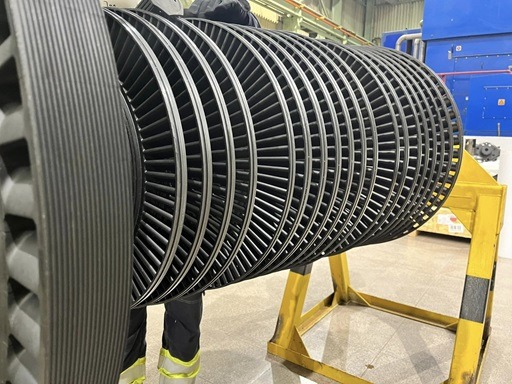
Steam turbines are mechanical devices that convert the thermal energy of steam into mechanical work, which can then be used to generate electricity or power industrial equipment. Since their invention in the late 19th century, steam turbines have become essential components of modern energy infrastructure, playing a vital role in power generation, petrochemical refining, and other industries.
The development of the steam turbine marked a significant leap forward from early steam engines, which were inefficient and bulky. Sir Charles Parsons and Gustaf de Laval were pioneers in developing the first practical steam turbines, which revolutionized industries by providing more efficient energy conversion mechanisms.
Steam turbines are commonly found in power plants—both fossil-fuel and nuclear—and in various industrial applications such as district heating, cogeneration, and mechanical drive systems for compressors and pumps. Their ability to operate efficiently under high-pressure and high-temperature conditions makes them indispensable for converting heat energy into mechanical power on an industrial scale.
Thermodynamic Principles
At the core of steam turbine operation is thermodynamics, particularly the Rankine cycle. The Rankine cycle is a thermodynamic process that describes how heat is transformed into work in a steam turbine system. This cycle typically includes four stages:
- Heat Addition (Boiler): Water is heated to form steam in a boiler at constant pressure.
- Expansion (Turbine): The high-pressure steam expands through the turbine, converting its thermal energy into mechanical work.
- Condensation (Condenser): The expanded steam is cooled in a condenser, returning to a liquid state.
- Pressurization (Pump): The condensed water is then pumped back to the boiler to begin the cycle again.
In steam turbines, energy conversion is based on the principles of entropy and enthalpy. As steam expands within the turbine, its entropy increases while its enthalpy decreases. This drop in enthalpy is what drives the turbine rotor, producing mechanical energy.
Understanding the thermodynamic principles behind steam turbines is crucial for optimizing their performance. Concepts such as isentropic efficiency, enthalpy drop, and steam enthalpy diagrams (Mollier diagrams) help engineers design turbines that maximize energy conversion while minimizing losses.
Steam Properties and Their Role in Turbine Efficiency
The properties of the steam used in a turbine—such as pressure, temperature, and steam quality—have a direct impact on turbine efficiency. The two main types of steam used in turbines are:
- Saturated Steam: Steam that is at the boiling point for its pressure. It contains both liquid and vapor phases.
- Superheated Steam: Steam heated beyond its boiling point at a given pressure, making it dry and containing no water droplets.
Superheated steam is typically used in industrial steam turbines due to its higher energy content and efficiency. The temperature and pressure of the steam entering the turbine determine the amount of work that can be extracted.
The quality of steam is also important, particularly when considering the presence of moisture in the steam. Wet steam can cause erosion of turbine blades and reduce efficiency. In high-efficiency systems, steam reheat and moisture separators are used to maintain high steam quality and prevent damage to the turbine components.
Types of Industrial Steam Turbines
Steam turbines are classified based on their working principles and applications. The two primary types are:
- Impulse Turbines:
- In impulse turbines, high-pressure steam is directed through nozzles, converting thermal energy into kinetic energy. This high-velocity steam jet impacts the turbine blades, causing the rotor to spin.
- Impulse turbines maintain constant pressure as steam flows through the turbine, while the velocity decreases.
- Reaction Turbines:
- In reaction turbines, the steam expands continuously as it flows over the blades. Both the moving blades and stationary blades serve as nozzles, allowing the steam to drop in pressure as it progresses through the turbine stages.
- Reaction turbines extract energy by utilizing both steam pressure and velocity changes across the blades.
Steam turbines are further classified by their applications, including condensing turbines (which maximize energy extraction by condensing exhaust steam) and non-condensing turbines (used in industrial processes where the steam is needed for heating after expansion). Extraction turbines allow steam to be extracted at various pressure levels for industrial processes, while back-pressure turbines exhaust steam at higher pressures for further use.
Components of a Steam Turbine
Steam turbines consist of several key components, each designed to withstand high pressures, temperatures, and rotational forces:
- Rotor:
- The rotor is a shaft that carries the turbine blades and is the main rotating part of the turbine. It converts the energy from steam into mechanical work. Rotors are usually made from high-strength alloys that can endure high thermal and mechanical stresses.
- Blades:
- Blades are mounted on the rotor and play a key role in converting steam energy into rotational motion. They come in various shapes and sizes, depending on the type of turbine and the stage of energy extraction.
- Blade Materials: Turbine blades are typically made of specialized alloys that can withstand high temperatures and pressures. Materials like nickel-based alloys or titanium are often used, with thermal coatings to prevent corrosion and erosion.
- Casings and Nozzles:
- The casing encloses the turbine, guiding steam flow and providing structural integrity. Nozzles are used to direct steam onto the blades, particularly in impulse turbines, where they convert steam pressure into velocity.
- Seals and Bearings:
- Seals prevent steam leakage, while bearings support the rotor and allow it to spin smoothly. These components are critical for ensuring efficient operation and minimizing energy losses.
- Control Systems:
- Modern steam turbines are equipped with advanced control systems that regulate steam flow, rotor speed, and load distribution. Governors play a key role in maintaining operational stability by adjusting the steam supply based on load demand.
Working Stages of a Steam Turbine
Industrial steam turbines are typically multi-stage machines, where steam passes through multiple sets of blades to extract energy more efficiently. The stages are divided into:
- High-Pressure (HP) Stage: Where steam enters at its highest pressure and temperature, delivering the maximum energy.
- Intermediate-Pressure (IP) Stage: Steam expands further, losing pressure but still containing significant energy for conversion.
- Low-Pressure (LP) Stage: By the time steam reaches this stage, its pressure and temperature are lower, but the remaining energy is extracted to maximize efficiency.
Each stage is designed to handle specific steam conditions, optimizing the energy conversion process. Blade staging ensures that energy is extracted gradually, preventing sudden drops in pressure that could damage the turbine or reduce efficiency.
Energy Conversion Efficiency
The efficiency of a steam turbine is determined by how well it converts the thermal energy in steam into mechanical work. Key factors influencing turbine efficiency include:
- Thermal Efficiency:
- The thermal efficiency of a steam turbine measures how effectively it converts heat into mechanical work. A highly efficient turbine will extract as much energy as possible from the steam before exhausting it.
- Heat Rate:
- Heat rate is a critical metric used to assess a power plant’s efficiency. It refers to the amount of fuel energy required to generate one unit of electricity. Lower heat rates indicate higher efficiency.
- Reheat Cycles:
- In a reheat cycle, steam is partially expanded in the turbine and then returned to the boiler to be reheated. This increases the efficiency of the cycle by allowing the turbine to extract more energy from the steam at a higher temperature.
- Mechanical Losses:
- Losses due to friction in bearings, seals, and other moving parts reduce the overall efficiency of the turbine. Minimizing these mechanical losses is key to maintaining high performance.
Steam Flow Dynamics
The flow of steam through a turbine is governed by complex fluid dynamics. Steam behaves differently depending on its velocity, pressure, and temperature, and the design of the turbine blades and nozzles plays a key role in managing these variables.
- Laminar vs. Turbulent Flow:
- Laminar flow refers to smooth, orderly steam movement, while turbulent flow is chaotic and less efficient. Engineers design turbines to maintain laminar flow as much as possible, reducing energy losses due to turbulence.
- Steam Speed and Pressure Gradients:
- As steam expands in the turbine, its speed increases while its pressure drops. The design of turbine blades ensures that these changes are controlled, allowing the steam to transfer its energy effectively to the rotor.
Operation and Maintenance of Steam Turbines
Efficient operation and proper maintenance are essential for the long-term performance of steam turbines. Key operational practices include:
- Start-up and Shutdown Procedures:
- Steam turbines must be carefully started and shut down to prevent thermal shock and mechanical stress. Gradually introducing steam at controlled temperatures and pressures helps maintain the turbine’s integrity.
- Common Operational Issues:
- Vibration: High-speed rotation can cause vibration, leading to mechanical wear and reduced efficiency. Advanced bearings and vibration monitoring systems are used to mitigate this issue.
- Corrosion and Erosion: Steam impurities can cause corrosion and erosion of turbine blades, reducing their lifespan and performance. Water treatment and anti-corrosion coatings are used to prevent this.
- Predictive Maintenance:
- Modern turbines are equipped with sensors that monitor performance in real-time. Predictive maintenance systems use this data to predict when components are likely to fail, allowing operators to perform maintenance before a breakdown occurs.
Future Trends in Steam Turbine Technology
As the energy industry evolves, so too do steam turbine technologies. Key trends include:
- Advanced Blade Materials:
- The development of new alloys and coatings is enabling turbines to operate at higher temperatures and pressures, improving efficiency.
- Digitalization and AI:
- The integration of digital tools, such as AI-driven performance optimization and digital twins, is enhancing turbine efficiency by allowing operators to monitor and adjust operations in real-time.
- Sustainability:
- Steam turbines are being integrated into renewable energy systems, such as biomass and geothermal plants, and are playing a role in carbon capture and storage (CCS) efforts, making them more relevant in the transition to cleaner energy sources.
Conclusion
Steam turbines have been, and will continue to be, fundamental to industrial energy conversion. Their principles of operation, based on thermodynamics and fluid dynamics, are essential for understanding how heat energy is transformed into mechanical work.
As industries seek to improve energy efficiency and reduce emissions, steam turbines will remain a critical technology, supported by advancements in materials science, digital monitoring, and sustainability initiatives.
Introduction to Industrial Steam Turbines
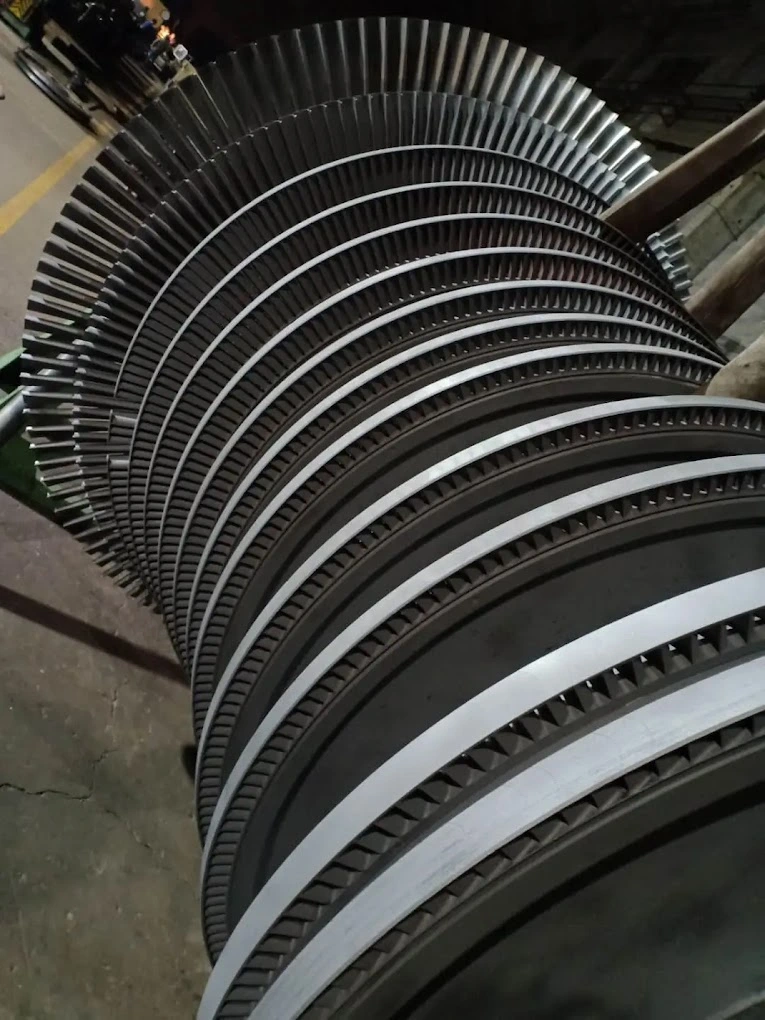
Industrial steam turbines are among the most critical components in energy conversion systems, used primarily for generating electricity and providing mechanical power in various industries. Their ability to efficiently transform thermal energy from steam into mechanical work makes them indispensable in a wide range of industrial applications, from power generation to petrochemical refining and beyond.
A steam turbine works by using high-pressure steam to rotate a series of blades mounted on a shaft (the rotor). As steam passes through the turbine, its energy is transferred to the blades, causing the rotor to spin. This rotational energy can then be used to drive an electrical generator or mechanical equipment. The fundamental principle behind steam turbines involves the conversion of the potential energy in steam into kinetic energy, and then into mechanical work.
Historical Development of Steam Turbines
The evolution of the steam turbine dates back to the late 19th century, with significant contributions from engineers like Sir Charles Parsons and Gustaf de Laval. Prior to their inventions, steam engines were the dominant technology for converting thermal energy into mechanical work. However, steam engines, while revolutionary for their time, had several limitations: they were inefficient, bulky, and required substantial amounts of fuel to operate.
Sir Charles Parsons is credited with the invention of the modern steam turbine in 1884. His design was a breakthrough in terms of efficiency and compactness, and it quickly replaced steam engines in many applications. Parsons’ turbine was initially designed for generating electricity, and it played a pivotal role in powering early electrical grids. His invention made it possible to generate electricity on a larger and more efficient scale, laying the foundation for the widespread adoption of steam turbines in power generation.
Gustaf de Laval, another pioneering engineer, developed the impulse turbine, which operates by directing high-speed jets of steam at a set of blades mounted on the rotor. This design allowed turbines to operate at much higher rotational speeds than earlier designs, improving efficiency and power output. De Laval’s innovations contributed to the development of turbines that could handle higher pressure and temperature steam, making them more suitable for industrial applications.
Throughout the 20th century, steam turbines became more sophisticated, with improvements in materials, design, and operational techniques. The introduction of superheated steam, which increases the thermal efficiency of the turbine, was a significant advancement. Additionally, the development of multi-stage turbines allowed for more efficient energy extraction, making steam turbines essential in industries where high energy output is required.
Importance of Steam Turbines in Industry
Today, steam turbines are used in a variety of industries, with their primary application being in power generation. In thermal power plants, steam turbines are used to convert the thermal energy produced by burning fossil fuels, such as coal, oil, or natural gas, into mechanical energy, which is then converted into electricity. In nuclear power plants, steam turbines are used to convert the heat generated by nuclear fission into electrical energy.
Beyond power generation, steam turbines play a critical role in industrial processes. In the petrochemical industry, for example, they are used to drive compressors, pumps, and other mechanical equipment necessary for refining crude oil and producing chemicals. In the pulp and paper industry, steam turbines are used in cogeneration systems, where they generate both electricity and steam for industrial processes.
Steam turbines are also used in combined heat and power (CHP) systems, where they simultaneously produce electricity and useful thermal energy. These systems are particularly efficient because they make use of the waste heat generated during electricity production, rather than letting it dissipate unused. In industrial plants, steam turbines can be used to drive mechanical processes while also providing steam for heating, drying, or chemical reactions.
The versatility of steam turbines makes them suitable for a wide range of applications, including:
- Electric Power Generation: In fossil-fuel, nuclear, and renewable energy plants.
- Cogeneration (CHP): Producing both electricity and heat for industrial processes or district heating systems.
- Mechanical Drive: Providing mechanical power for equipment such as compressors, pumps, and fans in industries like oil refining, chemical manufacturing, and steel production.
- Renewable Energy: Steam turbines are used in geothermal power plants, biomass plants, and solar thermal plants, where they convert renewable heat sources into electricity.
Current and Future Relevance
With global energy demands continuing to rise, steam turbines remain vital for producing large-scale electricity. Despite the shift toward renewable energy sources, steam turbines are still a key component in both conventional and renewable power plants. In combined-cycle power plants, which integrate gas turbines and steam turbines, the exhaust heat from a gas turbine is used to generate steam, which is then directed into a steam turbine. This configuration is one of the most efficient ways to generate electricity, often achieving efficiencies of over 60%.
Steam turbines also play an important role in the transition to cleaner energy systems. In renewable energy applications, such as geothermal and biomass power plants, steam turbines convert heat from sustainable sources into electricity. Additionally, carbon capture and storage (CCS) technologies, which aim to reduce the carbon emissions from fossil-fuel power plants, are being integrated into steam turbine systems to make energy generation more sustainable.
The future of steam turbines will likely involve further integration with digital technologies, such as artificial intelligence (AI) and Internet of Things (IoT) sensors, which allow for real-time monitoring and optimization of turbine performance. These technologies will help increase operational efficiency, reduce downtime, and improve maintenance practices, ensuring that steam turbines remain a reliable and cost-effective solution for industrial energy needs.
Thermodynamic Principles
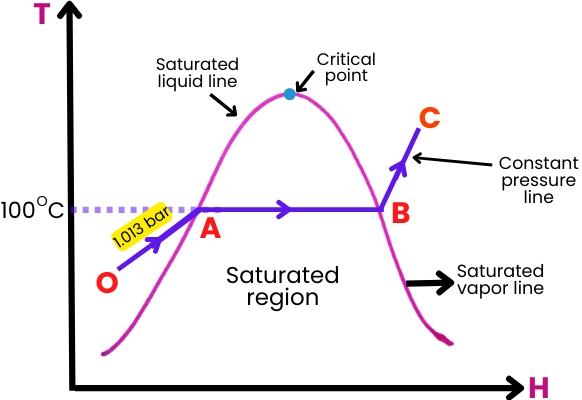
At the heart of steam turbine operation lies thermodynamics—the branch of physics that deals with the relationship between heat and work. Industrial steam turbines function by converting the thermal energy in steam into mechanical energy, which can be used to perform useful work, such as generating electricity or driving machinery. To understand how this process works, it’s important to grasp key thermodynamic principles and cycles, especially the Rankine cycle, as well as concepts like entropy, enthalpy, and isentropic efficiency.
The Rankine Cycle
The Rankine cycle is the fundamental thermodynamic cycle that describes how steam turbines convert heat into work. This cycle outlines the processes involved in generating steam, expanding it through the turbine to extract work, and then condensing it back into water for reuse. The Rankine cycle consists of four main stages:
- Heat Addition in the Boiler (Isobaric Process):
- In this stage, water is heated in a boiler under constant pressure until it becomes steam. The heat added during this process increases the energy of the water molecules, transforming it into steam. Depending on the system’s requirements, the steam may be saturated (steam that exists at the boiling point of water for a given pressure) or superheated (steam heated beyond its boiling point).
- Expansion in the Turbine (Isentropic Process):
- After the steam is generated, it enters the turbine at high pressure and temperature. As it expands through the turbine, its pressure and temperature drop, and it performs work by causing the turbine blades to rotate. This rotation is converted into mechanical work, which can then be used to drive an electric generator or mechanical equipment.
- This stage is ideally isentropic, meaning it occurs without any change in entropy, and the energy loss due to friction and other inefficiencies is minimized.
- Condensation in the Condenser (Isobaric Process):
- After the steam has passed through the turbine and given up most of its energy, it enters the condenser, where it is cooled and returned to liquid form at constant pressure. The condensation process releases the remaining heat into a cooling medium, such as water or air. This step is necessary to maintain the pressure differential across the turbine, which drives the continuous flow of steam.
- Pressurization in the Pump (Isentropic Process):
- In the final stage, the condensed water is pumped back into the boiler to restart the cycle. The pump increases the pressure of the liquid water to the level required for it to be heated again in the boiler, completing the closed loop.
The Rankine cycle is the basis for the operation of most steam turbines in power plants and industrial settings. By repeatedly cycling steam through these four stages, steam turbines can continuously convert thermal energy into mechanical work.
Energy Conversion: From Heat to Mechanical Work
The conversion of thermal energy from steam into mechanical energy is the core function of a steam turbine. This energy conversion process can be described using enthalpy and entropy—two key thermodynamic properties that determine how efficiently a steam turbine operates.
- Enthalpy (h):
- Enthalpy is a measure of the total energy content of the steam, including both its internal energy (related to temperature) and the energy associated with its pressure. When steam enters the turbine, it has a high enthalpy because it is under high pressure and temperature. As the steam expands through the turbine and performs work, its enthalpy decreases.
- The enthalpy drop between the high-pressure inlet and the low-pressure outlet of the turbine represents the amount of energy extracted from the steam and converted into mechanical work. A larger enthalpy drop indicates more work output from the turbine.
- Entropy (s):
- Entropy is a measure of disorder in a thermodynamic system. In the context of a steam turbine, entropy increases as the steam expands and loses energy. The goal of turbine design is to minimize entropy increases during the expansion process, which would otherwise represent energy losses due to irreversibilities, such as friction or heat transfer to the surroundings.
- In an ideal, reversible process, entropy remains constant (an isentropic process). However, real steam turbines operate with some level of inefficiency, meaning that the process is not perfectly isentropic, and some energy is lost as heat or due to friction.
Isentropic Efficiency of Steam Turbines
Isentropic efficiency is a measure of how closely a real turbine’s performance approaches the ideal isentropic process. It is defined as the ratio of the actual work output of the turbine to the maximum possible work output (isentropic work). This efficiency gives engineers a way to quantify the losses in the system and identify areas for improvement.
- Isentropic Efficiency Formula:ηisentropic=Actual Work OutputIsentropic Work Output\eta_{\text{isentropic}} = \frac{\text{Actual Work Output}}{\text{Isentropic Work Output}}ηisentropic=Isentropic Work OutputActual Work OutputIn practical terms, isentropic efficiency is typically between 70% and 90% for modern industrial steam turbines. Losses that prevent turbines from achieving 100% efficiency include:
- Friction losses: Occur between the moving parts of the turbine, such as in the bearings or between the blades and the steam.
- Steam leakage: If steam leaks around seals or other components, the energy carried by that steam is lost.
- Heat losses: Some energy may be lost as heat to the surroundings, particularly in older or poorly insulated turbines.
Improving isentropic efficiency is crucial for maximizing the performance of a steam turbine. Engineers work to reduce friction, minimize leakage, and enhance insulation to improve the overall efficiency of steam turbines.
Heat Rate and Efficiency in Power Generation
In power generation, the heat rate is a key metric used to evaluate the efficiency of a steam turbine. It is defined as the amount of heat energy required to produce one unit of electrical energy. In simpler terms, it reflects how efficiently a power plant converts fuel into electricity.
- Heat Rate Formula:Heat Rate=Energy Input (Fuel)Electrical Energy Output\text{Heat Rate} = \frac{\text{Energy Input (Fuel)}}{\text{Electrical Energy Output}}Heat Rate=Electrical Energy OutputEnergy Input (Fuel)The heat rate is usually expressed in terms of British Thermal Units (BTUs) per kilowatt-hour (kWh) of electricity produced. Lower heat rates indicate better efficiency, as less fuel is required to produce the same amount of electricity.
- Thermal Efficiency: Thermal efficiency is another way to express the overall performance of a steam turbine system. It is the ratio of the useful work output to the total energy input:ηthermal=Work OutputHeat Input\eta_{\text{thermal}} = \frac{\text{Work Output}}{\text{Heat Input}}ηthermal=Heat InputWork OutputThe higher the thermal efficiency, the more effectively the turbine converts heat into work, reducing fuel consumption and operational costs.
Improving the thermal efficiency of steam turbines not only increases energy output but also reduces the environmental impact by lowering fuel consumption and emissions. Technologies such as reheat cycles, regenerative feedwater heating, and superheated steam are commonly used to improve the thermal efficiency of steam turbines in power plants.
The Role of Reheat Cycles in Improving Efficiency
One of the most effective ways to improve the efficiency of a steam turbine is by incorporating a reheat cycle into the Rankine cycle. In a reheat cycle, the steam is partially expanded in the turbine, then returned to the boiler to be reheated before it continues through the remaining turbine stages.
- Reheat Process:
- During the reheat process, steam is expanded in the high-pressure turbine, losing some of its energy. It is then returned to the boiler, where it is reheated to its original or near-original temperature. This reheated steam is sent back into the intermediate- or low-pressure turbine for further expansion.
- Benefits of Reheat Cycles:
- Reheating the steam allows for more energy to be extracted at higher average temperatures, increasing the overall thermal efficiency of the turbine. The reheat process also reduces the moisture content in the steam, preventing damage to the turbine blades caused by wet steam.
Entropy and Enthalpy Diagrams (Mollier Diagrams)
Engineers and turbine operators frequently use Mollier diagrams (also known as enthalpy-entropy diagrams) to visualize the thermodynamic processes occurring in steam turbines. These diagrams plot enthalpy on the vertical axis and entropy on the horizontal axis, allowing for a graphical representation of the steam expansion process within the turbine.
- Using Mollier Diagrams: Mollier diagrams help engineers determine the state of the steam at various points in the Rankine cycle. By analyzing the enthalpy and entropy values before and after expansion, engineers can calculate the amount of work extracted by the turbine, assess the system’s efficiency, and identify potential areas for performance improvement.
Conclusion
Thermodynamic principles are central to the operation of steam turbines. The Rankine cycle provides the foundation for understanding how heat is converted into mechanical work, while key concepts like enthalpy, entropy, and isentropic efficiency offer insights into how energy is transferred and lost during the process. By applying these principles and optimizing the efficiency of steam turbines, engineers can enhance energy output, reduce fuel consumption, and improve the sustainability of industrial power systems.
Steam Properties and Their Role in Turbine Efficiency
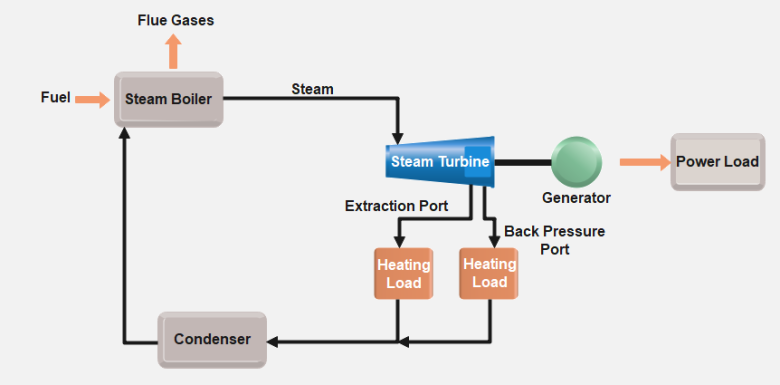
The properties of the steam used in a steam turbine—such as its pressure, temperature, and quality—play a crucial role in determining the turbine’s overall efficiency and performance. Steam is the working fluid in a turbine, and its ability to carry thermal energy for conversion into mechanical work depends on its specific characteristics. Understanding the distinctions between saturated steam, superheated steam, and the importance of steam quality is essential for optimizing turbine operation and ensuring long-term reliability.
Saturated vs. Superheated Steam
There are two primary types of steam used in industrial steam turbines: saturated steam and superheated steam. Each type has distinct characteristics that influence how efficiently energy can be extracted from the steam.
- Saturated Steam:
- Saturated steam is steam that is in equilibrium with liquid water at a given temperature and pressure. This means that the steam contains the maximum amount of energy it can hold at a specific pressure, without being heated beyond its boiling point. Any additional heat input would cause some of the water to vaporize into steam rather than increasing the temperature of the steam itself.
- Properties of Saturated Steam:
- Saturated steam contains both vapor and liquid phases, which can lead to issues such as condensation and moisture buildup during expansion in a turbine.
- It is ideal for applications where both heat and power are needed, such as in cogeneration systems, because the moisture content can be used for industrial heating processes.
- Challenges in Turbine Efficiency:
- In steam turbines, saturated steam can lead to inefficiencies because as it expands, some of the steam may condense back into water. This wet steam can cause blade erosion and reduce turbine efficiency by introducing energy losses due to the presence of liquid droplets.
- Superheated Steam:
- Superheated steam is produced by heating saturated steam beyond its boiling point, at a constant pressure, until it no longer contains any liquid water. Superheated steam is “dry” and has a higher energy content than saturated steam at the same pressure.
- Properties of Superheated Steam:
- Superheated steam has a higher temperature for the same pressure compared to saturated steam, which means it can deliver more energy to the turbine before it condenses.
- It is preferred in most power generation applications because its high thermal energy content allows for greater efficiency during the expansion process in the turbine.
- Advantages for Turbine Efficiency:
- Superheated steam improves the turbine’s thermal efficiency, as it allows for more complete energy extraction without the risk of moisture buildup. Additionally, it minimizes the risk of erosion on the turbine blades, extending the equipment’s lifespan and reducing maintenance requirements.
In most large-scale power generation systems, particularly in fossil-fuel and nuclear power plants, superheated steam is used because it provides higher efficiency and protects the turbine from damage.
Pressure, Temperature, and Steam Quality
The pressure and temperature of the steam entering the turbine significantly affect the turbine’s performance and energy output. Steam quality, which refers to the proportion of vapor in the steam (as opposed to liquid water), is also a critical factor in determining how efficiently the steam transfers energy to the turbine.
- Pressure and Temperature:
- The efficiency of a steam turbine is highly dependent on the inlet steam pressure and temperature. Higher steam pressures and temperatures lead to greater energy content in the steam, which allows for more work to be extracted from the steam as it expands in the turbine.
- High-pressure and High-temperature Steam:
- Modern steam turbines, particularly in power plants, often operate with superheated steam at extremely high pressures (up to 240 bar) and high temperatures (up to 600°C). This increases the amount of energy available for conversion into mechanical work.
- Efficiency Benefits: Higher inlet pressures and temperatures improve the thermal efficiency of the turbine, as more energy is extracted from the steam before it is exhausted or condensed. These turbines can achieve efficiency rates of 40-50% or higher in modern power plants.
- Steam Temperature Control: To prevent damage to turbine blades, it is essential to carefully control the temperature of the steam. Overheating can cause thermal stress and degrade the turbine materials, while steam that is too cool may condense prematurely, leading to moisture-related issues.
- Steam Quality:
- Steam quality refers to the ratio of vapor to liquid in a mixture of steam. A steam quality of 100% means that the steam is completely vapor (dry steam), while a steam quality of less than 100% indicates that some portion of the steam is liquid water.
- Importance of High Steam Quality:
- Steam quality is especially important for maintaining turbine efficiency and protecting turbine components. When steam enters a turbine with a quality lower than 100%, it contains liquid droplets that can cause erosion and pitting of the turbine blades, leading to reduced efficiency and costly repairs.
- For optimal turbine performance, it is crucial to maintain as high a steam quality as possible. This is particularly important in the low-pressure stages of a turbine, where steam tends to condense as it expands and cools.
- Dealing with Wet Steam:
- In some cases, moisture separators or reheat systems are used to reduce the moisture content of the steam. In a reheat cycle, partially expanded steam is returned to the boiler for reheating, which increases its energy content and improves steam quality before it reenters the turbine for further expansion.
Wetness and Steam Moisture Content
Steam moisture content, or the presence of liquid water in the steam, is a critical factor that can have a significant impact on turbine efficiency and longevity. As steam expands in the turbine, its temperature and pressure decrease, causing some of the steam to condense into water droplets. This is especially common in low-pressure turbines, where steam reaches lower temperatures.
- Effects of Wet Steam:
- Efficiency Losses: Wet steam is less efficient at transferring energy to the turbine blades because the liquid droplets do not contribute to the kinetic energy that drives the turbine. As a result, the presence of moisture reduces the overall efficiency of the turbine.
- Blade Erosion: The high-speed liquid droplets in wet steam can cause significant damage to the turbine blades over time. As the droplets collide with the blades, they can cause erosion, pitting, and surface wear, which leads to a decrease in turbine performance and increased maintenance requirements.
- Methods for Managing Steam Moisture:
- Reheat Cycles: One of the most effective methods for reducing steam moisture is the use of reheat cycles. After partial expansion in the high-pressure turbine, the steam is sent back to the boiler for reheating before entering the intermediate- or low-pressure stages. This increases the steam’s temperature and decreases its moisture content, resulting in higher efficiency and reduced wear on the blades.
- Moisture Separators: In some systems, moisture separators are used to remove water droplets from the steam before it enters the turbine or between turbine stages. These devices help to ensure that the steam entering the turbine has minimal moisture content, improving efficiency and reducing the risk of erosion.
Conclusion
The properties of steam—whether it is saturated or superheated, its pressure, temperature, and quality—are critical to the performance and efficiency of industrial steam turbines. Superheated steam, with its higher energy content, is generally preferred in power generation applications for its ability to maximize energy extraction and protect turbine components. Maintaining high steam quality is essential to prevent damage from moisture and ensure that the turbine operates efficiently over the long term. By optimizing steam properties and managing steam moisture content, engineers can enhance turbine performance, reduce maintenance costs, and extend the operational lifespan of the system.
Types of Industrial Steam Turbines
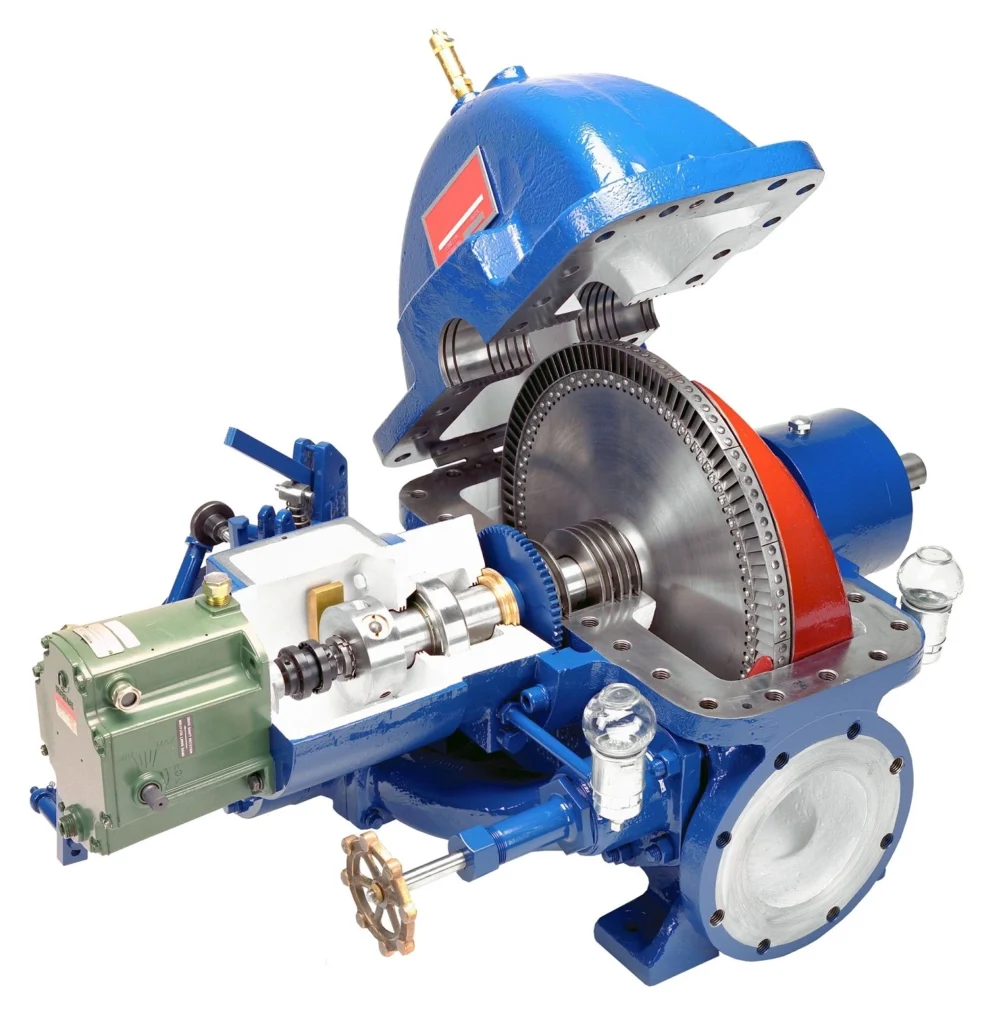
Industrial steam turbines are categorized based on their design, operating principles, and specific applications. While all steam turbines convert the thermal energy of steam into mechanical work, they differ in how they achieve this conversion, the steam conditions they handle, and the industrial processes they support. The main types of industrial steam turbines include impulse and reaction turbines, condensing and non-condensing turbines, as well as more specialized configurations such as back-pressure and extraction turbines.
Each type of steam turbine has its own advantages and is suited to different industrial settings, depending on factors like the need for electricity generation, cogeneration (combined heat and power), or industrial heating. This section explores these types in detail, explaining their working principles and applications.
Impulse vs. Reaction Turbines
One of the fundamental ways to classify steam turbines is by the working principle they use to convert steam energy into mechanical work. The two primary types are impulse turbines and reaction turbines, both of which operate based on different steam flow dynamics.
- Impulse Turbines:
- In an impulse turbine, steam is directed through nozzles, where it undergoes a rapid pressure drop and is converted into high-velocity steam jets. These steam jets are then directed onto the turbine blades, imparting a force that causes the rotor to spin. The energy conversion in an impulse turbine primarily occurs due to the kinetic energy of the steam.
- Working Principle: The nozzles accelerate the steam, increasing its velocity while keeping the pressure relatively constant as it moves across the turbine blades. The force of the high-velocity steam on the turbine blades creates a turning motion.
- Applications: Impulse turbines are often used in high-pressure stages of power plants and industrial processes where the steam enters the turbine at a high pressure. They are well-suited for applications requiring simple designs, such as in marine propulsion and mechanical drives.
- Advantages:
- Impulse turbines can handle high-pressure steam efficiently.
- They are relatively simple to design and maintain.
- Disadvantages:
- Impulse turbines tend to have lower efficiency at low steam velocities or pressures.
- Reaction Turbines:
- In a reaction turbine, the steam expands both in stationary nozzles and as it passes over the blades. In contrast to impulse turbines, where most of the energy conversion happens in the nozzles, reaction turbines extract energy from the steam as it changes both pressure and velocity as it moves through the turbine.
- Working Principle: As the steam moves through the turbine blades, it expands and undergoes a drop in pressure, which generates additional velocity. This gradual expansion and pressure drop allow the turbine to capture more energy from the steam.
- Applications: Reaction turbines are often used in medium- to low-pressure stages in power plants, where the steam expands more gradually. They are also employed in applications where efficiency needs to be maximized over a range of pressures and temperatures.
- Advantages:
- Reaction turbines tend to have higher efficiency at lower steam pressures compared to impulse turbines.
- The smooth expansion of steam results in a more continuous energy extraction process.
- Disadvantages:
- Reaction turbines are more complex in design and require precise control of steam flow and pressure.
Condensing vs. Non-condensing (Back-pressure) Turbines
Steam turbines can also be classified by how they handle exhaust steam after it has passed through the turbine. This leads to the distinction between condensing turbines and non-condensing turbines (also known as back-pressure turbines).
- Condensing Turbines:
- Condensing turbines are designed to maximize energy extraction by condensing the steam into water at the turbine’s exhaust. In these systems, steam is expanded to a very low pressure and temperature in the turbine before entering a condenser, where it is cooled and converted back into liquid water. This process creates a large pressure difference between the inlet and the outlet of the turbine, allowing for maximum energy extraction.
- Applications: Condensing turbines are primarily used in large-scale power generation, particularly in fossil fuel, nuclear, and geothermal power plants, where electricity production is the main goal.
- Advantages:
- Condensing turbines are highly efficient at extracting energy from steam, making them ideal for power generation.
- They are designed to handle low-pressure exhaust steam, which enhances overall energy conversion.
- Disadvantages:
- These turbines require complex systems, including cooling water circuits and condensers, which add to the plant’s cost and complexity.
- Large volumes of cooling water may be required, limiting their use in water-scarce regions.
- Non-condensing (Back-pressure) Turbines:
- In a non-condensing turbine, the steam is not condensed after passing through the turbine. Instead, it is exhausted at a higher pressure, which can be used for further industrial processes, such as heating, drying, or chemical reactions. These turbines are often used in cogeneration systems, where both electricity and heat are needed.
- Applications: Non-condensing turbines are widely used in industries such as chemical plants, refineries, pulp and paper mills, and food processing, where steam is required for both mechanical work and industrial heating.
- Advantages:
- Non-condensing turbines are highly efficient in cogeneration applications since they provide both electricity and heat for industrial processes.
- They are simpler and cheaper to install and maintain than condensing turbines, as they do not require condensers or extensive cooling systems.
- Disadvantages:
- Non-condensing turbines are not as efficient for electricity generation alone, as they extract less energy from the steam compared to condensing turbines.
Back-pressure, Extraction, and Reheat Turbines
Some industrial processes require more specialized steam turbine configurations to balance between electricity generation and heat supply. Back-pressure turbines, extraction turbines, and reheat turbines are tailored to specific industrial needs.
- Back-pressure Turbines:
- Back-pressure turbines exhaust steam at a higher pressure than condensing turbines, allowing the steam to be reused in other industrial processes. This makes them ideal for cogeneration or district heating systems.
- Working Principle: After generating electricity in the turbine, the steam exits at a pressure that is still high enough to be used in heating systems or other industrial processes.
- Applications: These turbines are common in industrial plants that need both mechanical power and steam for heating or processing purposes.
- Extraction Turbines:
- Extraction turbines are designed to extract steam at one or more intermediate stages in the turbine, allowing the steam to be used for industrial processes at different pressures. The remaining steam continues to expand through the turbine to generate more electricity.
- Working Principle: Steam is partially expanded in the turbine, then “extracted” at a desired pressure level for use in processes such as drying, heating, or cooling. The remaining steam continues to expand and generate electricity.
- Applications: Extraction turbines are commonly used in industries such as pulp and paper, petrochemical refining, and chemical manufacturing, where steam is needed at different pressures for various processes.
- Advantages:
- Flexible steam supply at different pressure levels allows the turbine to meet varying process demands.
- They offer a balance between power generation and steam supply for industrial use.
- Reheat Turbines:
- Reheat turbines are used in large power plants to increase the efficiency of the steam cycle. In these turbines, steam is partially expanded in the high-pressure section, then returned to the boiler for reheating before continuing through the intermediate- and low-pressure sections of the turbine.
- Working Principle: The reheat cycle increases the average temperature at which heat is added to the system, improving the overall efficiency of the Rankine cycle. Reheating the steam reduces the risk of condensation in the low-pressure stages, protecting the turbine blades from erosion.
- Applications: Reheat turbines are common in large power plants, particularly in coal, gas, and nuclear power plants, where maximizing efficiency is critical.
- Advantages:
- Reheating increases the efficiency of the turbine and reduces the moisture content of the steam.
- These turbines allow for more energy extraction before the steam is condensed or exhausted.
Conclusion
The various types of industrial steam turbines—whether classified by working principle (impulse vs. reaction) or by application (condensing, non-condensing, extraction, or reheat)—each offer unique advantages depending on the operational requirements. Understanding the specific characteristics of each type allows engineers to select the appropriate turbine for a given application, whether it’s maximizing power generation in a utility-scale power plant or efficiently meeting the needs of an industrial facility that requires both electricity and steam. As industries continue to seek more efficient and sustainable energy solutions, these specialized turbine configurations will remain vital in optimizing energy conversion processes.
Design and Construction of Steam Turbines
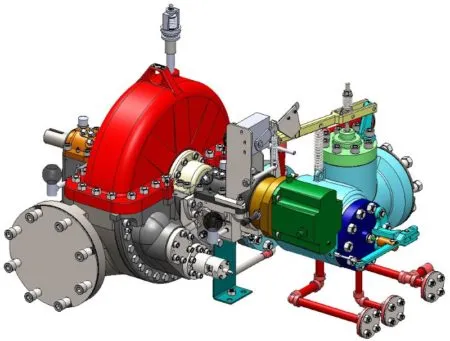
The design and construction of steam turbines require precision engineering to ensure high efficiency, reliability, and durability. Steam turbines operate in environments with extreme temperatures and pressures, making material selection, aerodynamic design, and thermal management critical to their performance. This section delves into the major components of a steam turbine, the materials used, key design considerations, and the technological advancements that have led to more efficient and longer-lasting turbines.
Major Components of a Steam Turbine
Steam turbines consist of several critical components, each playing a crucial role in the turbine’s ability to convert steam energy into mechanical work. These components must be designed to withstand the high thermal and mechanical stresses imposed by the steam’s energy and ensure smooth operation over long periods.
- Rotor:
- The rotor is the central rotating shaft of the steam turbine, onto which the turbine blades are mounted. It converts the energy from steam into rotational mechanical energy, which is then used to drive a generator or mechanical load.
- Design and Function: Rotors are designed to be as lightweight as possible while maintaining the strength to withstand the high centrifugal forces generated by the rotating blades. They are typically made from high-strength steel alloys or other advanced materials that resist thermal expansion and mechanical stress.
- Operational Challenges: As the rotor spins at thousands of revolutions per minute (RPM), it must be perfectly balanced to avoid excessive vibration. Even slight imbalances can cause wear on bearings and seals, leading to reduced efficiency and potential mechanical failures.
- Blades:
- The blades, also called buckets in some turbine configurations, are the primary components that interact with the steam. As steam flows through the turbine, it imparts kinetic energy to the blades, causing the rotor to spin. Blades are typically mounted in stages along the rotor, each stage designed to handle specific pressure and temperature conditions.
- Blade Materials: Steam turbine blades are exposed to extreme conditions, including high temperatures, high pressures, and high-velocity steam. To handle these conditions, blades are typically made from high-strength alloys, such as nickel-based superalloys, which retain their strength and resist corrosion at high temperatures. In addition, blades may be coated with special materials to resist erosion and corrosion from steam impurities.
- Blade Design: The shape and aerodynamic design of turbine blades are critical for optimizing efficiency. Engineers use computational fluid dynamics (CFD) to design blades with complex shapes that minimize energy losses due to turbulence and maximize the energy extracted from the steam. Modern turbines use 3D blades with variable angles of attack to ensure smooth and efficient steam flow.
- Casing:
- The casing encloses the turbine and houses the rotor and blades. It serves several important functions, including guiding the steam through the turbine stages and containing the high-pressure steam as it expands and releases energy.
- Materials: The casing is typically made from high-strength steel or cast iron, designed to withstand the thermal expansion and mechanical stresses caused by the high temperatures and pressures of the steam. The casing is often insulated to minimize heat loss and improve thermal efficiency.
- Thermal Expansion: Steam turbines operate at high temperatures, causing the metal components, including the casing, to expand. To prevent mechanical stress or misalignment, casings are designed with expansion joints or made from materials that tolerate thermal expansion without compromising structural integrity.
- Nozzles:
- Nozzles are responsible for directing the flow of high-pressure steam onto the turbine blades. In impulse turbines, the nozzles convert the steam’s pressure energy into kinetic energy by accelerating the steam to high velocities. In reaction turbines, the nozzles are incorporated into the blade design, allowing steam to expand and drop in pressure as it passes through each stage.
- Design Considerations: The shape and orientation of the nozzles are designed to ensure that steam impacts the blades at the correct angle, maximizing energy transfer and minimizing losses. Nozzles are often made from high-strength materials that resist erosion caused by high-velocity steam.
- Bearings:
- Bearings support the rotor and allow it to rotate smoothly at high speeds. Steam turbines use both radial and axial bearings to manage the forces generated by the rotor’s spinning motion.
- Material and Design: Bearings must be designed to handle the high loads and rotational speeds of the turbine while minimizing friction. They are typically made from high-performance materials such as steel alloys or ceramics, and may use oil or magnetic lubrication to reduce wear.
- Vibration Management: Bearings also play a key role in managing vibration, which can occur due to imbalances in the rotor or blade assembly. Advanced bearing systems use vibration-damping materials or designs to reduce mechanical stress and ensure smooth operation.
- Seals:
- Seals are used to prevent steam from escaping from the turbine at the points where the rotor passes through the casing. They are critical for maintaining efficiency, as any steam leakage reduces the amount of energy available for conversion into mechanical work.
- Types of Seals: Common seal types include labyrinth seals, which create multiple obstacles to prevent steam from leaking, and carbon seals, which provide a tighter seal but may wear over time. Seals must be designed to withstand the high temperatures and pressures inside the turbine while minimizing friction and wear.
- Condenser (in Condensing Turbines):
- In condensing turbines, after steam has passed through the turbine, it enters the condenser, where it is cooled and returned to liquid water. The condenser is a crucial component in power generation systems that use the Rankine cycle, as it helps maintain the pressure differential that drives steam flow through the turbine.
- Design: Condensers are usually large heat exchangers that use cooling water or air to remove heat from the exhaust steam. They are designed to maximize heat transfer efficiency while minimizing the amount of cooling water required.
Materials Used in Steam Turbine Construction
The materials used in the construction of steam turbines must withstand extreme temperatures, high pressures, and mechanical stresses. The choice of materials is critical for ensuring the long-term reliability and efficiency of the turbine.
- Nickel-based Alloys:
- Nickel-based superalloys are commonly used for turbine blades and other high-temperature components because of their excellent resistance to heat, oxidation, and creep (slow deformation under stress). These alloys maintain their strength and structural integrity at temperatures exceeding 600°C, making them ideal for use in both high-pressure and low-pressure turbine stages.
- Chromium-based Alloys:
- Chromium-based alloys are often used in turbine blades, rotors, and casings due to their corrosion resistance. Chromium helps form a protective oxide layer on the surface of the metal, preventing oxidation and chemical corrosion caused by the high-temperature steam.
- Steel Alloys:
- High-strength steel alloys are used for structural components such as the rotor, casing, and nozzles. These materials are chosen for their ability to withstand high mechanical stresses and resist fatigue caused by the turbine’s continuous operation.
- Ceramic Coatings:
- In some high-temperature applications, turbine blades are coated with ceramic materials to provide additional thermal protection. Ceramic coatings, also known as thermal barrier coatings (TBCs), protect the underlying metal from the extreme heat of the steam, allowing the turbine to operate at higher temperatures without suffering damage.
Design Considerations for Efficiency and Durability
Several key design considerations ensure that steam turbines operate at maximum efficiency while maintaining long-term durability. These factors include aerodynamic efficiency, thermal management, and minimizing mechanical losses.
- Aerodynamic Blade Design:
- The shape and profile of the turbine blades are critical for maximizing energy extraction from the steam. Engineers use computational fluid dynamics (CFD) to simulate steam flow and optimize the blade design. Modern turbine blades are designed to maintain laminar flow, which reduces turbulence and energy losses.
- 3D Blades: Many modern turbines use 3D blades with variable angles of attack and complex curvatures. These designs allow for better control of steam flow, minimizing energy losses and improving overall efficiency.
- Multi-stage Design:
- Most industrial steam turbines are multi-stage machines, where steam passes through several sets of blades before exiting the turbine. Each stage is designed to extract energy from the steam at different pressure and temperature levels. By using multiple stages, the turbine can extract more energy from the steam, improving efficiency.
- High-Pressure, Intermediate-Pressure, and Low-Pressure Stages: Multi-stage turbines are divided into high-pressure (HP), intermediate-pressure (IP), and low-pressure (LP) sections, with each section optimized for different steam conditions. The combination of these stages allows the turbine to operate efficiently across a wide range of steam pressures.
- Thermal Management and Cooling Systems:
- Efficient thermal management is essential for ensuring the turbine operates at high temperatures without suffering damage. Blade cooling systems are used in high-temperature turbines to prevent overheating and extend the life of the turbine blades.
- Internal Cooling: Many modern turbine blades have internal cooling channels that circulate air or coolant to reduce the temperature of the blades, allowing the turbine to operate at higher inlet temperatures while maintaining durability.
- Vibration Control and Noise Reduction:
- Steam turbines operate at very high rotational speeds, making vibration a significant concern. Excessive vibration can lead to mechanical failures, reduce efficiency, and shorten the turbine’s operational life. Advanced bearing designs, balanced rotors, and vibration-damping systems are used to manage and minimize vibration.
- Noise Reduction: Steam turbines can generate significant noise during operation, particularly at high speeds. Noise-reduction technologies, such as acoustic insulation and vibration-damping materials, are often used to reduce the impact of noise in industrial environments.
Advances in Turbine Technology
Technological advancements in materials, design, and digitalization have significantly improved the performance and efficiency of steam turbines in recent years. Some of the key advancements include:
- 3D Printing and Additive Manufacturing:
- The use of additive manufacturing (3D printing) in turbine construction has enabled the production of complex, high-precision parts that were previously difficult or impossible to manufacture using traditional methods. 3D printing allows for the optimization of blade designs, leading to higher efficiency and reduced manufacturing costs.
- Smart Turbines and Digital Twins:
- Modern turbines are increasingly equipped with sensors that monitor performance in real-time, providing data on temperature, pressure, vibration, and efficiency. This data can be used to predict maintenance needs and optimize turbine operation.
- Digital Twins: The concept of digital twins—virtual models of physical turbines—allows engineers to simulate different operating conditions and optimize turbine performance without disrupting actual operations.
- Advanced Coatings and Materials:
- New materials and coatings are being developed to increase the durability and efficiency of turbine blades. Erosion-resistant coatings and thermal barrier coatings (TBCs) improve the lifespan of components and allow turbines to operate at higher temperatures without suffering from thermal fatigue or corrosion.
Conclusion
The design and construction of steam turbines require a careful balance between maximizing efficiency and ensuring durability. By using advanced materials, precision engineering, and sophisticated design techniques, modern steam turbines are able to operate at high pressures and temperatures while maintaining long-term reliability. As technology continues to evolve, steam turbines will become even more efficient, durable, and adaptable to the changing demands of the energy industry.
Working Stages of a Steam Turbine
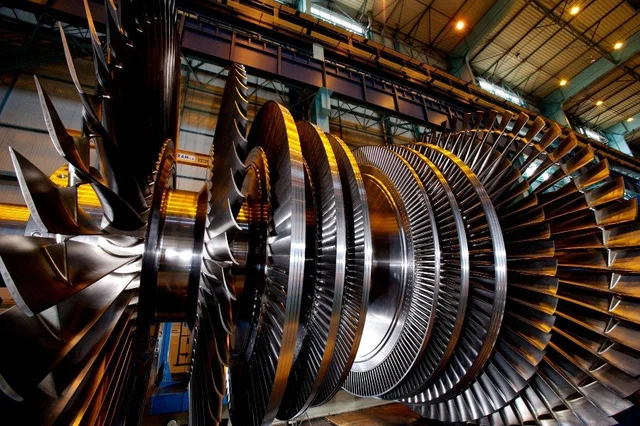
In industrial steam turbines, steam passes through several stages before exiting the system, with each stage optimized to extract energy from the steam at varying pressures and temperatures. These stages, known as the high-pressure (HP), intermediate-pressure (IP), and low-pressure (LP) stages, allow the turbine to efficiently convert the maximum amount of thermal energy from the steam into mechanical work. By using multiple stages, turbines achieve higher efficiencies and can handle larger variations in steam conditions, making them ideal for power generation and industrial applications.
This section outlines the working principles of each stage, the design considerations, and how multi-stage designs enhance turbine efficiency.
High-Pressure (HP) Stage
The high-pressure (HP) stage is the first stage in most industrial steam turbines, where steam enters at its highest pressure and temperature. This stage is designed to extract the maximum energy from the steam before it begins to expand and lose pressure.
- Function of the HP Stage:
- Steam enters the HP stage directly from the boiler, often at pressures as high as 200–240 bar and temperatures between 500°C and 600°C in modern power plants. The high temperature and pressure at this stage mean the steam contains a significant amount of thermal energy, which is then converted into mechanical work as it passes through the turbine.
- The blades in the HP stage are designed to handle these extreme conditions and are typically made from advanced materials like nickel-based superalloys that can withstand high temperatures and pressures without degrading.
- Energy Extraction:
- As the high-pressure steam expands through the HP stage, it undergoes a rapid drop in pressure and temperature, converting its enthalpy (thermal energy) into kinetic energy, which drives the turbine blades.
- The high-pressure stage is particularly important because it accounts for a significant portion of the turbine’s overall energy extraction. The high temperature and pressure conditions provide a larger enthalpy drop, which results in more work being done on the turbine blades.
- Design Considerations:
- The blades and nozzles in the HP stage must be carefully designed to maximize energy extraction while minimizing mechanical stress. Since the steam in this stage is at its hottest and most energetic, efficient thermal management is essential to prevent overheating and material fatigue.
- Advanced blade cooling systems may be used in this stage to ensure that the turbine blades remain at safe operating temperatures, even when handling superheated steam.
Intermediate-Pressure (IP) Stage
After passing through the high-pressure stage, the steam moves into the intermediate-pressure (IP) stage, where it continues to expand and lose pressure. The IP stage is designed to extract additional energy from the steam as it cools and decreases in pressure, but still contains significant energy for conversion.
- Function of the IP Stage:
- The steam entering the IP stage has already lost a portion of its pressure and temperature but still contains enough thermal energy to perform mechanical work. Typical steam pressures in the IP stage range from 30 to 80 bar, depending on the specific turbine design and the steam conditions.
- The blades in the IP stage are optimized for handling lower pressures and temperatures compared to the HP stage. As the steam expands further, the size and shape of the turbine blades are adjusted to capture the remaining energy efficiently.
- Energy Extraction:
- The IP stage continues to extract energy from the steam as it expands and loses pressure. Since the temperature and pressure are lower than in the HP stage, the rate of energy extraction is somewhat lower, but it is still critical for the turbine’s overall efficiency.
- In many modern power plants, the IP stage may also include steam reheating, where the steam is sent back to the boiler for reheating before entering the IP turbine. Reheating increases the steam’s temperature without significantly raising its pressure, allowing for more efficient energy extraction while preventing excessive moisture buildup in the later stages of the turbine.
- Design Considerations:
- The design of the IP stage blades is optimized for steam that is cooler and at a lower pressure compared to the HP stage. The blades in this stage are typically larger and have a different aerodynamic profile to accommodate the expanded steam flow.
- In multi-stage turbines, the IP section may contain multiple rows of blades to allow for more gradual steam expansion, ensuring that as much energy as possible is extracted before the steam moves to the low-pressure stage.
Low-Pressure (LP) Stage
The low-pressure (LP) stage is the final stage in most steam turbines, where the steam expands to its lowest pressure and temperature. By the time the steam reaches the LP stage, much of its energy has already been extracted, but there is still residual energy that can be converted into mechanical work.
- Function of the LP Stage:
- The LP stage is designed to handle steam at significantly lower pressures, often in the range of 0.05 to 5 bar, and at temperatures closer to the boiling point of water. In some cases, the steam may even reach near-vacuum conditions in the LP stage to maximize energy extraction.
- The LP stage typically features the largest blades in the turbine, as the steam expands and occupies a much larger volume. These large blades are necessary to capture the remaining energy from the low-pressure steam.
- Energy Extraction:
- While the energy extraction in the LP stage is lower compared to the HP and IP stages, it is still a critical part of the overall process. The steam is now at a much lower pressure and temperature, meaning that the turbine must efficiently capture the remaining energy before the steam is exhausted.
- In condensing turbines, the LP stage is connected to a condenser, where the steam is cooled and condensed back into liquid water. This creates a vacuum at the turbine exhaust, which helps to increase the pressure differential across the LP stage and improve efficiency.
- Design Considerations:
- The blades in the LP stage must be designed to handle the large volumes of low-pressure steam, which expands significantly as it cools. These blades are usually the longest in the turbine and are made from materials that can withstand the mechanical stresses of spinning at high speeds while handling large steam volumes.
- Moisture control is critical in the LP stage, as the steam may begin to condense into water droplets as it cools. Excess moisture can cause blade erosion and reduce the efficiency of the turbine. To mitigate this, moisture separators or reheat systems may be used to maintain the steam quality.
Multi-stage Designs for Efficiency
Modern industrial steam turbines use multiple stages to maximize energy extraction and improve efficiency. Each stage (HP, IP, and LP) is carefully designed to handle the specific pressure and temperature conditions of the steam as it progresses through the turbine.
- Advantages of Multi-stage Designs:
- Increased Efficiency: Multi-stage turbines allow for more complete energy extraction by using different blade designs for high-, medium-, and low-pressure steam. This ensures that as much energy as possible is converted into mechanical work.
- Improved Flexibility: Multi-stage turbines can handle a wide range of steam conditions, making them ideal for large power plants and industrial systems that operate at varying loads and steam qualities.
- Better Steam Quality Control: By using reheat cycles and moisture separators between stages, multi-stage turbines can maintain high steam quality and minimize the risk of moisture-related issues like blade erosion.
- Reheat Cycles:
- In many large-scale turbines, particularly those used in power plants, a reheat cycle is used to improve efficiency. After the steam passes through the HP stage, it is sent back to the boiler to be reheated before entering the IP stage. This reheating process increases the steam’s temperature without significantly increasing its pressure, allowing for more efficient energy extraction in the later stages.
Conclusion
The working stages of a steam turbine—high-pressure, intermediate-pressure, and low-pressure—are designed to extract the maximum amount of energy from steam as it expands and loses pressure. Each stage plays a critical role in ensuring that the turbine operates efficiently, converting as much thermal energy as possible into mechanical work. Multi-stage designs, combined with reheat cycles and moisture management, ensure that steam turbines can operate efficiently and reliably in a wide range of industrial and power generation applications.
Condensing Turbines
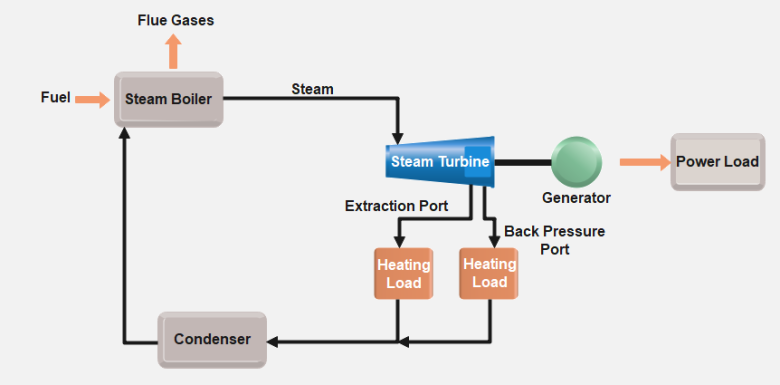
Condensing turbines are a type of steam turbine used primarily in power generation, where the goal is to maximize the extraction of energy from steam. In these turbines, steam is expanded to a low pressure and then condensed back into water using a condenser. The purpose of condensing the steam is to create a large pressure difference across the turbine, which helps to maximize the amount of energy extracted from the steam. The condensed water is then pumped back to the boiler to restart the cycle.
Importance in Industrial Power Generation
Condensing turbines are critical in large-scale power plants, especially those fueled by coal, natural gas, oil, or nuclear energy. They are highly efficient at converting thermal energy into mechanical work, making them ideal for power plants where the primary objective is electricity generation. Unlike back-pressure or extraction turbines, which may also produce heat for industrial processes, condensing turbines focus on electricity production by condensing steam into water to achieve the lowest possible pressure at the turbine outlet.
Historical Background and Evolution
The evolution of steam turbines began with early steam engines, which were inefficient and bulky. The invention of the steam turbine by Sir Charles Parsons in 1884 revolutionized power generation by introducing a far more efficient method of converting steam energy into electricity. Over the years, condensing turbines have become a staple in power plants, evolving with advancements in materials, design, and technology to handle higher pressures and temperatures, resulting in more efficient power generation.
Basic Overview of Function and Applications
In a condensing turbine, high-pressure steam is directed into the turbine’s high-pressure stage, where it expands and transfers energy to the rotating blades. As the steam moves through successive stages—intermediate and low-pressure stages—it continues to expand, losing pressure and temperature. Finally, the steam enters a condenser, where it is cooled and condensed back into water. This process enables the turbine to maximize energy extraction, making condensing turbines ideal for power plants that focus solely on electricity generation.
Working Principles of Condensing Turbines
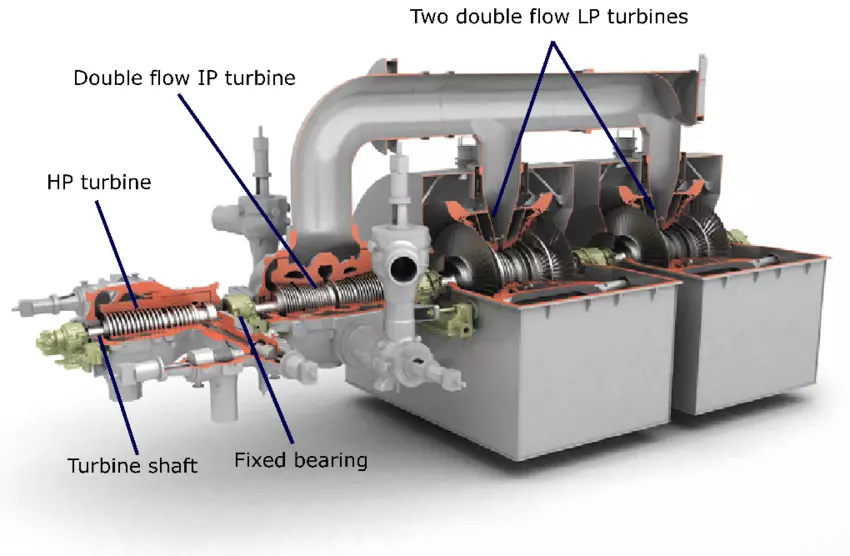
The working principle of condensing turbines is based on the Rankine cycle, a thermodynamic cycle that describes how heat is converted into mechanical work. In this cycle, water is heated to produce steam, which is then expanded through a turbine to generate mechanical energy. After passing through the turbine, the steam is condensed back into water, completing the cycle.
- Heat Addition: Water is heated in a boiler to create steam.
- Expansion: The steam is expanded through the turbine, converting thermal energy into mechanical work.
- Condensation: The steam is condensed in a condenser, reducing its volume and pressure.
- Pressurization: The condensed water is pumped back to the boiler to restart the process.
The key difference between condensing turbines and other steam turbines lies in the condenser, which allows the steam to be cooled and condensed, creating a low-pressure environment that enhances the efficiency of energy extraction.
Energy Conversion: From Steam to Mechanical Work
In condensing turbines, steam enters the turbine at high pressure and temperature, and as it expands through the turbine blades, its energy is converted into rotational mechanical energy. The rotor spins at high speeds, driving a generator to produce electricity. The energy conversion process is highly efficient because the condenser allows for a large pressure differential between the steam entering and exiting the turbine, ensuring that as much energy as possible is extracted.
Role of the Condenser in Pressure Differentials
The condenser plays a critical role in condensing turbines by maintaining a low-pressure environment at the turbine’s exhaust. The steam is cooled in the condenser, typically by water or air, and condensed back into liquid form. This process creates a vacuum at the turbine’s exit, which increases the pressure differential across the turbine stages, allowing for maximum energy extraction from the steam.
How Condensing Turbines Maximize Energy Extraction
By condensing the steam after it has passed through the turbine, condensing turbines can operate with a greater pressure differential than non-condensing turbines. This allows them to extract more energy from the steam, improving the overall efficiency of the power generation process. Condensing turbines are designed to handle large volumes of steam at varying pressures and temperatures, and their multi-stage design ensures that energy is extracted efficiently at every point in the expansion process.
Design of Condensing Turbines
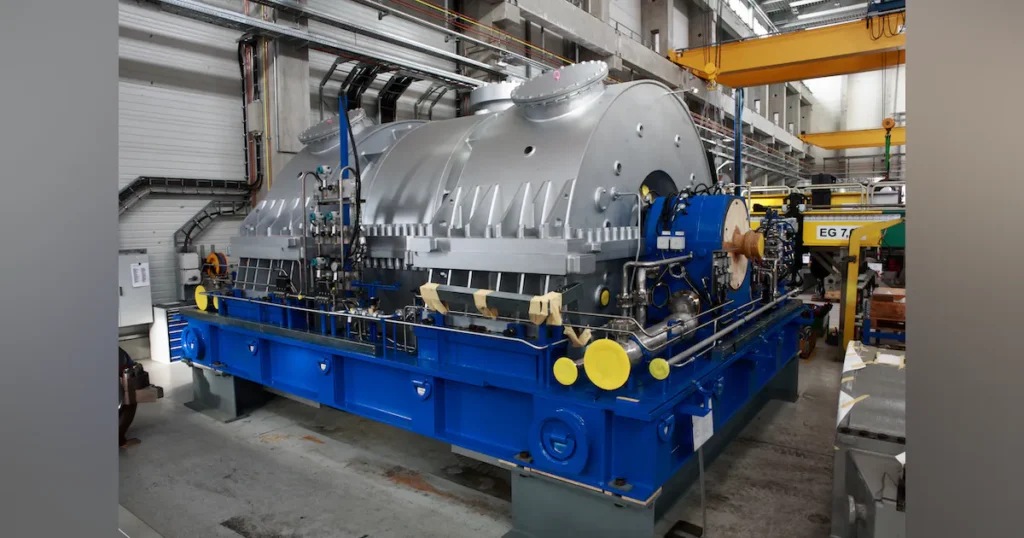
Key Components: Rotor, Blades, Nozzles, Condenser, Bearings, and Seals
The design of condensing turbines involves several key components that work together to efficiently convert thermal energy into mechanical work.
- Rotor: The central shaft of the turbine, which rotates as steam passes over the blades. The rotor is made from high-strength alloys designed to withstand high rotational speeds and thermal stresses.
- Blades: The turbine blades extract energy from the steam by converting its kinetic energy into rotational motion. The blades are designed to handle high-pressure, high-temperature steam and are typically made from corrosion- and heat-resistant alloys.
- Nozzles: In the impulse stages of the turbine, nozzles direct high-velocity steam onto the blades, maximizing energy transfer. The nozzles are designed to minimize pressure loss and optimize the flow of steam through the turbine.
- Condenser: The condenser is one of the most critical components in a condensing turbine. It cools the exhaust steam and condenses it back into liquid water, creating a low-pressure environment that increases the turbine’s efficiency.
- Bearings and Seals: Bearings support the rotor and ensure smooth operation at high speeds, while seals prevent steam leakage, ensuring that the system operates at maximum efficiency.
Materials and Construction
Condensing turbines are constructed from materials that can withstand the high temperatures, pressures, and stresses of steam expansion. Common materials include high-strength steel for the rotor, nickel-based superalloys for the blades, and corrosion-resistant materials for the condenser and other components exposed to steam and water. These materials ensure long-term durability and resistance to wear and tear.
Aerodynamic Blade Design for Efficiency
The design of turbine blades plays a critical role in maximizing the efficiency of condensing turbines. Blades are shaped to minimize turbulence and maximize the conversion of steam’s kinetic energy into rotational energy. Advanced computational fluid dynamics (CFD) is used to design blades that optimize steam flow through the turbine, reducing energy losses and improving overall performance.
Turbine Stages: High-pressure, Intermediate-pressure, and Low-pressure
Condensing turbines are typically multi-stage machines, with steam passing through high-pressure (HP), intermediate-pressure (IP), and low-pressure (LP) stages. Each stage is optimized to extract energy from the steam as it expands and loses pressure:
- High-pressure stage: Handles high-temperature, high-pressure steam from the boiler.
- Intermediate-pressure stage: Expands the steam further, extracting additional energy as pressure and temperature decrease.
- Low-pressure stage: Extracts the remaining energy from the steam before it is condensed in the condenser.
Condenser Design and Function
The condenser in a condensing turbine is designed to maximize heat transfer between the steam and the cooling medium (water or air). Efficient condenser design ensures that the steam is cooled rapidly and condensed back into liquid water, creating a vacuum that improves turbine efficiency. Condensers can be water-cooled or air-cooled, depending on the availability of cooling resources.
Steam Properties and Their Effect on Performance
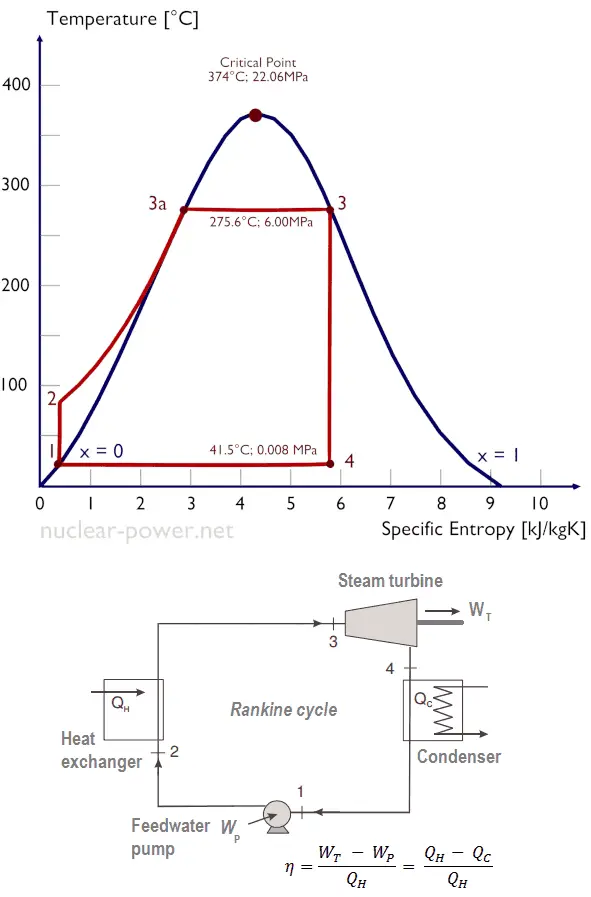
The performance of condensing turbines is heavily influenced by the properties of the steam used in the system. Superheated steam, which has been heated beyond its boiling point at a given pressure, is preferred in condensing turbines because it contains more thermal energy and reduces the risk of condensation during expansion. Saturated steam, which contains both liquid and vapor phases, can lead to efficiency losses and blade erosion due to moisture content.
Pressure, Temperature, and Steam Quality in Condensing Turbines
The pressure and temperature of the steam entering the turbine significantly impact its efficiency. High-pressure, high-temperature steam allows for greater energy extraction, as the pressure differential between the inlet and outlet is maximized. Steam quality, which refers to the ratio of vapor to liquid in the steam, must also be carefully controlled to ensure efficient operation. High-quality steam (dry steam) ensures that more energy is available for conversion into mechanical work.
Dealing with Wet Steam and Moisture Control
As steam expands through the turbine, its temperature and pressure decrease, leading to condensation and the formation of wet steam. Moisture in the steam can cause blade erosion and reduce the turbine’s efficiency. To manage this, modern condensing turbines use moisture separators and reheat systems to ensure that the steam remains as dry as possible throughout the expansion process.
How Steam Properties Influence Efficiency and Blade Erosion
Steam properties such as temperature, pressure, and quality directly affect turbine efficiency. Superheated steam allows for more energy extraction, while wet steam can lead to mechanical wear and tear. Blade erosion from moisture is a common issue in condensing turbines, but this can be mitigated with advanced materials and coatings that protect the blades from wear. Optimizing steam properties is essential for maintaining high turbine efficiency and reducing maintenance costs.
Applications in Power Generation
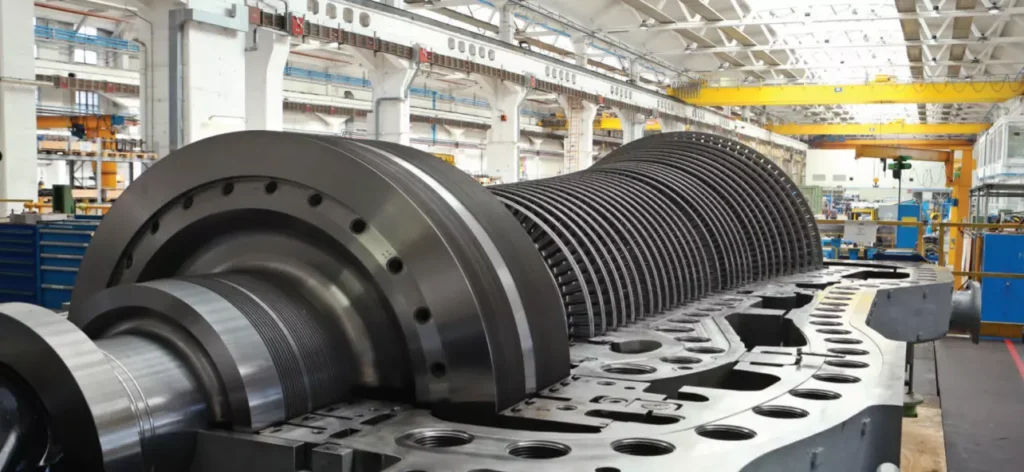
Condensing turbines play a vital role in fossil-fuel power plants, where they are used to convert the thermal energy from burning fuels like coal, natural gas, and oil into electricity. In these plants, steam is generated in a boiler by burning fuel, and the high-pressure steam is directed into the turbine. Condensing turbines are ideal for fossil-fuel plants because they maximize energy extraction by condensing the steam after it has passed through the turbine, allowing for more efficient electricity production.
- Coal-fired Power Plants: Condensing turbines are commonly used in coal-fired power plants, where steam is generated by burning coal in large boilers. These turbines extract the maximum amount of energy from the high-temperature steam, ensuring efficient electricity generation. The use of condensing turbines in coal plants has been a key factor in improving their efficiency and reducing emissions.
- Natural Gas and Combined-cycle Power Plants: In combined-cycle power plants, condensing turbines are used in conjunction with gas turbines to improve overall plant efficiency. The exhaust heat from the gas turbine is used to generate steam, which is then expanded through the condensing turbine to produce additional electricity. This dual-turbine system allows for significantly higher efficiency compared to traditional power plants.
Nuclear Power Plant Applications
Condensing turbines are critical in nuclear power plants, where they convert the heat generated by nuclear fission into electricity. In a nuclear reactor, heat is produced when uranium or other nuclear fuels undergo fission. This heat is used to generate steam, which is directed into the condensing turbine. Due to the immense amounts of heat produced by nuclear reactions, condensing turbines are designed to handle large volumes of steam at high pressures, making them ideal for maximizing energy extraction in nuclear plants.
Use in Combined-cycle Plants
Combined-cycle power plants integrate both gas and steam turbines to improve efficiency. In these plants, a gas turbine generates electricity by burning natural gas, and the hot exhaust gases from the gas turbine are used to produce steam for the condensing turbine. This combined approach increases the overall efficiency of the power plant, often exceeding 60%, making combined-cycle plants one of the most efficient forms of electricity generation.
Integration with Renewable Energy (Biomass and Geothermal)
Condensing turbines are also used in renewable energy applications, particularly in biomass and geothermal power plants. In a biomass plant, organic materials such as wood chips, agricultural waste, or dedicated energy crops are burned to produce steam, which is then expanded through the condensing turbine to generate electricity. In geothermal plants, steam is produced by tapping into geothermal reservoirs beneath the Earth’s surface. Condensing turbines in these plants convert the steam from the hot water or steam reservoirs into electricity, providing a sustainable energy source.
Large-scale Power Plants vs. Distributed Generation
Condensing turbines are predominantly used in large-scale power plants, where their ability to efficiently extract energy from steam makes them ideal for centralized electricity generation. However, advances in turbine technology have led to the development of smaller, more efficient condensing turbines that can be used in distributed generation applications. These turbines are used in industrial facilities and smaller power plants to generate electricity on-site, reducing the need for large-scale infrastructure and improving energy efficiency.
Advantages of Condensing Turbines
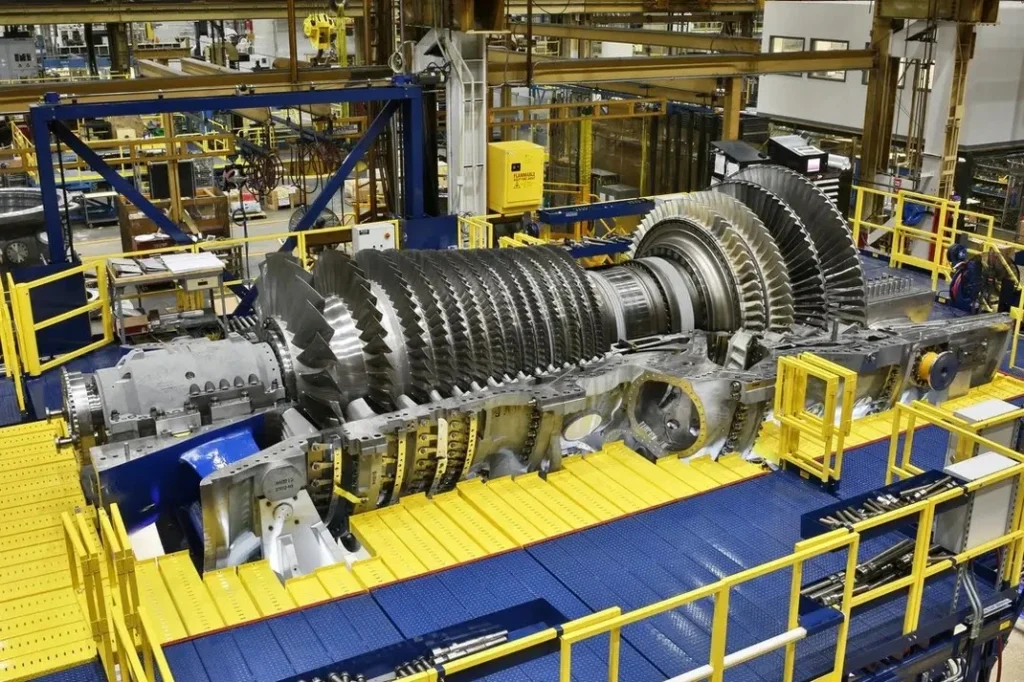
Condensing turbines are among the most efficient types of steam turbines, thanks to their ability to maximize energy extraction from steam. By condensing the steam after it has passed through the turbine, these systems create a large pressure differential between the inlet and outlet, ensuring that as much energy as possible is converted into mechanical work. This makes condensing turbines ideal for applications where electricity generation is the primary objective.
Maximizing Power Output in Power Plants
In large power plants, condensing turbines are used to maximize the amount of electricity produced from a given amount of fuel. By condensing the steam, these turbines allow for more complete energy extraction, leading to higher power output and improved overall efficiency. This is particularly important in fossil-fuel and nuclear power plants, where efficiency gains can significantly reduce fuel consumption and emissions.
Flexibility in Handling Different Steam Conditions
Condensing turbines are designed to handle a wide range of steam conditions, from superheated steam at high pressure to wet steam at lower pressures. This flexibility allows them to be used in various applications, from traditional power plants to renewable energy systems. In particular, condensing turbines are well-suited to combined-cycle plants, where they must handle steam at different temperatures and pressures produced by gas turbine exhaust heat.
Environmental Benefits through Efficiency Improvements
By maximizing the efficiency of electricity generation, condensing turbines help reduce the environmental impact of power plants. Higher efficiency means less fuel is needed to produce the same amount of electricity, leading to lower carbon dioxide (CO₂) emissions and reduced fuel consumption. In addition, the use of condensing turbines in combined-cycle and biomass power plants supports the transition to cleaner energy sources, helping to mitigate climate change.
Challenges and Limitations
One of the main challenges associated with condensing turbines is their dependence on cooling systems to condense the steam after it has passed through the turbine. Water-cooled condensers are commonly used, but they require large amounts of water, which may not be available in all locations. Air-cooled condensers can be used in areas with limited water resources, but they are generally less efficient than water-cooled systems and may require larger infrastructure.
Environmental Challenges: Water Use and Thermal Pollution
The use of large quantities of water for cooling in condensing turbines can lead to environmental issues, including thermal pollution, where heated water is discharged into nearby bodies of water, raising the temperature and potentially harming aquatic ecosystems. Water use in cooling systems also presents a challenge in water-scarce regions, where the availability of cooling water may limit the operation of condensing turbines.
Mechanical Wear and Tear from Wet Steam
As steam expands through the turbine, it can condense into water droplets, leading to wet steam in the later stages of the turbine. This wet steam can cause mechanical wear and tear on the turbine blades, leading to blade erosion and reduced efficiency over time. Managing steam moisture and minimizing blade erosion are ongoing challenges in the operation of condensing turbines.
Blade Erosion and Maintenance Challenges
Blade erosion from moisture in the steam is one of the most common maintenance issues in condensing turbines. Over time, the impact of water droplets on the blades can cause pitting and erosion, reducing the efficiency of the turbine and requiring costly repairs. Modern turbines use advanced materials and coatings to reduce the impact of erosion, but regular maintenance is still necessary to ensure long-term performance.
Performance Monitoring and Efficiency Improvements
Modern condensing turbines are equipped with real-time performance monitoring systems that track key operating parameters such as temperature, pressure, vibration, and steam quality. These systems allow operators to monitor turbine performance and identify potential issues before they lead to equipment failure. By using real-time data, operators can optimize turbine performance and improve overall efficiency.
Digitalization and AI-driven Maintenance
Advances in digitalization and artificial intelligence (AI) are transforming the way condensing turbines are maintained and operated. AI-driven maintenance systems use data from sensors to predict when components are likely to fail, allowing operators to perform maintenance before a breakdown occurs. This approach, known as predictive maintenance, reduces downtime and improves the reliability of condensing turbines.
Efficiency Optimization through Design Improvements
Continued improvements in turbine design are helping to increase the efficiency of condensing turbines. Advances in blade design, aerodynamics, and materials science have led to turbines that can operate at higher temperatures and pressures, improving the efficiency of energy extraction. By optimizing turbine components and reducing energy losses, modern condensing turbines achieve higher efficiencies than older models.
Retrofitting Older Turbines with Modern Technology
Many older condensing turbines can be retrofitted with modern technology to improve their efficiency and performance. Retrofitting options include upgrading turbine blades to more aerodynamic designs, installing advanced cooling systems, and integrating digital monitoring tools. These retrofits can extend the lifespan of older turbines and improve their efficiency, reducing the need for new equipment and lowering operating costs.
Future Trends and Innovations in Condensing Turbines
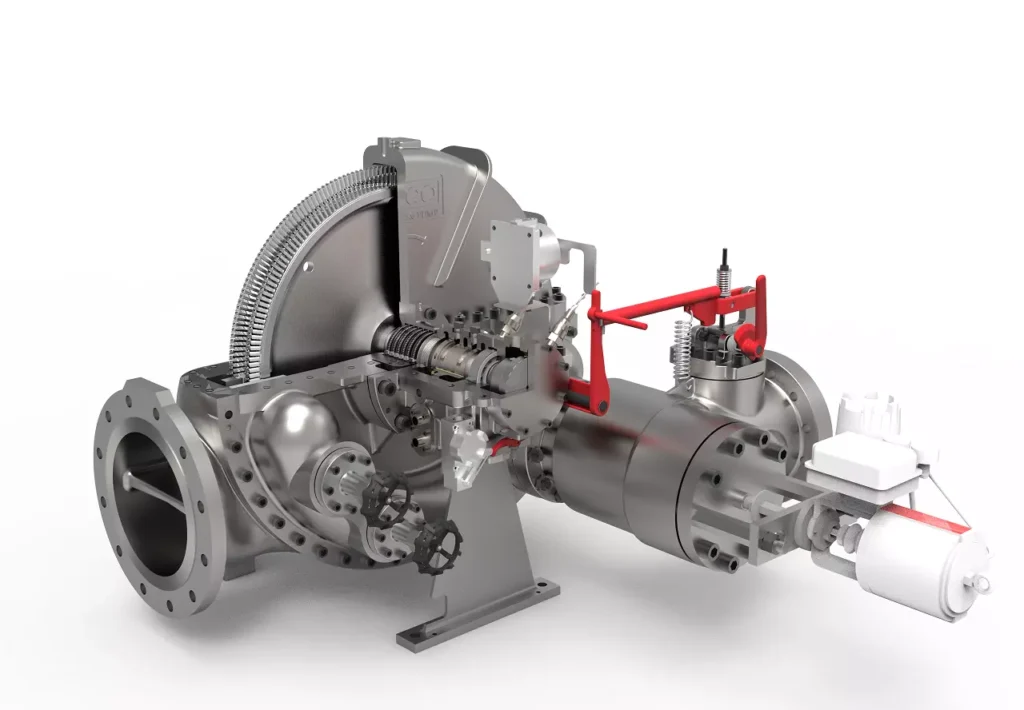
The development of new blade materials and coatings is one of the most exciting areas of innovation in condensing turbines. Advanced materials, such as nickel-based superalloys and ceramic coatings, are allowing turbines to operate at higher temperatures and pressures without suffering from corrosion or erosion. These materials improve the efficiency and durability of turbine blades, reducing the need for frequent maintenance.
Role of Digital Twins and Predictive Analytics
The use of digital twins—virtual models of physical systems—is revolutionizing turbine operation and maintenance. By creating a digital twin of a condensing turbine, operators can simulate different operating conditions and optimize turbine performance without disrupting real-world operations. Predictive analytics tools use data from the digital twin to identify potential performance issues and recommend adjustments, improving turbine efficiency and reliability.
Integration with Renewable and Sustainable Energy Solutions
Condensing turbines are increasingly being integrated into renewable energy systems, such as biomass and geothermal power plants. By using steam generated from renewable sources, these turbines help reduce reliance on fossil fuels and support the transition to cleaner energy. In addition, condensing turbines are being used in conjunction with carbon capture and storage (CCS) technologies to reduce the carbon emissions from fossil-fuel power plants, helping to mitigate climate change.
Future Prospects for Carbon Capture and Storage (CCS)
As the world moves toward reducing carbon emissions, condensing turbines will play a key role in carbon capture and storage (CCS) systems. CCS technology captures carbon dioxide (CO₂) emissions from power plants and industrial facilities and stores them underground, preventing them from entering the atmosphere. Condensing turbines, with their high efficiency and ability to operate at large scales, are well-suited to CCS applications, making them a critical technology in the fight against climate change.
Hybrid Systems and Energy Storage Integration
The future of condensing turbines may also involve greater integration with hybrid energy systems and energy storage technologies. By combining condensing turbines with battery storage or hydrogen production, power plants can store excess energy generated during periods of low demand and release it when demand increases. This approach improves grid stability and allows for more efficient use of renewable energy sources.
Conclusion
Condensing turbines remain a cornerstone of power generation, thanks to their high efficiency and ability to extract maximum energy from steam. From their historical development to their modern applications in fossil-fuel, nuclear, and renewable power plants, condensing turbines continue to evolve with advances in materials, digital technology, and energy storage. Their role in future energy systems, particularly in carbon capture and hybrid energy solutions, will be critical as the world transitions to a more sustainable energy future.
Non-condensing (Back-pressure) Turbines
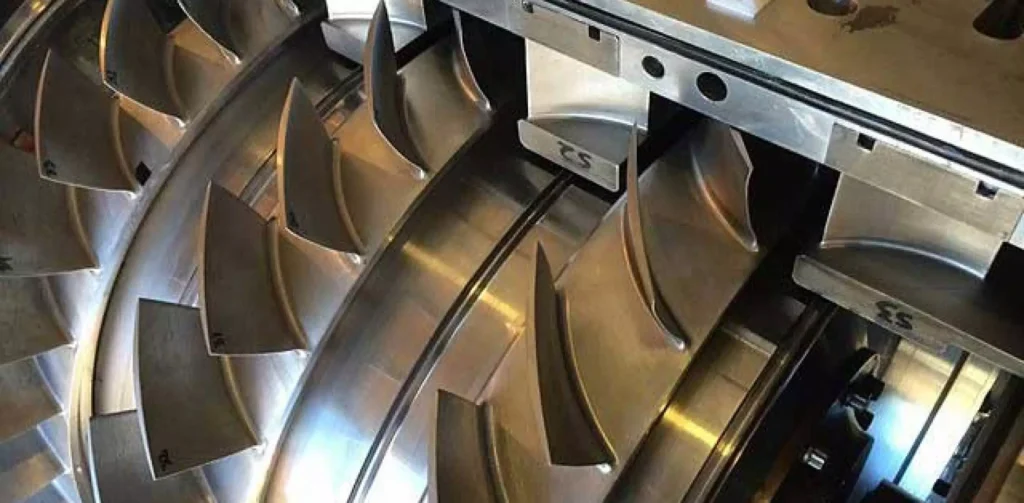
Non-condensing turbines, also known as back-pressure turbines, are a type of steam turbine where the exhaust steam is released at a pressure higher than atmospheric pressure and is used for industrial processes or heating. Unlike condensing turbines, which maximize energy extraction by condensing the steam into water after it passes through the turbine, back-pressure turbines allow the steam to be used in other parts of the plant.
These turbines are typically employed in combined heat and power (CHP) systems, where steam is required for both electricity generation and industrial processes. The “back pressure” refers to the residual pressure of the steam after it has passed through the turbine, which is then used in heating, drying, or mechanical processes in industries such as petrochemicals, food processing, and pulp and paper manufacturing.
Basic Function and Operation
Non-condensing turbines operate by using steam at high pressure to drive a turbine, converting thermal energy into mechanical energy. After passing through the turbine, the steam is exhausted at a lower pressure, but still contains sufficient energy to be used in downstream processes. This configuration allows industries to extract both power and heat from the same steam source, making back-pressure turbines ideal for cogeneration applications, where both power and process heat are required.
Historical Development
The use of steam turbines dates back to the late 19th century when Sir Charles Parsons developed the first practical steam turbine. Non-condensing turbines have evolved alongside their condensing counterparts, finding niche applications in industries that require both steam and power. Over time, advancements in turbine materials, blade design, and thermodynamics have led to more efficient and reliable back-pressure turbines that are crucial to various industrial processes today.
Importance in Industrial Applications
Non-condensing turbines play a vital role in industries where steam is needed for processes like heating, chemical reactions, or mechanical work, as well as electricity generation. They are commonly used in:
- Cogeneration Plants: Producing both electricity and heat for industrial processes.
- District Heating: Providing steam for heating buildings in cities and industrial complexes.
- Industries like Pulp and Paper, Food Processing, and Petrochemicals: Where both power and steam are required for operations.
Their ability to maximize energy efficiency by utilizing the steam for multiple purposes makes them indispensable in sectors aiming to reduce energy costs and environmental impact.
Working Principles of Non-condensing Turbines
The working principle of non-condensing turbines is based on the Rankine cycle, a thermodynamic cycle that describes how heat is converted into mechanical work. In a non-condensing turbine system, water is heated to generate steam, which expands through the turbine, converting thermal energy into mechanical energy. Unlike in condensing turbines, the steam in a back-pressure turbine is not cooled and condensed but is exhausted at a pressure that can be used for other processes.
- Heat Addition: Water is heated in a boiler, converting it into steam.
- Expansion in the Turbine: The high-pressure steam is expanded in the turbine, doing work as it drives the turbine blades.
- Exhaust at Back Pressure: The steam exits the turbine at a higher pressure than in condensing systems, making it useful for industrial processes that require heat.
Role of Pressure and Temperature
The efficiency of a non-condensing turbine is largely dependent on the pressure and temperature of the steam entering and exiting the turbine. The higher the pressure of the incoming steam, the more energy it carries for conversion into mechanical work. The “back pressure,” or exhaust pressure, is usually set to meet the needs of downstream industrial processes, such as heating or chemical reactions.
Energy Conversion: From Steam to Mechanical Work
In a back-pressure turbine, energy is extracted from the steam by converting its thermal energy into mechanical work. As the steam expands through the turbine stages, it loses pressure and temperature, transferring its energy to the turbine rotor, which spins and generates mechanical power. This power can then be used to drive machinery or generate electricity. Unlike condensing turbines, the steam is not fully exhausted; it retains enough energy to be used in other industrial processes.
Comparison with Condensing Turbines
Compared to condensing turbines, non-condensing turbines offer greater efficiency in situations where both steam and power are needed. Condensing turbines focus on maximizing power generation by extracting as much energy as possible from the steam, while back-pressure turbines prioritize process steam for industrial use. The choice between condensing and non-condensing turbines depends on the specific energy and process needs of the facility. In applications where heat and power are required, back-pressure turbines provide a more efficient solution by minimizing wasted energy.
Key Components and Design of Non-condensing Turbines
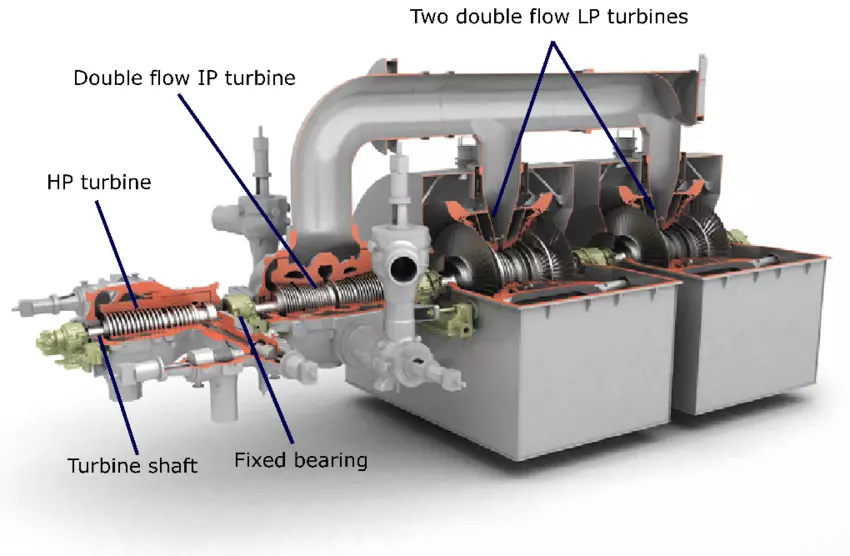
Non-condensing turbines are composed of several key components that ensure their efficient operation and long-term durability:
- Rotor: The central shaft of the turbine, connected to the generator or mechanical system. The rotor converts the energy from steam into rotational mechanical work.
- Blades: Turbine blades extract energy from the steam, causing the rotor to spin. These blades are carefully designed to handle varying steam conditions and are typically made from heat- and corrosion-resistant materials.
- Nozzles: Nozzles direct the flow of high-pressure steam onto the turbine blades, maximizing energy transfer.
- Bearings: Bearings support the rotor and allow it to spin at high speeds with minimal friction. These components are essential for maintaining the turbine’s efficiency and preventing mechanical wear.
- Seals: Seals prevent steam leakage, ensuring that the system operates at maximum efficiency. They also play a role in maintaining pressure balance within the turbine.
Back-pressure Stage Design and Optimization
The design of a back-pressure turbine must account for the specific pressure requirements of the exhaust steam. This means optimizing the turbine stages to ensure that the steam exits at the desired back pressure, while still extracting sufficient energy for mechanical work. The size and shape of the turbine blades are optimized to ensure smooth and efficient steam expansion, minimizing losses due to turbulence and inefficiencies in the flow.
Materials Used in Construction
Non-condensing turbines are built from materials that can withstand high temperatures, pressures, and corrosive environments. Nickel-based alloys and stainless steel are commonly used for turbine blades and rotors due to their ability to maintain strength and resist oxidation at high temperatures. Additionally, specialized coatings may be applied to turbine blades to prevent corrosion and erosion caused by steam impurities.
Blade Design for Performance and Durability
The design of the blades in a non-condensing turbine is critical for optimizing performance and ensuring durability. Blades are shaped to maximize the energy extracted from steam while minimizing the mechanical stress placed on the rotor. Aerodynamic design is essential for reducing turbulence and improving efficiency. Modern blade designs also incorporate materials that resist erosion from wet steam, which can damage the blades over time.
Steam Properties and Their Role in Performance
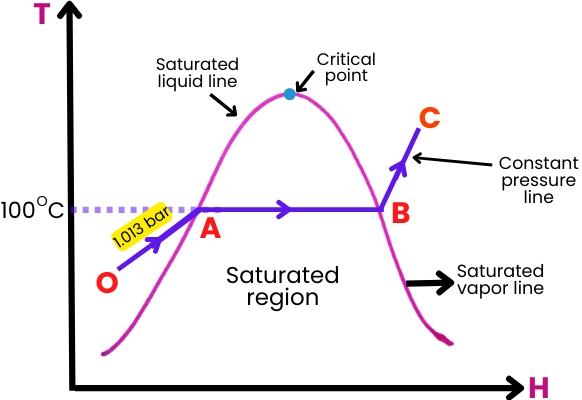
The performance of non-condensing turbines is heavily influenced by the properties of the steam used in the system. Superheated steam is often preferred because it contains more thermal energy and reduces the risk of condensation during expansion. However, saturated steam is sometimes used, depending on the specific requirements of the industrial process.
- Superheated Steam: Steam that has been heated beyond its boiling point at a given pressure. It has a higher energy content and is less likely to condense into water during expansion, which can improve efficiency.
- Saturated Steam: Steam that exists at the boiling point for its pressure. It contains both liquid and vapor phases, which can lead to moisture in the turbine and potential erosion of the blades.
Importance of Pressure and Temperature in Back-pressure Turbines
The pressure and temperature of the steam entering the turbine have a significant impact on the turbine’s performance. Higher-pressure, higher-temperature steam allows for more energy extraction, as the steam carries more thermal energy that can be converted into mechanical work. The back pressure, or exhaust pressure, must be carefully controlled to ensure that the steam can be used effectively in downstream industrial processes.
Managing Steam Quality and Moisture
In non-condensing turbines, maintaining the quality of the steam is essential for ensuring efficiency and minimizing mechanical wear. Wet steam, which contains water droplets, can cause erosion of the turbine blades, reducing efficiency and leading to maintenance issues. To address this, modern turbines use moisture separators or superheating systems to ensure that the steam remains dry throughout the expansion process.
Impact on Efficiency and Equipment Life
The properties of the steam directly influence the efficiency of the turbine and the longevity of its components. Superheated steam tends to increase efficiency by allowing more complete energy extraction, while saturated steam may lead to moisture-related issues. Properly managing steam quality and ensuring that the turbine operates within its design limits helps maximize efficiency and extend the lifespan of the equipment.
Applications of Non-condensing Turbines
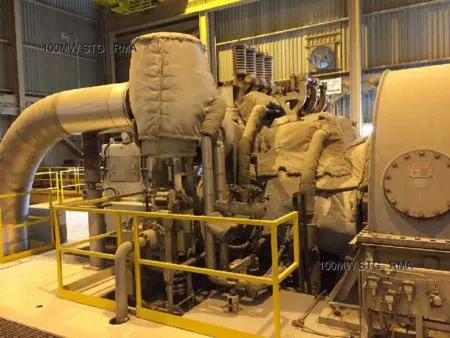
One of the most important applications of non-condensing turbines is in cogeneration, also known as Combined Heat and Power (CHP) systems. In these systems, non-condensing turbines are used to produce both electricity and steam for industrial processes. The exhaust steam from the turbine is used to provide heat for industrial applications, such as drying, heating, or chemical reactions, while the turbine generates electricity for on-site use or distribution to the grid.
Cogeneration is highly efficient because it makes use of both the electrical and thermal energy generated from steam, reducing fuel consumption and lowering overall energy costs. Industries such as pulp and paper, food processing, and chemical manufacturing commonly use cogeneration systems with non-condensing turbines.
Use in Petrochemical Refineries, Pulp and Paper Mills, and Food Processing
Non-condensing turbines are widely used in petrochemical refineries, pulp and paper mills, and food processing plants, where both electricity and steam are needed for industrial processes. In these industries, back-pressure turbines provide a flexible and efficient solution for meeting the energy demands of the plant.
- Petrochemical Refineries: In petrochemical refineries, steam is used to drive compressors, pumps, and other equipment. The exhaust steam from non-condensing turbines is often used for heating or chemical processes within the refinery.
- Pulp and Paper Mills: In the pulp and paper industry, steam is required for both drying paper and generating electricity. Non-condensing turbines allow mills to produce power while also supplying steam for drying processes, improving overall energy efficiency.
- Food Processing: Food processing plants use steam for cooking, sterilization, and drying. Non-condensing turbines generate electricity while providing the steam necessary for these operations, allowing the plant to reduce energy costs and improve operational efficiency.
District Heating Systems
In district heating systems, non-condensing turbines provide steam for heating large industrial complexes, residential areas, or entire cities. The exhaust steam from the turbine is distributed through a network of pipes to provide heating for buildings, reducing the need for individual heating systems. Non-condensing turbines are ideal for district heating applications because they can provide both electricity and heating from a single source of energy, improving the overall efficiency of the system.
Integration in Power Plants and Energy Systems
Non-condensing turbines are used in power plants where both electricity generation and steam for industrial processes are required. In combined-cycle power plants, for example, non-condensing turbines are used alongside gas turbines to maximize efficiency. The gas turbine generates electricity, while the waste heat from the gas turbine is used to produce steam, which is expanded through the non-condensing turbine to generate additional electricity and provide steam for industrial processes.
Renewable Energy: Biomass and Geothermal Applications
Non-condensing turbines are also used in renewable energy applications, particularly in biomass and geothermal power plants. In biomass plants, organic materials such as wood chips, agricultural waste, or dedicated energy crops are burned to produce steam, which is expanded through a non-condensing turbine to generate electricity. The exhaust steam is then used for heating or other industrial processes.
In geothermal power plants, steam is produced by tapping into geothermal reservoirs beneath the Earth’s surface. The steam is expanded through a non-condensing turbine to generate electricity, and the remaining heat can be used for district heating or industrial applications. Non-condensing turbines in renewable energy systems help maximize the use of available resources, improving overall efficiency and reducing environmental impact.
Advantages of Non-condensing Turbines
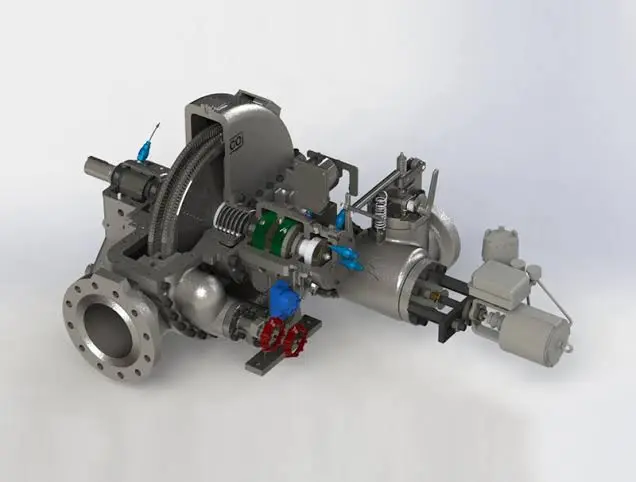
Non-condensing turbines are highly efficient in cogeneration systems because they make use of both the electrical and thermal energy produced from steam. By using the exhaust steam for industrial processes or heating, cogeneration systems with back-pressure turbines achieve higher overall efficiencies compared to systems that generate only electricity. This dual-use of steam reduces fuel consumption and lowers energy costs for industries that require both power and process steam.
Flexibility in Steam and Power Output
One of the key advantages of non-condensing turbines is their flexibility. They can be used in a variety of applications, from generating electricity to providing steam for industrial processes. This flexibility allows industries to optimize their energy use based on their specific needs, whether they require more electricity or more steam. Additionally, the back pressure of the turbine can be adjusted to meet the requirements of downstream processes, ensuring that the system operates at maximum efficiency.
Reduced Environmental Impact
By improving energy efficiency, non-condensing turbines help reduce the environmental impact of industrial processes. Cogeneration systems with non-condensing turbines use less fuel to produce the same amount of energy, resulting in lower carbon dioxide (CO₂) emissions and reduced fuel consumption. In industries where both power and process heat are required, non-condensing turbines offer a more sustainable and cost-effective solution compared to traditional power generation methods.
Operational Benefits and Cost Efficiency
Non-condensing turbines provide several operational benefits, including lower energy costs and improved system efficiency. By generating electricity and process steam from the same steam source, these turbines help industries reduce their reliance on external energy sources, lowering fuel costs and improving operational efficiency. Additionally, the simplicity of non-condensing turbines makes them easier to maintain and operate compared to more complex turbine systems.
Challenges and Limitations
One of the main challenges associated with non-condensing turbines is steam management. The pressure and temperature of the steam must be carefully controlled to ensure that the turbine operates efficiently and that the steam can be used effectively in downstream processes. If the steam pressure is too high or too low, it can reduce the efficiency of the turbine and the performance of the industrial processes that rely on the steam.
Mechanical Wear and Steam Quality
The quality of the steam used in a non-condensing turbine has a significant impact on its performance and durability. Wet steam, which contains water droplets, can cause erosion of the turbine blades, leading to mechanical wear and reduced efficiency. Managing steam quality and ensuring that the steam remains dry throughout the expansion process is essential for maximizing the lifespan of the turbine and maintaining high efficiency.
Efficiency Concerns in Power Generation-only Applications
While non-condensing turbines are highly efficient in cogeneration applications, they are less efficient in power generation-only applications compared to condensing turbines. In power plants where electricity generation is the primary objective, condensing turbines are typically preferred because they can extract more energy from the steam by condensing it into water. In contrast, non-condensing turbines leave some energy in the exhaust steam, which can reduce overall efficiency in power generation applications.
Maintenance and Erosion Risks
Non-condensing turbines are subject to blade erosion from wet steam, which can lead to costly maintenance and reduced efficiency over time. While modern turbines use advanced materials and coatings to reduce the impact of erosion, regular maintenance is still necessary to ensure that the turbine operates efficiently. Managing steam quality and ensuring that the turbine is operating within its design limits can help reduce the risk of erosion and extend the lifespan of the turbine.
Performance Optimization and Efficiency Improvements
Digitalization and Real-time Monitoring Systems
Advances in digitalization are transforming the way non-condensing turbines are monitored and maintained. Modern turbines are equipped with sensors that provide real-time data on temperature, pressure, vibration, and steam quality. This data can be used to optimize turbine performance, identify potential issues before they lead to equipment failure, and improve overall efficiency.
Predictive Maintenance and AI-driven Maintenance Strategies
Predictive maintenance systems use data from sensors to predict when turbine components are likely to fail, allowing operators to perform maintenance before a breakdown occurs. This reduces downtime and improves the reliability of non-condensing turbines. Artificial intelligence (AI) is increasingly being used in these systems to analyze data and recommend maintenance actions, improving the efficiency of maintenance operations and reducing costs.
Design Improvements for Higher Efficiency
Continued improvements in turbine design are helping to increase the efficiency of non-condensing turbines. Advances in blade design, aerodynamics, and materials science have led to turbines that can operate at higher temperatures and pressures, improving the efficiency of energy extraction. By optimizing the design of turbine components and reducing energy losses, modern non-condensing turbines achieve higher efficiencies than older models.
Retrofitting and Upgrading Existing Turbines
Many older non-condensing turbines can be retrofitted with modern technology to improve their efficiency and performance. Retrofitting options include upgrading turbine blades to more aerodynamic designs, installing advanced cooling systems, and integrating digital monitoring tools. These retrofits can extend the lifespan of older turbines and improve their efficiency, reducing the need for new equipment and lowering operating costs.
Future Trends and Innovations in Non-condensing Turbines
Advances in Blade and Turbine Design
The development of new blade materials and designs is one of the most exciting areas of innovation in non-condensing turbines. Advanced materials, such as nickel-based superalloys and ceramic coatings, are allowing turbines to operate at higher temperatures and pressures without suffering from corrosion or erosion. These materials improve the efficiency and durability of turbine blades, reducing the need for frequent maintenance.
Integration with Renewable Energy Systems
Non-condensing turbines are increasingly being integrated into renewable energy systems, such as biomass and geothermal power plants. By using steam generated from renewable sources, these turbines help reduce reliance on fossil fuels and support the transition to cleaner energy. In addition, non-condensing turbines are being used in conjunction with carbon capture and storage (CCS) technologies to reduce the carbon emissions from fossil-fuel power plants, helping to mitigate climate change.
Hybrid Systems and Energy Storage Integration
The future of non-condensing turbines may also involve greater integration with hybrid energy systems and energy storage technologies. By combining non-condensing turbines with battery storage or hydrogen production, power plants can store excess energy generated during periods of low demand and release it when demand increases. This approach improves grid stability and allows for more efficient use of renewable energy sources.
Future Role in Decarbonization and Sustainability Efforts
As the world moves toward reducing carbon emissions, non-condensing turbines will play a key role in decarbonization efforts. By integrating non-condensing turbines into renewable energy systems and combining them with carbon capture technologies, industries can reduce their carbon footprint and transition to more sustainable energy sources. Non-condensing turbines, with their flexibility and efficiency, will continue to be an important part of the energy landscape as industries move toward cleaner and more sustainable energy solutions.
Digital Twin Technology for Simulation and Optimization
The use of digital twins—virtual models of physical systems—is revolutionizing turbine operation and maintenance. By creating a digital twin of a non-condensing turbine, operators can simulate different operating conditions and optimize turbine performance without disrupting real-world operations. Predictive analytics tools use data from the digital twin to identify potential performance issues and recommend adjustments, improving turbine efficiency and reliability.
Conclusion
Non-condensing (back-pressure) turbines remain a vital part of many industries, providing efficient solutions for facilities that require both electricity and process steam. Their role in cogeneration systems, district heating, and industrial processes makes them indispensable in sectors that aim to optimize energy use and reduce operational costs. As industries continue to evolve, non-condensing turbines will play a key role in the transition toward more sustainable and efficient energy systems, particularly in renewable energy applications and hybrid power systems.
With ongoing advancements in turbine design, digitalization, and materials science, non-condensing turbines are poised to become even more efficient and reliable. Their flexibility, cost-effectiveness, and ability to integrate with future energy technologies ensure that they will remain relevant in the future of global energy systems.
Introduction to Extraction and Regenerative Turbines
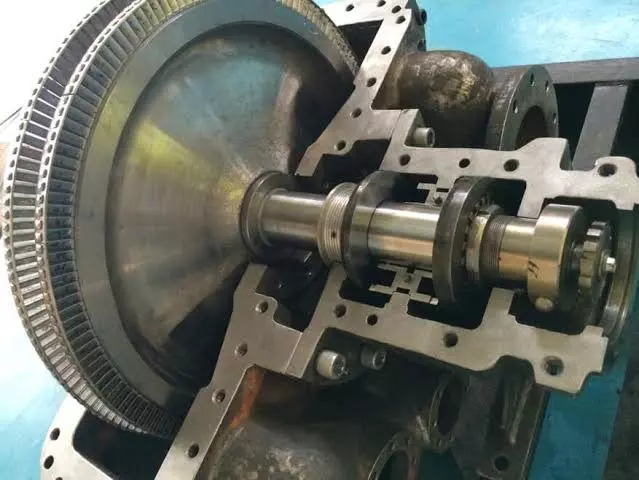
Extraction and regenerative turbines are steam turbines designed to extract steam at intermediate stages for use in industrial processes or for improving the overall efficiency of power plants. The extraction turbine allows steam to be taken out of the turbine at various pressure levels, providing steam for industrial heating or other processes. The regenerative turbine, on the other hand, focuses on increasing efficiency by using extracted steam to preheat the feedwater before it enters the boiler.
Both types of turbines are integral to combined heat and power (CHP) systems and various industrial processes where both electrical power and steam are required. These turbines ensure that steam is efficiently utilized in both power generation and industrial operations, minimizing waste and maximizing energy efficiency.
Importance in Industrial Applications
Extraction and regenerative turbines are widely used in industries like chemical manufacturing, refineries, pulp and paper, and district heating systems. Their ability to meet varying power and steam demands makes them essential for facilities that require process steam and electricity simultaneously. They provide significant economic and environmental advantages by improving fuel efficiency and reducing emissions.
Historical Development and Evolution
The use of steam turbines dates back to the 19th century, with significant innovations by pioneers like Sir Charles Parsons and Gustaf de Laval. The concept of steam extraction for industrial use emerged as industries sought ways to maximize the utilization of steam for both power generation and heating. Over time, the development of regenerative heating in turbines further improved efficiency by reducing the heat required to generate steam, thus conserving fuel and enhancing overall system performance.
Basic Function and Operation
Extraction turbines work by tapping steam at various stages of the expansion process, allowing it to be diverted for industrial or heating processes. The steam is extracted at controlled pressure levels, leaving the remaining steam to continue expanding through the turbine and generating power. Regenerative turbines, on the other hand, use some of the extracted steam to heat the feedwater, reducing the amount of fuel required to generate new steam, thus improving the thermal efficiency of the entire system.
Working Principles of Extraction and Regenerative Turbines
Thermodynamics of Extraction Turbines
Extraction turbines operate based on the Rankine cycle, where steam is generated in a boiler, expanded through the turbine to produce mechanical work, and then condensed back into water. In an extraction turbine, steam is extracted at one or more intermediate points before the final low-pressure stage. This steam can be used for industrial processes, heating, or other applications that require medium or low-pressure steam.
- Heat Addition: Steam is generated in a boiler from feedwater.
- Expansion in the Turbine: High-pressure steam is expanded through the turbine, producing mechanical energy. Steam is extracted at intermediate pressure points for external use.
- Condensation: The remaining steam continues to the low-pressure turbine stage, where it is condensed and returned to the cycle.
The Role of the Rankine Cycle
Both extraction and regenerative turbines operate within the framework of the Rankine cycle. In a regenerative turbine, part of the steam extracted from intermediate stages is used to preheat the feedwater entering the boiler. This process reduces the heat energy required to convert water into steam, improving the overall thermal efficiency of the cycle by recovering waste heat.
Energy Extraction through Multiple Pressure Levels
One of the key features of extraction turbines is their ability to supply steam at different pressure levels. This flexibility is crucial for industries that require steam at various temperatures and pressures for processes such as chemical reactions, drying, or heating. The ability to extract steam at multiple points makes these turbines highly adaptable to different industrial applications.
Regenerative Turbines: Enhancing Efficiency with Feedwater Heating
In regenerative turbines, the extraction of steam is used specifically to preheat the feedwater before it enters the boiler. By raising the temperature of the feedwater, less heat is required to produce steam, which significantly improves the overall efficiency of the power generation process. Regenerative feedwater heating is commonly used in large power plants to enhance efficiency and reduce fuel consumption.
Comparison with Other Types of Steam Turbines
Extraction and regenerative turbines offer unique benefits compared to traditional condensing or non-condensing turbines. While condensing turbines focus on maximizing power output by exhausting steam at very low pressures, extraction turbines prioritize flexibility by allowing steam to be diverted for industrial use. Similarly, regenerative turbines are designed to enhance efficiency by reducing fuel consumption, making them ideal for power plants focused on optimizing energy use.
Design and Key Components of Extraction and Regenerative Turbines
Turbine Structure: Rotor, Blades, Nozzles, and Seals
The core components of extraction and regenerative turbines are similar to those found in conventional steam turbines. These include the rotor, blades, nozzles, and seals, each of which plays a critical role in the turbine’s ability to convert steam energy into mechanical work.
- Rotor: The rotor is the central rotating shaft, which converts the energy from steam into mechanical motion. It is designed to withstand high rotational speeds and the stresses caused by expanding steam.
- Blades: Turbine blades are designed to extract energy from the steam as it expands through the turbine. The blades are carefully engineered to handle high pressures and temperatures while minimizing energy losses due to turbulence.
- Nozzles: Nozzles direct high-pressure steam onto the turbine blades, maximizing energy transfer. In extraction turbines, nozzles also control the flow of steam that is extracted at intermediate stages.
- Seals: Seals prevent steam leakage from the turbine, ensuring that the system operates efficiently.
Extraction Points: Steam Control and Flow Management
In extraction turbines, extraction points are located at various stages of the turbine, allowing steam to be diverted for industrial processes. These points are equipped with valves and control systems to regulate the flow and pressure of the extracted steam, ensuring that the right amount of steam is delivered at the required pressure for downstream processes.
Feedwater Heaters in Regenerative Turbines
Regenerative turbines include feedwater heaters that use extracted steam to preheat the water before it enters the boiler. These heaters are designed to recover waste heat from the turbine, improving the overall efficiency of the steam cycle. Feedwater heaters can be open or closed, depending on the specific design of the power plant.
- Open Feedwater Heaters: Steam and feedwater are mixed directly, allowing the steam to condense and transfer its heat to the water.
- Closed Feedwater Heaters: Steam transfers its heat to the feedwater through heat exchangers, without direct contact.
Material Selection for High-Temperature, High-Pressure Operation
Materials used in extraction and regenerative turbines must withstand high pressures and temperatures, as well as the mechanical stresses caused by steam expansion. Common materials include nickel-based alloys for turbine blades and rotors, which provide excellent resistance to heat and corrosion. Stainless steel and other high-strength alloys are also used to ensure long-term durability and reliability.
Blade Design and Aerodynamics
The design of turbine blades is crucial for maximizing efficiency and minimizing mechanical stress. Engineers use computational fluid dynamics (CFD) to optimize blade shape and aerodynamic profiles, ensuring that the steam flows smoothly over the blades and transfers as much energy as possible. Modern turbine blades are also designed to resist erosion from wet steam, which can cause wear over time.
Steam Properties and Their Role in Performance
The performance of extraction turbines is significantly influenced by the properties of the steam used in the system. Superheated steam, which is steam that has been heated beyond its boiling point, is generally preferred because it contains more energy and reduces the risk of condensation within the turbine. However, saturated steam—which contains both liquid and vapor phases—is also used in some applications, particularly when the extracted steam is required for heating processes that do not require superheated steam.
Steam Pressure, Temperature, and Quality Considerations
The pressure and temperature of the steam entering the turbine have a direct impact on the turbine’s performance and efficiency. High-pressure, high-temperature steam allows for greater energy extraction, as the steam carries more thermal energy that can be converted into mechanical work. The quality of the steam, which refers to the ratio of vapor to liquid, must also be carefully managed to avoid the formation of moisture, which can cause blade erosion and reduce efficiency.
Impact of Moisture Content on Efficiency and Blade Durability
As steam expands through the turbine, its pressure and temperature decrease, which can lead to condensation and the formation of wet steam. Wet steam contains water droplets that can erode the turbine blades, reducing the turbine’s efficiency and leading to maintenance issues. Managing moisture content is crucial in both extraction and regenerative turbines, as excessive moisture can reduce both performance and equipment lifespan.
The Role of Steam Extraction in Managing Steam Quality and Performance
In extraction turbines, steam is often extracted before it reaches the point where it starts to condense, ensuring that the steam remains dry and efficient for industrial processes. By controlling the pressure and temperature of the extracted steam, turbine operators can maintain high efficiency and prevent moisture-related issues in downstream applications.
Industrial Applications of Extraction and Regenerative Turbines
One of the most important applications of extraction and regenerative turbines is in power plants and CHP systems. In these systems, the turbines are used to generate electricity while simultaneously providing steam for industrial heating or other processes. This dual use of steam improves the overall efficiency of the power plant and reduces fuel consumption.
- CHP Systems: Combined heat and power systems use extraction turbines to provide both electricity and process steam. The extracted steam is used for heating, drying, or other industrial applications, while the turbine continues to generate electricity from the remaining steam.
- Power Plants: In large power plants, extraction turbines allow operators to balance power generation with steam extraction, ensuring that the plant operates at maximum efficiency.
Use in Petrochemical Refineries, Chemical Plants, and Manufacturing
Extraction turbines are widely used in petrochemical refineries, chemical plants, and manufacturing facilities where both electricity and steam are needed for industrial processes. These industries require steam at various pressures and temperatures for heating, chemical reactions, and other applications, making extraction turbines an ideal solution for meeting these demands.
- Petrochemical Refineries: In petrochemical refineries, steam is used to drive compressors, pumps, and other equipment. The extracted steam from the turbine is often used for heating or chemical processes within the refinery.
- Chemical Plants: Chemical plants use extraction turbines to provide process steam for chemical reactions and other manufacturing processes, while also generating electricity for on-site use.
- Manufacturing: In manufacturing plants, extraction turbines provide steam for drying, heating, and other processes that require medium or low-pressure steam.
Pulp and Paper Industry: Extraction and Heating Requirements
In the pulp and paper industry, steam is required for both drying paper and generating electricity. Extraction turbines allow mills to produce power while also supplying steam for drying processes, improving overall energy efficiency.
District Heating and Cooling Systems
In district heating and cooling systems, extraction turbines provide steam for heating or cooling buildings in cities and industrial complexes. The steam is extracted at intermediate pressure levels and distributed through a network of pipes to provide heating or cooling, improving the overall efficiency of the system.
Integration with Renewable Energy Sources (Biomass, Geothermal)
Extraction and regenerative turbines are increasingly being integrated into renewable energy systems, particularly in biomass and geothermal power plants. In biomass plants, organic materials such as wood chips, agricultural waste, or dedicated energy crops are burned to produce steam, which is expanded through an extraction turbine to generate electricity. The extracted steam is then used for heating or other industrial processes, improving overall efficiency.
In geothermal plants, steam is produced by tapping into geothermal reservoirs beneath the Earth’s surface. The steam is expanded through a turbine to generate electricity, and the extracted steam is used for district heating or industrial applications.
Advantages of Extraction and Regenerative Turbines
Flexibility in Power and Steam Generation
Extraction and regenerative turbines offer unparalleled flexibility in power and steam generation. They can be used to provide both electricity and process steam at various pressures and temperatures, making them ideal for industries that require both power and heat. This flexibility allows operators to adjust the system based on the specific energy demands of the facility, improving overall efficiency.
Improved Efficiency through Steam Extraction
By extracting steam at intermediate stages, extraction turbines improve the overall efficiency of the steam cycle. The extracted steam is used for industrial processes or heating, reducing the amount of fuel required to produce additional steam. This improves the overall thermal efficiency of the system and reduces fuel consumption.
Economic Benefits in Industrial Cogeneration
In cogeneration systems, extraction turbines provide significant economic benefits by reducing energy costs. By generating both electricity and process steam from the same steam source, industries can reduce their reliance on external energy sources, lowering fuel costs and improving operational efficiency.
Environmental Impact Reduction
The improved efficiency of extraction and regenerative turbines also leads to reduced environmental impact. By reducing fuel consumption, these turbines help lower carbon dioxide (CO₂) emissions and other pollutants, supporting industries in their efforts to meet environmental regulations and reduce their carbon footprint.
Enhanced Heat Recovery and Energy Conservation in Regenerative Turbines
Regenerative turbines offer additional benefits by improving heat recovery and energy conservation. By using extracted steam to preheat the feedwater, regenerative turbines reduce the amount of fuel required to generate steam, improving the overall thermal efficiency of the power plant. This reduces both fuel consumption and emissions, making regenerative turbines an essential part of energy-efficient power generation.
Challenges and Limitations
Complexity of Steam Flow and Control Systems
One of the main challenges associated with extraction and regenerative turbines is the complexity of steam flow and control systems. Extracting steam at various pressure levels requires careful control to ensure that the right amount of steam is delivered to downstream processes without compromising the efficiency of the turbine. Balancing steam extraction and power generation requires sophisticated control systems and monitoring tools.
Balancing Power Output and Steam Extraction
In extraction turbines, balancing power output and steam extraction can be challenging. If too much steam is extracted, the turbine may not generate enough electricity to meet the plant’s needs. Conversely, if not enough steam is extracted, the industrial processes may not receive the required amount of steam. Achieving the right balance between power and steam generation requires careful planning and system design.
Risk of Erosion from Wet Steam and Moisture
The formation of wet steam in extraction turbines can lead to blade erosion, reducing the efficiency of the turbine and leading to costly maintenance. Managing moisture content in the steam and ensuring that the turbine operates within its design limits can help reduce the risk of erosion and extend the lifespan of the turbine.
Maintenance and Reliability Issues in High-Pressure Systems
Extraction and regenerative turbines operate at high pressures and temperatures, which can lead to mechanical wear and tear over time. Regular maintenance is required to ensure that the turbine operates efficiently and reliably. In high-pressure systems, maintenance challenges can be more pronounced, as the equipment is subject to higher mechanical stresses.
Efficiency Losses in Non-ideal Operating Conditions
The efficiency of extraction and regenerative turbines can be affected by non-ideal operating conditions, such as fluctuations in steam pressure, temperature, or demand. Ensuring that the turbine operates under optimal conditions requires sophisticated control systems and real-time monitoring, which can be challenging to implement and maintain.
Performance Optimization and Efficiency Improvements
Digitalization and Real-time Monitoring
Advances in digitalization and real-time monitoring are transforming the way extraction and regenerative turbines are operated and maintained. Modern turbines are equipped with sensors that provide real-time data on temperature, pressure, vibration, and steam quality. This data can be used to optimize turbine performance, identify potential issues before they lead to equipment failure, and improve overall efficiency.
AI-driven Predictive Maintenance and System Optimization
Artificial intelligence (AI) is increasingly being used to analyze data from turbines and recommend maintenance actions. Predictive maintenance systems use data from sensors to predict when components are likely to fail, allowing operators to perform maintenance before a breakdown occurs. This reduces downtime and improves the reliability of extraction and regenerative turbines.
Advanced Blade Materials and Coatings
The development of new blade materials and coatings is helping to improve the efficiency and durability of extraction and regenerative turbines. Advanced materials, such as nickel-based superalloys and ceramic coatings, allow turbines to operate at higher temperatures and pressures without suffering from corrosion or erosion. These materials improve the efficiency of the turbine and reduce the need for frequent maintenance.
Retrofitting Older Turbines for Efficiency Gains
Many older extraction and regenerative turbines can be retrofitted with modern technology to improve their efficiency and performance. Retrofitting options include upgrading turbine blades to more aerodynamic designs, installing advanced control systems, and integrating digital monitoring tools. These retrofits can extend the lifespan of older turbines and improve their efficiency, reducing the need for new equipment and lowering operating costs.
Techniques for Improving Feedwater Heating Efficiency in Regenerative Turbines
In regenerative turbines, the efficiency of feedwater heating can be improved by optimizing the design of feedwater heaters and heat exchangers. Modern regenerative systems use advanced designs that maximize heat transfer while minimizing pressure losses, improving the overall efficiency of the steam cycle.
Future Trends and Innovations in Extraction and Regenerative Turbines
Integration with Smart Grids and Hybrid Power Systems
The future of extraction and regenerative turbines will involve greater integration with smart grids and hybrid power systems. By connecting turbines to smart grids, operators can optimize energy production based on real-time demand, improving efficiency and reducing energy waste. Hybrid power systems that combine extraction turbines with battery storage or renewable energy sources will allow for more flexible and efficient energy management.
Digital Twins for Predictive Analytics and Turbine Management
The use of digital twins—virtual models of physical systems—is revolutionizing turbine operation and maintenance. By creating a digital twin of an extraction or regenerative turbine, operators can simulate different operating conditions and optimize turbine performance without disrupting real-world operations. Predictive analytics tools use data from the digital twin to identify potential performance issues and recommend adjustments, improving turbine efficiency and reliability.
Role in Carbon Capture and Storage (CCS) Systems
Extraction and regenerative turbines will play a key role in carbon capture and storage (CCS) systems, which aim to reduce carbon emissions from fossil-fuel power plants. CCS technology captures carbon dioxide (CO₂) emissions from power plants and industrial facilities and stores them underground, preventing them from entering the atmosphere. Extraction turbines, with their ability to provide steam for CCS processes, will be an integral part of this technology.
Advances in Blade Design, Materials, and 3D Printing
The development of new blade designs and materials is helping to improve the efficiency and performance of extraction and regenerative turbines. 3D printing technology is being used to create complex, high-precision parts that were previously difficult or impossible to manufacture using traditional methods. This allows for the optimization of blade designs, leading to higher efficiency and reduced manufacturing costs.
Sustainable Energy Solutions: Biomass, Geothermal, and Solar Integration
Extraction and regenerative turbines are increasingly being integrated into sustainable energy solutions, such as biomass, geothermal, and solar power plants. By using steam generated from renewable sources, these turbines help reduce reliance on fossil fuels and support the transition to cleaner energy. In addition, extraction turbines are being used in conjunction with solar thermal systems to generate electricity and provide heating, improving the overall efficiency of solar power plants.
Conclusion
Extraction and regenerative turbines remain a vital part of many industries, providing efficient solutions for facilities that require both electricity and process steam. Their role in cogeneration systems, district heating, and industrial processes makes them indispensable in sectors that aim to optimize energy use and reduce operational costs. As industries continue to evolve, extraction and regenerative turbines will play a key role in the transition toward more sustainable and efficient energy systems, particularly in renewable energy applications and hybrid power systems.
With ongoing advancements in turbine design, digitalization, and materials science, extraction and regenerative turbines are poised to become even more efficient and reliable. Their flexibility, cost-effectiveness, and ability to integrate with future energy technologies ensure that they will remain relevant in the future of global energy systems.
To write a comprehensive 10,000-word document on Impulse vs. Reaction Turbines, this content will cover their definitions, working principles, design differences, applications, performance, advantages, challenges, and future trends. This detailed outline provides a logical and structured approach to understanding the two types of turbines, highlighting how they are similar, how they differ, and where each is best used.
Impulse vs. Reaction Turbines
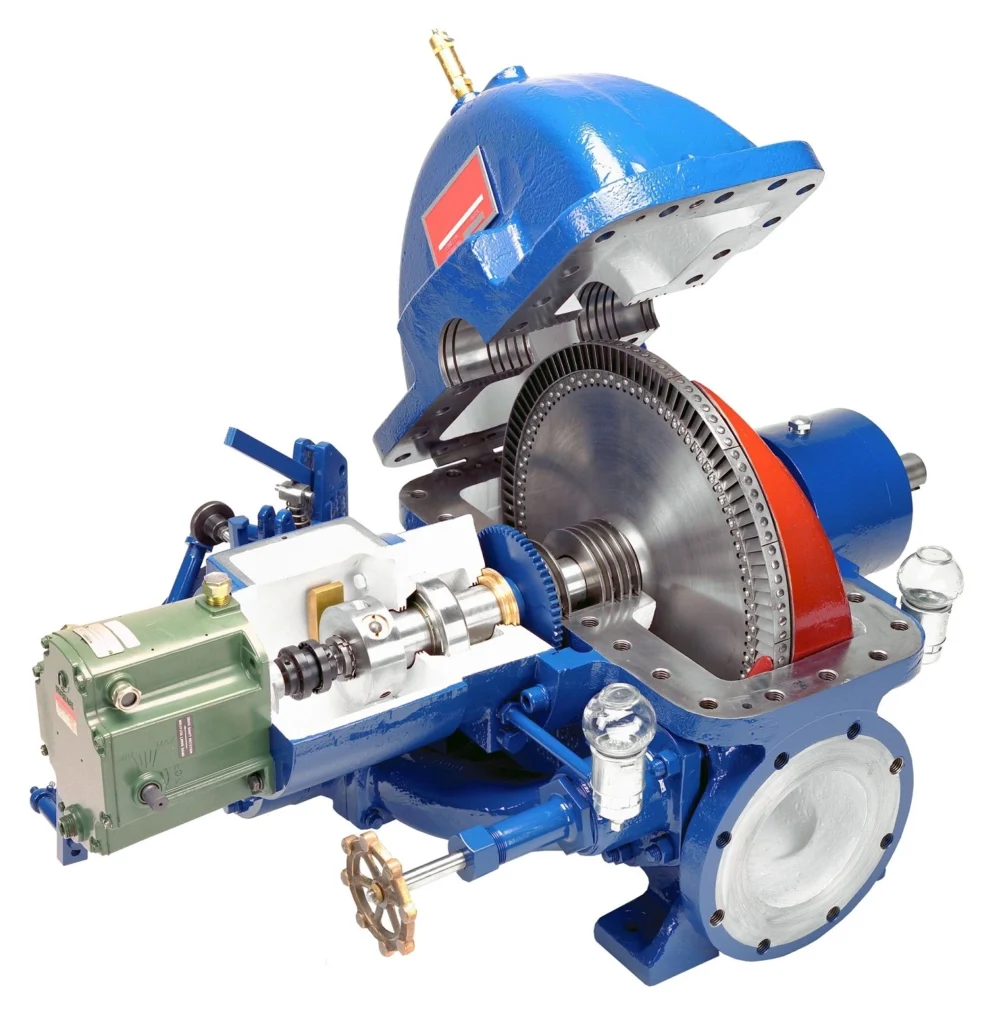
Steam turbines are devices that convert the thermal energy in steam into mechanical work, which can then be used to generate electricity or drive mechanical equipment. They are integral components in power plants, industrial facilities, and marine propulsion systems. The two primary types of steam turbines are impulse turbines and reaction turbines, each with distinct characteristics and applications.
Significance of Impulse and Reaction Turbines in Industry
Both impulse and reaction turbines play crucial roles in energy generation and mechanical power applications worldwide. Understanding their differences is key to optimizing efficiency, performance, and cost-effectiveness in various industrial settings. While impulse turbines are often used where high-pressure, low-flow steam is required, reaction turbines are better suited to scenarios where low-pressure, high-flow steam is advantageous.
Purpose of the Comparison
This document aims to provide a detailed comparison between impulse and reaction turbines, exploring their principles, designs, and applications. By examining the strengths and weaknesses of each type, readers will gain a comprehensive understanding of which turbine is best suited for specific uses and why.
Basic Definitions
- Impulse Turbines: These turbines convert kinetic energy from high-velocity steam jets into mechanical energy. The steam passes through nozzles that accelerate it, and the resulting high-speed jets hit the turbine blades, causing the rotor to spin.
- Reaction Turbines: In these turbines, steam expands continuously as it passes over the blades, generating thrust that drives the rotor. The blades themselves act as nozzles, allowing for smooth and continuous energy conversion.
Steam turbines are mechanical devices that convert the thermal energy in steam into mechanical energy, which is then used to drive generators for electricity production or power industrial machinery. Steam turbines have been a cornerstone of industrial power generation since the late 19th century and continue to play a crucial role in various applications today, from large power plants to marine propulsion systems.
These machines operate on the principle of the Rankine cycle, where water is heated, converted into steam, expanded through a turbine to produce work, and then condensed back to water to complete the cycle. By effectively harnessing the energy of high-pressure steam, steam turbines have enabled significant advancements in electricity generation and mechanical power.
Steam turbines are categorized based on how they extract energy from steam, and two of the most prominent types are impulse turbines and reaction turbines. Understanding the differences between these two types is essential for selecting the right equipment for a given application, as each has its own unique advantages, operational characteristics, and optimal usage scenarios.
Importance of Impulse and Reaction Turbines in Power Generation
Both impulse and reaction turbines are essential for efficient power generation and mechanical power applications around the world. These turbines are used in thermal power plants, nuclear power stations, geothermal plants, industrial plants, and marine vessels, among other settings. Their ability to convert the energy of steam into mechanical work with high efficiency makes them indispensable in industries where reliable and efficient energy conversion is a priority.
Impulse turbines and reaction turbines are designed to handle different types of steam conditions and flow rates. While impulse turbines are known for their ability to handle high-pressure, low-flow steam conditions, reaction turbines excel in environments where low-pressure, high-flow steam is available. Each turbine type has its own set of characteristics, which makes it more suitable for certain applications. For example:
- Impulse turbines are often used in situations where steam enters at high pressure and needs to be expanded quickly to produce mechanical energy. They are typically found in smaller, high-pressure steam systems, such as in certain sections of thermal power plants and marine engines.
- Reaction turbines, on the other hand, are better suited for applications where steam flow is continuous, and gradual energy extraction is desired. They are commonly used in large-scale power plants and situations where low-pressure, high-flow steam must be efficiently utilized.
Understanding when to use impulse versus reaction turbines can significantly impact the efficiency, cost, and reliability of a power generation system. This makes the comparison between the two turbine types crucial for engineers, operators, and decision-makers.
Purpose of the Comparison
The primary purpose of this comparison is to provide a detailed analysis of impulse turbines and reaction turbines, focusing on their design, working principles, applications, efficiency, and performance. By examining the strengths, weaknesses, and unique characteristics of each type, this document aims to guide readers in understanding which turbine type is best suited for specific industrial and power generation scenarios.
This analysis will explore various factors, including:
- Mechanics and Thermodynamics: How each turbine type converts steam energy into mechanical energy.
- Design Considerations: Differences in blade structure, nozzle arrangement, and stage configuration.
- Efficiency Metrics: Performance of each turbine under varying operating conditions.
- Applications: Real-world examples of where each turbine type is most effectively used.
- Cost and Maintenance: Operational costs, maintenance requirements, and reliability issues.
- Technological Developments: Recent advances and future trends in turbine design and operation.
By the end of this document, readers will have a comprehensive understanding of impulse and reaction turbines, which will help them make informed decisions about turbine selection and operation in various industrial contexts.
Basic Definitions and Concepts
To begin, it’s essential to understand the basic definitions of impulse and reaction turbines, as well as the concepts that differentiate them:
- Impulse Turbines:
- In impulse turbines, steam is directed through nozzles that convert its pressure energy into high-velocity jets. These jets strike the blades of the turbine, causing the rotor to spin. The key characteristic of an impulse turbine is that the pressure drop (and therefore energy conversion) occurs entirely in the nozzles, and not on the blades. This means that the blades themselves only absorb kinetic energy, and there is no pressure change as steam flows over them.
- Reaction Turbines:
- Reaction turbines work on a different principle where steam undergoes continuous expansion as it passes over the blades. Here, the blades are shaped to act as nozzles themselves, allowing steam to expand and accelerate while flowing through the turbine. This causes a pressure drop across both the stator (fixed blades) and the rotor (moving blades), with energy being extracted from both. The main characteristic of reaction turbines is that both pressure and velocity changes occur as steam passes through the turbine blades, leading to a more gradual and continuous energy conversion process.
- Key Differences:
- Impulse turbines rely on discrete high-velocity steam jets for energy conversion, and the energy transfer happens primarily due to the impact of these jets on the turbine blades.
- Reaction turbines operate on the principle of a continuous pressure drop and expansion of steam across the turbine, with energy extracted from the reaction forces generated by this process.
Understanding these fundamental differences is crucial to appreciating why each turbine design is used in specific scenarios and how they can be optimized for maximum efficiency. The sections that follow will delve deeper into the working principles, design features, and applications of both impulse and reaction turbines, providing a thorough comparison that highlights their unique characteristics.
History and Evolution of Impulse and Reaction Turbines
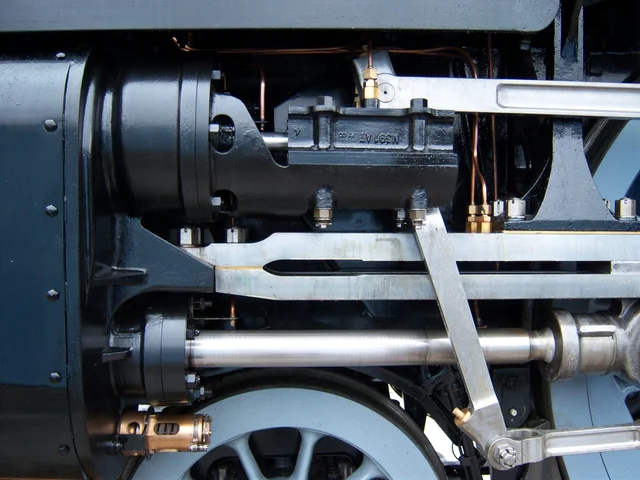
The evolution of steam turbines began in the late 19th century as industries sought more efficient ways to convert heat energy into mechanical work. Before steam turbines, steam engines were the predominant method of harnessing steam power. However, they were relatively inefficient, bulky, and required significant maintenance. The development of steam turbines marked a turning point in the field of mechanical engineering, leading to significant advancements in efficiency and reliability.
The basic concept of using steam to generate mechanical energy dates back to ancient times, with early designs such as the Aeolipile, invented by Hero of Alexandria in the 1st century AD. This device used steam to create rotary motion, but it was more of a novelty than a practical engine. It wasn’t until the Industrial Revolution that steam power became integral to industries, primarily through the use of steam engines. However, it was the innovation of steam turbines that truly revolutionized power generation.
Inventions by Charles Parsons, Gustaf de Laval, and Others
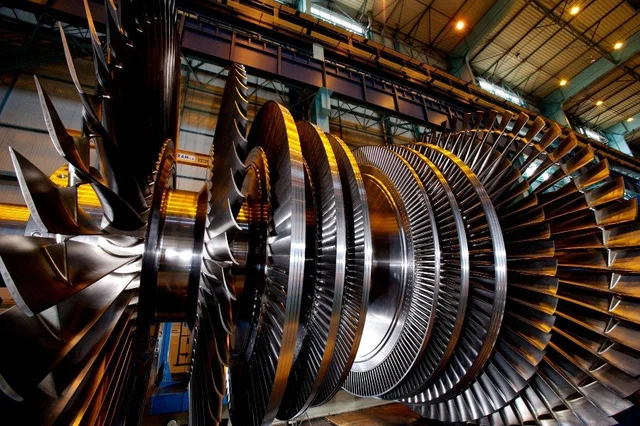
The modern steam turbine emerged in the late 1800s, thanks to the pioneering work of inventors like Sir Charles Parsons and Gustaf de Laval, who laid the foundations for the impulse and reaction turbine designs, respectively.
- Gustaf de Laval and the Impulse Turbine:
- In 1883, Swedish engineer Gustaf de Laval developed one of the first impulse turbines, which was initially used for driving cream separators. De Laval’s design utilized high-velocity steam jets directed onto a set of blades attached to a rotating disc. The key innovation was the use of nozzles to accelerate steam to high speeds, converting its thermal energy into kinetic energy before it impacted the turbine blades.
- This design was simple, effective, and could operate at extremely high speeds, making it suitable for small-scale applications. De Laval’s impulse turbine could reach speeds of up to 30,000 RPM, which was unprecedented at the time. It demonstrated that steam turbines could be more efficient and reliable than traditional steam engines.
- Sir Charles Parsons and the Reaction Turbine:
- Sir Charles Parsons, a British engineer, is credited with inventing the first practical reaction turbine in 1884. Parsons’ design was revolutionary because it allowed for a gradual expansion of steam across multiple stages, leading to continuous energy extraction. Unlike de Laval’s impulse turbine, which relied on high-velocity steam jets, Parsons’ reaction turbine used a combination of stationary and moving blades that allowed steam to expand and transfer energy continuously.
- Parsons’ turbine was initially used to drive electrical generators, and it marked the beginning of the widespread use of turbines for electricity generation. His turbine was capable of operating at much lower speeds than de Laval’s, but it was far more efficient in converting steam energy into mechanical work, especially for larger applications. Parsons’ design became the standard for power generation in thermal plants, and its principles are still used in modern turbines.
- Further Innovations and Hybrid Designs:
- Following the pioneering work of de Laval and Parsons, numerous engineers and inventors contributed to the advancement of steam turbine technology. The early 20th century saw the development of multi-stage turbines, which combined the principles of impulse and reaction designs. These hybrid turbines utilized impulse stages at the high-pressure end, where steam enters at high velocity, and reaction stages at the low-pressure end, where continuous expansion was more efficient.
- These innovations allowed turbines to operate across a wider range of pressures and temperatures, making them suitable for various industrial applications. The flexibility of combining impulse and reaction stages led to the development of highly efficient compound turbines, which became standard in power plants worldwide.
Evolution of Impulse and Reaction Turbines Over Time
The development of steam turbines has continued to evolve, driven by the need for greater efficiency, reliability, and adaptability. Both impulse and reaction turbines have undergone significant improvements since their inception, with advancements in materials, engineering design, and thermodynamics contributing to their performance.
- Advancements in Materials and Manufacturing:
- Early turbines were limited by the materials available, which often could not withstand high temperatures and pressures. Advances in metallurgy, particularly the development of alloy steels and later superalloys, enabled turbines to operate at much higher temperatures and pressures without succumbing to mechanical stress or thermal fatigue.
- The use of advanced manufacturing techniques, such as precision casting and additive manufacturing (3D printing), has further enhanced the design of turbine blades and rotors. These advancements have allowed for more complex and aerodynamically optimized blade shapes, which improve efficiency and reduce energy losses.
- Development of Multi-stage and Compounding Designs:
- The introduction of multi-stage turbines was a game-changer in steam turbine technology. In early single-stage impulse turbines, steam was expanded in one step, which often led to significant energy losses. Multi-stage turbines, by contrast, allowed for steam to be expanded gradually across multiple stages, with each stage extracting a portion of the energy. This approach greatly improved the overall efficiency of the turbine.
- Compound turbines that combine impulse and reaction stages are now common in many power plants. By leveraging the strengths of both designs, these turbines can handle varying pressure conditions and optimize energy extraction at different stages of the steam expansion process.
- Thermodynamic Efficiency Improvements:
- Over the years, engineers have developed ways to improve the thermodynamic efficiency of turbines. One such method is the reheat cycle, where steam is partially expanded in the turbine, reheated in a boiler, and then expanded further. This process reduces moisture content and increases the average temperature of the heat input, which improves efficiency.
- Regenerative feedwater heating is another technique used to enhance efficiency. In this process, steam is extracted from the turbine at various stages and used to preheat the feedwater before it enters the boiler. This reduces the energy required to convert water into steam, leading to better overall cycle efficiency. Both impulse and reaction turbines can be integrated with these thermodynamic improvements.
Technological Milestones and Improvements
The continued evolution of impulse and reaction turbines has been marked by several key technological milestones, which have expanded their applications and enhanced their performance:
- Blade Cooling Technologies:
- In high-temperature applications, turbine blades can be subject to extreme thermal stress. To address this, engineers developed blade cooling technologies that allow turbines to operate at higher temperatures without damaging the blades. Impulse and reaction turbines have benefited from these innovations, enabling them to operate more efficiently in high-temperature environments like gas-fired and nuclear power plants.
- Computational Fluid Dynamics (CFD) and Blade Design:
- The use of computational fluid dynamics (CFD) has revolutionized the design of turbine blades. CFD allows engineers to simulate the flow of steam through the turbine and optimize the shape of the blades for maximum efficiency. This has led to the development of more aerodynamic blades that minimize energy losses due to turbulence and improve the overall performance of both impulse and reaction turbines.
- Digitalization and Smart Monitoring:
- Modern turbines are equipped with smart monitoring systems that collect real-time data on temperature, pressure, and mechanical performance. This data is used to optimize turbine operation, predict maintenance needs, and prevent failures. The integration of digital technology has made turbines more reliable and easier to maintain, reducing downtime and extending the lifespan of equipment.
- Predictive maintenance and digital twins are examples of how digital technology is being applied to improve turbine performance. Digital twins are virtual models of physical turbines that allow operators to test different operating conditions and optimize performance without disrupting actual operations.
- Integration with Renewable Energy Sources:
- Impulse and reaction turbines have found new applications in renewable energy systems, such as biomass, geothermal, and solar thermal power plants. These turbines can be adapted to utilize steam generated from renewable sources, helping to reduce carbon emissions and support the transition to cleaner energy.
- The adaptability of impulse and reaction designs has made them essential components in hybrid energy systems, where they can work alongside other technologies, such as gas turbines and wind power, to provide stable and reliable energy output.
Conclusion of Historical Evolution
The history of impulse and reaction turbines is a testament to the ingenuity and innovation of engineers who sought to harness the power of steam in the most efficient way possible. From the early designs of de Laval and Parsons to the sophisticated multi-stage, digitally optimized turbines of today, the development of these machines has been driven by the need for higher efficiency, reliability, and adaptability.
Today, impulse and reaction turbines continue to be refined and improved, ensuring they remain essential tools in power generation and industrial processes around the world. Understanding their historical evolution helps engineers appreciate the technological advancements that make modern turbines so effective and highlights the potential for future innovations in turbine technology.
Working Principles of Impulse Turbines
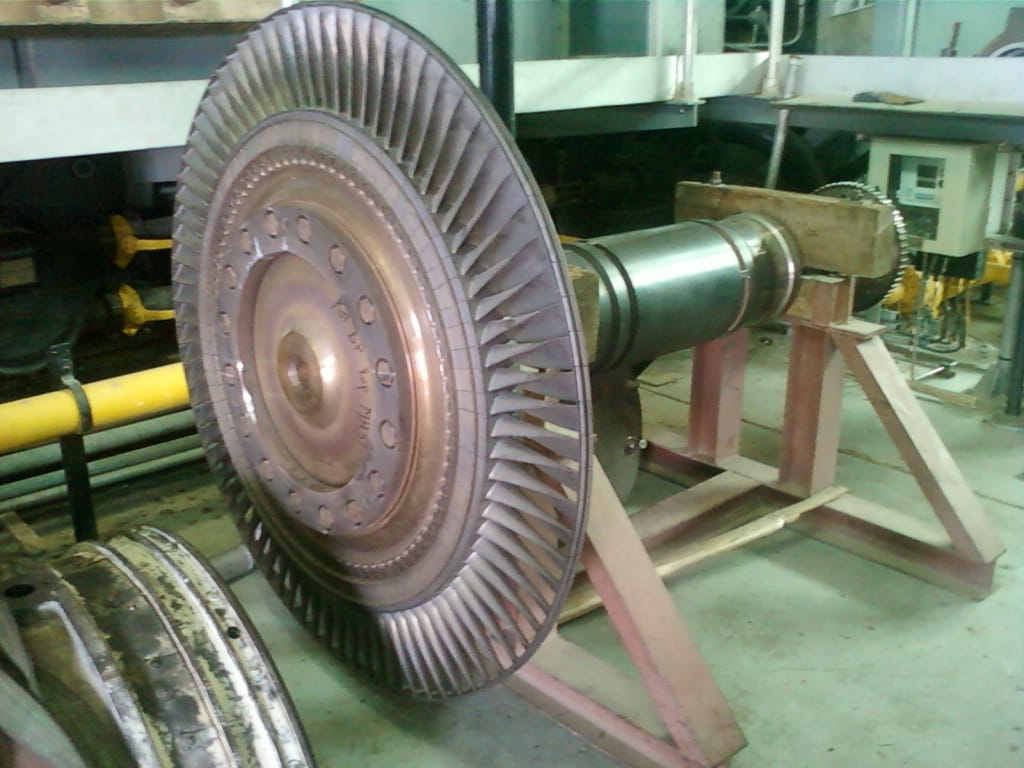
Impulse turbines operate based on the principle of impulse, where high-pressure steam is converted into high-velocity jets, which then strike the turbine blades, causing the rotor to spin. The core idea behind impulse turbines is that the energy transfer happens solely through the kinetic energy of the steam jets, rather than through a pressure difference across the turbine blades.
- The Role of Nozzles:
- In an impulse turbine, steam from the boiler is directed into a set of nozzles. The purpose of these nozzles is to convert the pressure energy of the steam into kinetic energy by accelerating the steam to a high velocity. The nozzles are designed to create a focused jet of steam that moves at a very high speed.
- The design of the nozzles is crucial because it determines how efficiently the turbine can convert steam energy into mechanical work. The nozzles are typically shaped to expand the steam as it exits, causing the pressure to drop and the velocity to increase. This process is known as isentropic expansion, meaning that the steam expands without any heat exchange, ensuring that the conversion from pressure to kinetic energy is efficient.
- High-Velocity Steam Jets and Blade Interaction:
- Once the steam exits the nozzles, it is directed toward the turbine blades. These blades are mounted on a rotating disc, known as the rotor, and are positioned in a way that they intercept the high-velocity steam jets. As the steam hits the blades, it transfers its kinetic energy to them, causing the rotor to spin.
- The blades of an impulse turbine are typically bucket-shaped or cup-shaped to efficiently capture the energy of the steam jets. The design ensures that the steam is redirected as it leaves the blades, which helps in maintaining the momentum and minimizing energy loss. The force exerted by the steam on the blades is what drives the turbine, converting the kinetic energy of the steam into rotational mechanical energy.
- No Pressure Change Across the Blades:
- A defining characteristic of impulse turbines is that the pressure drop occurs only in the nozzles, not across the blades. This means that by the time the steam reaches the blades, it is already at a lower pressure but a much higher velocity. The blades are designed only to capture and redirect this high-velocity steam without causing any further pressure drop.
- This aspect makes impulse turbines suitable for situations where steam enters at very high pressure and needs to be expanded quickly. Since there is no pressure change across the blades, the turbine can operate efficiently without needing a complex blade design to manage pressure variations.
Energy Conversion: Kinetic to Mechanical Energy
Impulse turbines convert the kinetic energy of steam into mechanical energy through the following process:
- Steam Acceleration and Expansion:
- The high-pressure steam from the boiler is first expanded through the nozzles, which causes a drop in pressure and an increase in velocity. The nozzles effectively convert the enthalpy (heat content) of the steam into kinetic energy, producing a jet of steam that is much faster and has a lower pressure than the original steam.
- The velocity of the steam can be exceptionally high, often reaching speeds of up to 1,200 meters per second (m/s) or more. This rapid acceleration is what allows the turbine to extract a significant amount of energy from the steam.
- Impulse Force on Blades:
- As the high-velocity steam strikes the turbine blades, it imparts an impulse force to them. The design of the blades ensures that the steam is redirected efficiently, allowing for maximum energy transfer. The steam jets exert a force on the blades due to the change in momentum, and this force causes the rotor to spin.
- The force exerted by the steam on the blades can be described using Newton’s Second Law of motion, which states that the force is equal to the rate of change of momentum. In the case of impulse turbines, the change in direction and speed of the steam as it strikes the blades results in a significant transfer of energy to the rotor.
- Conversion to Mechanical Work:
- The spinning rotor can be connected to a generator or any mechanical device that needs to be powered. As the rotor turns, it converts the kinetic energy of the steam into mechanical work, which can then be used to generate electricity, drive compressors, or power pumps, depending on the application.
- Impulse turbines are particularly efficient at handling high-speed, high-pressure steam, making them ideal for power generation in situations where steam can be expanded rapidly to create high-velocity jets.
Thermodynamics and the Rankine Cycle in Impulse Turbines
Impulse turbines are an integral part of the Rankine cycle, which is a thermodynamic cycle used to describe the conversion of heat energy into mechanical work. The Rankine cycle consists of four main processes:
- Isobaric Heat Addition (Boiler):
- In the boiler, water is heated under constant pressure until it becomes superheated steam. This steam is stored at high pressure and temperature, ready to be expanded through the turbine.
- Isentropic Expansion (Turbine):
- The high-pressure steam enters the nozzles of the impulse turbine, where it expands isentropically (without heat exchange), converting pressure energy into kinetic energy. The steam then strikes the blades, transferring its kinetic energy to the rotor and causing it to spin.
- During this process, the steam’s pressure drops significantly, and most of its energy is converted into mechanical work. Because impulse turbines only involve a pressure drop in the nozzles, they allow for efficient conversion of steam energy into rotational motion.
- Isobaric Heat Rejection (Condenser):
- After passing through the turbine, the steam is directed into a condenser, where it cools down and condenses back into water. This process is necessary to maintain the flow of steam through the system and to recycle the water for continuous use.
- Isentropic Compression (Pump):
- The condensed water is pumped back into the boiler at high pressure, completing the cycle. The pump raises the pressure of the water without significantly increasing its temperature, preparing it to be reheated and converted back into steam.
Impulse turbines play a critical role in the isentropic expansion part of the Rankine cycle, where they convert the steam’s thermal and pressure energy into mechanical energy. Their design ensures that the process is efficient, with minimal energy losses during the conversion.
Multi-stage Impulse Turbines: Compounding and Efficiency
To improve efficiency and allow for better control of steam expansion, many modern impulse turbines are designed with multiple stages, known as compounding. This approach involves dividing the steam expansion process into several smaller steps, with each step extracting a portion of the energy from the steam.
- Why Compounding is Necessary:
- In a single-stage impulse turbine, expanding all the steam in one go would result in extremely high steam velocities, which can lead to mechanical inefficiencies and excessive wear on the turbine blades. It would also mean a significant loss of energy as the steam exits the turbine at a high velocity, leading to wasted kinetic energy.
- Compounding addresses this issue by dividing the steam expansion into multiple stages, where each stage consists of a nozzle and a set of blades. This gradual reduction in steam pressure and velocity across several stages allows for more efficient energy extraction and reduces wear on the turbine components.
- Types of Compounding:
- Velocity Compounding: In this design, high-velocity steam from the nozzles is directed through multiple rows of moving and stationary blades. The moving blades absorb energy from the steam, while the stationary blades redirect the flow to ensure it hits the next row of moving blades effectively. This helps to manage extremely high steam velocities and makes the turbine more efficient.
- Pressure Compounding: Pressure compounding divides the pressure drop into multiple stages, with each stage having its own set of nozzles and blades. This approach reduces the pressure gradually, ensuring that the steam does not expand too rapidly and allowing for smoother, more controlled energy extraction.
- Combination of Both: Modern impulse turbines often use a combination of both velocity and pressure compounding to achieve optimal performance. This design ensures that steam expansion is gradual and efficient, leading to improved energy conversion rates and lower mechanical wear.
- Efficiency Gains:
- Multi-stage impulse turbines are far more efficient than their single-stage counterparts, as they allow for greater control over the expansion process. By managing the steam pressure and velocity across multiple stages, these turbines can achieve higher isentropic efficiency, meaning they convert a greater percentage of the steam’s energy into useful mechanical work.
- Compounded turbines also help to reduce the exit velocity of the steam, minimizing energy losses as the steam leaves the turbine. This means more of the energy generated in the boiler is used for productive work, rather than being lost as waste heat.
Conclusion on Impulse Turbine Principles
Impulse turbines are highly efficient machines that convert the kinetic energy of high-velocity steam jets into mechanical work. By focusing on accelerating steam through nozzles and then using that kinetic energy to drive turbine blades, they effectively harness the power of steam without relying on pressure differences across the blades. This design makes them ideal for high-pressure applications where rapid energy conversion is needed.
The use of multi-stage designs and compounding has further enhanced the efficiency of impulse turbines, making them suitable for a wide range of applications, from power generation to mechanical drives. Understanding the working principles of impulse turbines helps engineers and operators optimize their performance, ensuring they are used effectively in various industrial and energy settings.
Working Principles of Reaction Turbines
Reaction turbines operate on a different principle compared to impulse turbines. While impulse turbines rely on high-velocity steam jets that impact the blades, reaction turbines utilize the reaction force generated by the expansion and acceleration of steam as it flows over the blades. In a reaction turbine, energy is extracted continuously as steam expands and passes through both fixed and moving blades, leading to a smooth and gradual conversion of thermal energy into mechanical energy.
- Continuous Expansion and Pressure Drop:
- Unlike impulse turbines, where steam undergoes a pressure drop only in the nozzles, reaction turbines have a continuous pressure drop across both the fixed and moving blades. The steam expands as it flows through the turbine, and this expansion creates a reaction force that drives the rotor.
- The key to the reaction turbine’s operation is that both the stationary (fixed) and rotating (moving) blades act as nozzles, allowing the steam to expand and accelerate. As the steam accelerates, it exerts a reactive force on the blades, pushing them and causing the rotor to spin.
- Role of Fixed and Moving Blades:
- In a reaction turbine, the steam first passes through a set of fixed blades (stator), which direct the steam onto the moving blades (rotor) at an appropriate angle. The fixed blades are designed to control the steam flow and ensure that it strikes the moving blades efficiently.
- As the steam flows over the moving blades, it undergoes further expansion and acceleration, generating a reaction force that drives the blades forward. This continuous process of expansion and acceleration allows the turbine to extract energy from the steam smoothly and efficiently.
- Reaction Force and Energy Conversion:
- The principle of operation in a reaction turbine is based on Newton’s Third Law of Motion, which states that for every action, there is an equal and opposite reaction. As steam flows through the blades and accelerates, it generates a reactive force that pushes against the blades, causing them to move.
- The continuous nature of this energy conversion process makes reaction turbines particularly suitable for situations where a steady and smooth power output is required. Because the steam expands across both the fixed and moving blades, energy extraction is more gradual and continuous compared to the discrete, high-impact energy transfer in impulse turbines.
Pressure and Velocity Dynamics in Reaction Blades
One of the defining characteristics of reaction turbines is the way they handle changes in steam pressure and velocity. The design of the blades ensures that these changes are managed efficiently, allowing the turbine to extract maximum energy from the steam.
- Pressure Drop Across Blades:
- In reaction turbines, the pressure drop occurs across both the stationary and rotating blades. As steam flows from one set of blades to the next, its pressure gradually decreases, and its velocity increases. This contrasts with impulse turbines, where the pressure drop occurs only in the nozzles, and the blades merely redirect high-velocity steam.
- The gradual pressure drop across multiple stages makes reaction turbines ideal for applications where the steam needs to be expanded over a longer period, allowing for continuous energy extraction. This design minimizes the abrupt changes in energy that can lead to inefficiencies and mechanical stress.
- Blade Design for Expansion and Acceleration:
- The blades of a reaction turbine are designed to allow steam to expand and accelerate as it flows over them. This is achieved by shaping the blades in a way that they act like nozzles, causing the steam to accelerate as it exits. This acceleration generates a reaction force that drives the blades and the rotor.
- The aerodynamic design of the blades is crucial for ensuring that the steam flow remains smooth and efficient, minimizing turbulence and energy losses. The blades are often curved or twisted to optimize the flow path of the steam, ensuring that it strikes the blades at the right angle and with maximum efficiency.
- Velocity and Energy Transfer:
- As the steam moves through the reaction turbine, its velocity increases while its pressure decreases. This continuous acceleration ensures that energy is transferred smoothly from the steam to the rotor, providing a steady and consistent power output.
- The energy transfer process in reaction turbines is highly efficient because it utilizes both the kinetic energy (from the steam’s velocity) and the pressure energy (from the continuous expansion) of the steam. This dual energy extraction mechanism is one of the reasons why reaction turbines can achieve high efficiencies in certain operating conditions.
Thermodynamics and the Rankine Cycle in Reaction Turbines
Reaction turbines are also integral to the Rankine cycle, the thermodynamic process that governs how steam is converted into mechanical work. However, the way they fit into the cycle is slightly different from impulse turbines due to their continuous pressure and energy changes.
- Isentropic Expansion in Reaction Stages:
- In the Rankine cycle, the steam expands isentropically (without heat loss) through the turbine, converting thermal energy into mechanical energy. In a reaction turbine, this expansion is continuous and occurs across multiple stages of fixed and moving blades.
- The design of the reaction turbine allows the steam to expand gradually, maintaining a more consistent flow of energy transfer. This gradual expansion helps to reduce moisture content in the steam, which is a critical factor in maintaining efficiency and preventing blade erosion.
- Pressure and Temperature Changes:
- The continuous expansion in reaction turbines means that the pressure and temperature of the steam decrease steadily as it passes through the turbine. This contrasts with the sharp pressure drop seen in impulse turbines. The steady reduction in pressure and temperature is beneficial for maintaining smooth and efficient energy conversion.
- The thermodynamic efficiency of a reaction turbine is often higher when operating under conditions where steam can be expanded over a wide range of pressures. This makes reaction turbines well-suited for large power plants, where the goal is to maximize energy extraction from steam.
- Integration with Regenerative and Reheat Cycles:
- Like impulse turbines, reaction turbines can be integrated with regenerative feedwater heating and reheat cycles to improve efficiency. In a regenerative cycle, steam is extracted from the turbine at various stages and used to preheat the feedwater, reducing the amount of energy needed to convert it into steam. This preheating process improves the overall efficiency of the system.
- Reheating is another technique used in conjunction with reaction turbines. After steam has partially expanded in the turbine, it is sent back to the boiler to be reheated and then expanded further. This reduces the formation of moisture in the later stages of the turbine, which can improve efficiency and extend the life of the turbine blades.
Stage Configuration: Single and Multi-stage Reaction Turbines
Reaction turbines can be configured as single-stage or multi-stage machines, depending on the requirements of the application. The choice between these configurations affects the efficiency, power output, and operational flexibility of the turbine.
- Single-stage Reaction Turbines:
- Single-stage reaction turbines are simpler and typically used in applications where the power demand is relatively low, and the steam conditions do not require extensive expansion. These turbines are easier to design and maintain, but they may not achieve the same level of efficiency as multi-stage configurations.
- They are often used in smaller industrial applications where the primary goal is to provide mechanical power for processes rather than generate large amounts of electricity.
- Multi-stage Reaction Turbines:
- Multi-stage reaction turbines are more complex and are designed to handle significant steam expansion over multiple stages. Each stage consists of a set of fixed and moving blades that gradually expand the steam, extracting energy at every point.
- The advantage of multi-stage designs is that they can handle larger volumes of steam and operate efficiently over a wide range of pressures. This makes them ideal for large-scale power plants and marine propulsion systems, where continuous, high-efficiency operation is essential.
- Multi-stage turbines can also be compound turbines, which combine elements of impulse and reaction designs to optimize performance. For example, the high-pressure stages might use impulse designs, while the low-pressure stages employ reaction principles to take advantage of continuous expansion.
- Efficiency Considerations:
- Multi-stage reaction turbines are generally more efficient than single-stage versions because they can extract more energy from the steam by expanding it gradually. However, this increased efficiency comes with a trade-off in terms of complexity and cost.
- The choice between single-stage and multi-stage configurations depends on the specific requirements of the application, including factors like steam pressure, flow rate, and desired power output. Engineers must carefully evaluate these parameters to select the most appropriate turbine design.
Conclusion on Reaction Turbine Principles
Reaction turbines offer a continuous and smooth method of converting steam energy into mechanical work. Unlike impulse turbines, which rely on discrete high-velocity steam jets, reaction turbines allow steam to expand and accelerate continuously across multiple stages. This approach makes them particularly efficient for applications where a steady and consistent power output is required, such as in large power plants and marine propulsion systems.
The design of reaction turbines, with their gradual pressure drop and continuous energy extraction, allows for high efficiency in scenarios where steam can be expanded over a wide range of pressures. The integration of regenerative and reheat cycles further enhances their performance, making them suitable for large-scale energy production.
Understanding the working principles of reaction turbines helps engineers optimize their operation and choose the right turbine for specific industrial and power generation needs. Their ability to handle continuous steam flow and gradual expansion makes them a vital component in modern energy systems, and ongoing technological advancements promise even greater efficiency and reliability in the future.
Steam Turbines
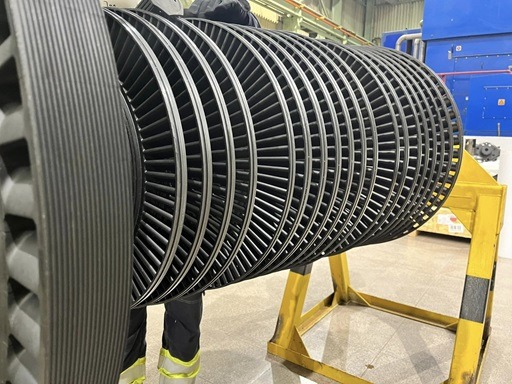
Steam turbines are remarkable machines that convert thermal energy from steam into mechanical work, widely used in power generation, industrial processes, and propulsion systems. They operate on the principle of expanding high-pressure steam through a series of blades, causing rotation that can drive generators or other machinery. Over time, engineers have developed various types of steam turbines, each designed to optimize efficiency, performance, and application-specific requirements. Below is an exploration of the primary types of steam turbines, their configurations, and their uses.
1. Impulse Turbines
Impulse turbines operate based on the impulse principle, where high-pressure steam is directed through nozzles to form high-velocity jets that strike the turbine blades. The kinetic energy of the steam is transferred to the blades, causing the rotor to spin. In this design, the pressure drop occurs entirely in the nozzles, and the blades experience no significant pressure change as the steam passes through. A classic example of an impulse turbine is the De Laval turbine, which features a single stage and is known for its simplicity and high rotational speeds. Another well-known design is the Curtis turbine, which uses multiple stages of moving and stationary blades to extract energy more efficiently in a compact form.
Impulse turbines are often used in small-scale power generation or as the high-pressure stages in larger systems. Their advantages include simplicity and the ability to handle high-pressure steam effectively, though they may be less efficient at lower speeds or with variable loads.
2. Reaction Turbines
In contrast to impulse turbines, reaction turbines rely on both pressure drop and steam expansion across the turbine blades themselves. As steam passes through the moving blades, it accelerates and expands, creating a reactive force (similar to how a rocket works) that drives the rotor. This design was pioneered by Sir Charles Parsons, and the Parsons turbine remains a foundational example. Reaction turbines typically feature multiple stages, with alternating rows of fixed (stator) and moving (rotor) blades, allowing for gradual energy extraction and higher efficiency.
Reaction turbines are widely used in large power plants because they excel at handling lower-pressure steam and can achieve greater efficiency over a range of operating conditions. However, they are more complex and costly to manufacture due to the precision required in blade design and staging.
3. Combination (Impulse-Reaction) Turbines
Many modern steam turbines combine impulse and reaction principles to optimize performance across different pressure ranges. For example, the high-pressure stages might use an impulse design to handle the initial steam conditions, while the low-pressure stages transition to a reaction design for better efficiency as the steam expands. This hybrid approach allows turbines to adapt to a wide variety of operating conditions, making them common in large-scale electricity generation plants.
4. Back-Pressure Turbines
Back-pressure turbines exhaust steam at a pressure higher than atmospheric pressure, allowing the exhaust steam to be used for industrial processes like heating, drying, or driving other machinery. These turbines are often found in cogeneration systems, where both electricity and heat are needed, such as in paper mills, chemical plants, or district heating systems. While they sacrifice some efficiency in power generation compared to condensing turbines, their ability to provide dual outputs makes them highly economical in specific applications.
5. Condensing Turbines
Condensing turbines are designed to maximize power output by exhausting steam into a vacuum, typically created by a condenser. This lowers the back pressure, allowing the steam to expand further and extract more energy. These turbines are the backbone of most large-scale power plants, including coal, nuclear, and combined-cycle gas plants. Their high efficiency comes at the cost of requiring a cooling system (often water-based), which adds complexity and environmental considerations.
6. Extraction Turbines
Extraction turbines are a versatile subtype that allow steam to be “extracted” at intermediate pressures from various stages of the turbine. This extracted steam can be used for industrial processes or heating, while the remaining steam continues through the turbine to generate power. These turbines are common in facilities needing both electricity and steam at different pressure levels, offering flexibility and efficiency in combined heat and power (CHP) systems.
7. Reheat Turbines
Reheat turbines improve efficiency by incorporating a reheat cycle. After passing through the high-pressure stages, steam is sent back to the boiler to be reheated before entering the intermediate- or low-pressure stages. This process increases the average temperature at which heat is added, boosting the turbine’s thermodynamic efficiency. Reheat designs are standard in large, high-efficiency power plants, though they require additional equipment and control systems.
8. Low-Pressure, Intermediate-Pressure, and High-Pressure Turbines
In large power plants, steam turbines are often divided into separate sections based on steam pressure: high-pressure (HP), intermediate-pressure (IP), and low-pressure (LP) turbines. These sections are typically mounted on a single shaft and work together to extract energy as the steam expands from high to low pressure. Each section is optimized for its specific pressure range, with blade sizes and designs varying accordingly—HP turbines have smaller, robust blades, while LP turbines have larger blades to handle the expanded, lower-pressure steam.
9. Single-Stage vs. Multi-Stage Turbines
Steam turbines can also be classified by the number of stages. Single-stage turbines, like the De Laval design, are simple and compact, suitable for small-scale or high-speed applications. Multi-stage turbines, such as those used in power plants, consist of multiple sets of blades, allowing for gradual energy extraction and higher efficiency. Multi-stage designs dominate in large-scale applications due to their ability to handle large steam volumes and pressure drops.
Applications and Considerations
Each type of steam turbine serves a specific purpose. Impulse turbines might power small generators or pumps, while reaction turbines drive massive gigawatt-scale power stations. The choice of turbine type depends on factors like steam conditions (pressure, temperature, and flow rate), desired output (power, heat, or both), and operational constraints (space, cost, and maintenance). Let’s dive deeper into each type with additional details on their design, mechanics, and real-world applications.
1. Impulse Turbines
Impulse turbines rely on the conversion of steam’s potential energy into kinetic energy before it interacts with the blades. The steam is accelerated through stationary nozzles, which are precisely shaped (often converging-diverging nozzles) to achieve supersonic velocities. When this high-speed jet hits the turbine’s bucket-shaped blades, the momentum transfer causes rotation. The blades are symmetrically designed to minimize axial thrust, and the steam exits at roughly the same pressure it entered, having lost much of its kinetic energy.
- De Laval Turbine: Invented by Gustaf de Laval in the late 19th century, this single-stage turbine was revolutionary for its time. It’s compact, with a single row of blades, and can reach speeds exceeding 30,000 RPM, making it ideal for driving high-speed machinery like centrifugal pumps or small generators. However, its efficiency drops with varying loads, limiting its use to niche applications.
- Curtis Turbine: Developed by Charles G. Curtis, this design adds a velocity-compounding feature. Steam passes through multiple rows of moving blades interspersed with stationary blades that redirect the flow. This staged approach reduces the rotor speed (compared to De Laval) while extracting more energy, making it suitable for early electrical generation systems.
- Applications: Impulse turbines shine in high-pressure, low-flow scenarios, such as topping turbines in combined-cycle plants or standalone units in remote locations. They’re less common in modern large-scale power generation due to efficiency limitations but remain critical in specialized industrial setups.
2. Reaction Turbines
Reaction turbines operate on a different principle: the blades act as nozzles themselves, accelerating and expanding the steam as it flows through. This creates a drop in pressure across each stage, generating a reactive force that drives the rotor. The stator blades (fixed) direct steam onto the rotor blades (moving), and the process repeats across multiple stages. The degree of reaction—typically around 50% in a Parsons turbine—refers to the proportion of energy extracted via reaction versus impulse.
- Parsons Turbine: Sir Charles Parsons’ 1884 invention introduced the multi-stage reaction concept, a breakthrough that transformed power generation. His turbines feature dozens or even hundreds of stages, with blade heights increasing as steam expands. This gradual energy extraction maximizes efficiency, especially at lower pressures.
- Design Nuances: Reaction turbine blades are airfoil-shaped, requiring precise manufacturing to handle aerodynamic forces and steam expansion. The rotor and stator blades are often paired in a 1:1 ratio, creating a balanced, continuous flow. Axial thrust is a challenge, necessitating thrust bearings to stabilize the rotor.
- Applications: Reaction turbines dominate in large fossil-fuel, nuclear, and geothermal power plants due to their scalability and efficiency at handling high steam volumes. They’re less suited to small-scale or high-pressure-only applications, where impulse designs may outperform.
3. Combination (Impulse-Reaction) Turbines
Combination turbines blend the strengths of both designs. The high-pressure section often uses impulse stages to manage the intense initial conditions (e.g., 200 bar, 540°C), where nozzles and robust blades excel. As steam pressure drops, the turbine transitions to reaction stages, leveraging expansion for efficiency in the intermediate- and low-pressure zones. This hybrid layout is tailored to the steam cycle’s thermodynamic profile.
- Mechanics: The transition between impulse and reaction stages is seamless, with blade designs and staging adjusted to match pressure gradients. For example, early stages might feature pure impulse (100% pressure drop in nozzles), while later stages approach 50% reaction.
- Advantages: This design optimizes efficiency across a wide pressure range, reduces mechanical stress, and allows for compact yet powerful turbines. It’s a staple in modern supercritical and ultra-supercritical coal plants, where steam conditions push material limits.
- Applications: Found in utility-scale power generation, especially where efficiency and output must be maximized, such as in combined-cycle plants integrating gas and steam turbines.
4. Back-Pressure Turbines
Back-pressure turbines exhaust steam at a usable pressure (e.g., 5-20 bar) rather than condensing it into a vacuum. The exhaust steam retains significant thermal energy, making it ideal for downstream processes. These turbines often operate in a non-condensing mode, with exhaust piped directly to industrial systems.
- Design Details: Simpler than condensing turbines, they lack a condenser and cooling system, reducing capital costs. Blade staging is optimized for a specific exhaust pressure, balancing power output with steam quality for process use.
- Efficiency Trade-Off: Electrical efficiency is lower than condensing turbines (since less energy is extracted), but total energy efficiency soars when process heat is factored in—sometimes exceeding 80% in cogeneration setups.
- Applications: Common in industries like pulp and paper (for drying), sugar refining (for evaporation), and petrochemical plants (for heating). They’re also used in district heating systems, where exhaust steam warms buildings.
5. Condensing Turbines
Condensing turbines push efficiency to the limit by exhausting steam into a vacuum (e.g., 0.05 bar), created by a condenser cooled with water or air. This maximizes the pressure drop across the turbine, extracting nearly all available energy from the steam.
- Mechanics: The low-pressure stages feature massive blades—sometimes over a meter long—to handle the high-volume, low-density steam. Condensers require significant infrastructure, including cooling towers or river/ocean water systems, adding complexity.
- Materials and Challenges: LP blades face erosion from wet steam (containing water droplets), necessitating alloys like titanium or protective coatings. Vacuum maintenance is critical, as leaks reduce efficiency.
- Applications: The backbone of baseload power plants—coal, nuclear, and gas-fired—where maximum electrical output is the goal. They’re less practical in small-scale or heat-focused systems due to their reliance on cooling.
6. Extraction Turbines
Extraction turbines offer flexibility by allowing steam to be tapped at intermediate points. Valves control the extraction process, diverting steam at specific pressures (e.g., 10 bar for heating, 2 bar for feedwater preheating) while the rest continues to the condenser.
- Design Complexity: Multiple extraction points require sophisticated control systems and additional piping. Blade staging must account for variable flow rates, as extraction reduces steam volume in later stages.
- Benefits: They balance power and heat output, adapting to fluctuating demands. Efficiency remains high when extraction is optimized with process needs.
- Applications: Prevalent in refineries, steel mills, and CHP plants, where steam serves dual purposes—electricity for operations and heat for processes like distillation or drying.
7. Reheat Turbines
Reheat turbines enhance efficiency by interrupting the expansion process. After the HP stages, steam (now at reduced pressure and temperature) returns to the boiler for reheating (e.g., back to 540°C), then re-enters the IP and LP stages. This raises the cycle’s average heat-addition temperature, a key thermodynamic advantage.
- Mechanics: Reheat requires additional piping, valves, and boiler capacity. Double-reheat systems (two reheats) push efficiency further but increase costs. LP stages must handle wetter steam post-reheat, requiring moisture separators.
- Efficiency Gains: Single reheat boosts efficiency by 4-5%, while double reheat adds another 2-3%, making them viable in ultra-efficient plants (e.g., 45%+ thermal efficiency).
- Applications: Standard in modern fossil-fuel plants, especially supercritical designs, and some nuclear plants with high steam output.
8. Low-Pressure, Intermediate-Pressure, and High-Pressure Turbines
In large systems, turbines are segmented into HP, IP, and LP units, often on a single shaft. Each section is a mini-turbine tailored to its steam conditions:
- HP Turbine: Small, robust blades handle ultra-high pressures (up to 300 bar) and temperatures (600°C+). Materials like chromium-steel alloys resist creep and corrosion.
- IP Turbine: Mid-sized blades manage reheated steam (20-50 bar), balancing strength and flow capacity.
- LP Turbine: Large blades (up to 1.5 meters) process low-pressure, high-volume steam, often in twin-flow designs to split the exhaust load.
- Applications: Universal in utility-scale plants, where modularity simplifies maintenance and optimization.
9. Single-Stage vs. Multi-Stage Turbines
- Single-Stage: Compact, with one set of blades, they’re fast and simple but inefficient for large power outputs. Used in small pumps, fans, or emergency generators.
- Multi-Stage: Multiple blade rows extract energy gradually, ideal for high-power applications. Complexity increases, but so does efficiency—up to 90% of available energy in modern designs.
Closing Thoughts
Steam turbines are marvels of engineering, with each type fine-tuned to its role. From the brute simplicity of a De Laval impulse turbine to the intricate staging of a reheat reaction turbine, their diversity reflects the ingenuity behind harnessing steam’s power. Whether driving a factory or lighting a city, these machines remain central to our energy landscape, evolving with advances in materials, controls, and thermodynamics.
Let’s continue expanding on the fascinating world of steam turbines, diving even deeper into the nuances of each type—covering their operational mechanics, historical context, material considerations, and modern innovations. The goal here is to provide a comprehensive picture of how these machines function and why they’re engineered the way they are.
1. Impulse Turbines
The impulse turbine’s elegance lies in its straightforward energy transfer: steam’s kinetic energy is the sole driver. The nozzles are critical—they’re often made of high-strength alloys like stainless steel or Inconel to withstand erosion from high-velocity steam, especially if it carries moisture or particulates. Blade design is equally vital; the “buckets” are typically curved and polished to minimize friction losses, with precise angles to maximize momentum transfer.
- Historical Context: Gustaf de Laval’s 1880s design was a leap forward during the Second Industrial Revolution, enabling high-speed machinery when electricity was still emerging. His turbines powered early cream separators (a key invention of his), showcasing their versatility beyond power generation.
- Velocity Compounding (Curtis): In a Curtis turbine, steam ricochets between moving and stationary blades multiple times within a stage. This reduces the rotor speed to manageable levels (e.g., 3,000-6,000 RPM) for coupling with generators, avoiding the need for gearboxes—a common requirement with De Laval’s ultra-fast designs.
- Modern Use: Today, impulse turbines are often “topping” units in hybrid plants, taking the first bite of high-pressure steam before handing it off to reaction stages. Advances in computational fluid dynamics (CFD) have refined nozzle and blade profiles, squeezing out extra efficiency.
- Challenges: Efficiency drops sharply if steam flow deviates from design conditions, and they’re prone to losses from turbulence if not perfectly aligned. Materials must also resist thermal shock from rapid startups.
2. Reaction Turbines
Reaction turbines are the workhorses of large-scale power, thriving on their ability to extract energy incrementally. Each stage contributes a small pressure drop, with steam velocity increasing as it passes through the rotor blades. This continuous expansion demands tight tolerances—gaps between rotor and stator must be minimized to prevent steam leakage, often requiring labyrinth seals or brush seals.
- Parsons’ Legacy: Parsons’ first turbine, built in 1884, powered a ship’s lighting system, proving the concept. By 1900, his multi-stage designs were generating megawatts, outpacing rivals. His insight was staging: rather than one big energy drop, he spread it across dozens of steps.
- Blade Dynamics: Blades are aerodynamically complex, resembling aircraft wings. Steam accelerates through converging passages in the rotor, creating lift-like forces. Blade lengths increase down the turbine (e.g., 20 mm in HP stages to 1 m in LP stages) to match the expanding steam volume.
- Material Science: High-temperature stages use nickel-based superalloys (e.g., Inconel 718) for creep resistance, while LP blades might use titanium to combat wet-steam erosion. Coatings like chromium carbide protect against wear.
- Applications Today: Reaction turbines power nuclear plants (handling saturated steam at 70 bar) and geothermal systems (low-pressure, corrosive steam), showcasing their adaptability. Efficiency can hit 90% of the ideal Rankine cycle in optimized designs.
3. Combination (Impulse-Reaction) Turbines
The combination turbine’s hybrid nature makes it a masterpiece of compromise. The impulse stages handle the brutal initial conditions—supercritical steam at 300 bar and 600°C—where robust, simple blades thrive. As pressure falls, reaction stages take over, leveraging expansion for finesse and efficiency. This staged approach mirrors the steam’s thermodynamic journey.
- Staging Details: A typical layout might feature 2-4 impulse stages followed by 10-20 reaction stages. The transition isn’t abrupt—designers blend characteristics, gradually increasing the reaction component (e.g., 10% to 50% across stages).
- Thermodynamic Edge: By tailoring each section to its pressure zone, losses from over-expansion or under-utilization are minimized. CFD and 3D modeling now optimize this blend, reducing shock losses where steam shifts behavior.
- Real-World Example: In a 1,000 MW coal plant, the HP turbine might be impulse-driven, the IP turbine a mix, and the LP turbine fully reaction-based, all on one shaft spinning at 3,600 RPM (for 60 Hz grids).
- Innovations: Adaptive staging, where blade angles adjust via actuators, is emerging, allowing turbines to handle variable steam inputs from renewable-integrated grids.
4. Back-Pressure Turbines
Back-pressure turbines prioritize versatility over raw power. The exhaust pressure is a design parameter—say, 10 bar for a chemical reactor or 3 bar for a dryer—set by downstream needs. This steam isn’t wasted in a condenser but repurposed, making these turbines stars of energy efficiency in the right context.
- Operational Mechanics: The turbine’s last stage is tuned to maintain the target exhaust pressure, often with fewer blades than a condensing turbine’s sprawling LP section. Steam quality (dryness) is critical—wet steam can damage process equipment downstream.
- Historical Use: Early 20th-century factories used back-pressure turbines to power machinery while heating workspaces, a precursor to modern cogeneration.
- Modern Systems: In a pulp mill, a 20 MW back-pressure turbine might generate electricity while supplying 50 tons/hour of 5-bar steam for digesters. Combined efficiency (power + heat) can exceed 85%.
- Limitations: Power output is capped by the exhaust pressure—lower pressure means more electricity, but less usable heat. Balancing these trade-offs requires precise engineering.
5. Condensing Turbines
Condensing turbines chase the thermodynamic ideal: maximum work from steam. The condenser creates a vacuum (e.g., 0.03-0.1 bar), dropping the exhaust temperature to 30-50°C, far below atmospheric boiling. This extends the Rankine cycle’s pressure range, extracting energy that other turbines leave behind.
- LP Stage Scale: LP blades are engineering marvels—some span over 1.5 meters, with tips moving at supersonic speeds (e.g., 500 m/s). Dual-flow designs split steam to reduce axial thrust and fit within casing limits.
- Cooling Systems: Water-cooled condensers dominate, using rivers or cooling towers (evaporating 1-2% of a plant’s output as waste heat). Air-cooled variants are growing in arid regions, though they’re less efficient.
- Challenges: Wet steam in LP stages erodes blades, requiring moisture separators or superheating. Vacuum leaks sap efficiency, demanding robust seals and monitoring.
- Applications: A 1,200 MW nuclear turbine might have three LP sections, each exhausting to its own condenser, producing enough power for a small city.
6. Extraction Turbines
Extraction turbines are the multitaskers of the turbine world, juggling power and process steam. Extraction points—typically 1-3 per turbine—are fitted with control valves, allowing operators to adjust flow on the fly. This adaptability suits industries with variable heat demands.
- Control Systems: Advanced turbines use digital governors to balance extraction and power output, responding to real-time needs. Steam bypassing an extraction point continues to the next stage, maintaining rotor stability.
- Design Trade-Offs: Each extraction reduces mass flow downstream, shrinking LP blade sizes compared to a pure condensing turbine. Efficiency hinges on matching extraction pressures to process requirements.
- Example: In a refinery, a 50 MW extraction turbine might supply 15 bar steam for reboilers (20 tons/hour) and 2 bar steam for tracing (10 tons/hour), with the rest condensed for power.
- Modern Twist: Some units pair with solar-thermal systems, extracting steam for industrial use while generating renewable power.
7. Reheat Turbines
Reheat turbines push the Rankine cycle’s limits by adding heat mid-process. After the HP turbine drops steam to 20-50 bar, reheating to 540-600°C boosts its enthalpy, allowing more work in the IP and LP stages. Double-reheat designs repeat this, though cost-benefit diminishes beyond two cycles.
- Mechanics: Reheat piping adds 10-20% to a plant’s footprint, with superheaters handling corrosive, high-temperature steam. Moisture separators post-reheat dry the steam, protecting LP blades.
- Efficiency Math: A single reheat lifts thermal efficiency from ~38% to 42-43%; double reheat might hit 45-47% in ultra-supercritical plants (600°C, 300 bar).
- Applications: Dominant in high-efficiency fossil plants, like Germany’s lignite stations or Japan’s advanced coal units. Nuclear plants occasionally use reheat with moisture separation for saturated steam cycles.
8. Low-Pressure, Intermediate-Pressure, and High-Pressure Turbines
These segmented turbines are a symphony of specialization. The HP turbine faces the harshest conditions, often operating near material limits (e.g., 620°C with advanced ferritic steels). IP turbines bridge the gap, while LP turbines handle the voluminous exhaust.
- Shaft Dynamics: A single shaft aligns all three, spinning at 3,000 RPM (50 Hz) or 3,600 RPM (60 Hz). Bearings and couplings absorb massive torque and thermal expansion.
- Applications: A 900 MW combined-cycle plant might pair an HP-IP turbine with twin LP units, optimizing gas-steam integration.
9. Single-Stage vs. Multi-Stage Turbines
- Single-Stage: Limited to 1-5 MW, they’re rugged and low-maintenance—think backup generators or marine pumps.
- Multi-Stage: Scaling to gigawatts, they’re the pinnacle of efficiency, with 50+ stages in some LP sections.
Final Notes
Steam turbines evolve with technology—ceramic coatings, 3D-printed blades, and AI-driven controls are pushing boundaries. Their adaptability keeps them relevant, from fossil giants to renewable hybrids, proving their enduring role in energy.
What Are Steam Turbines?
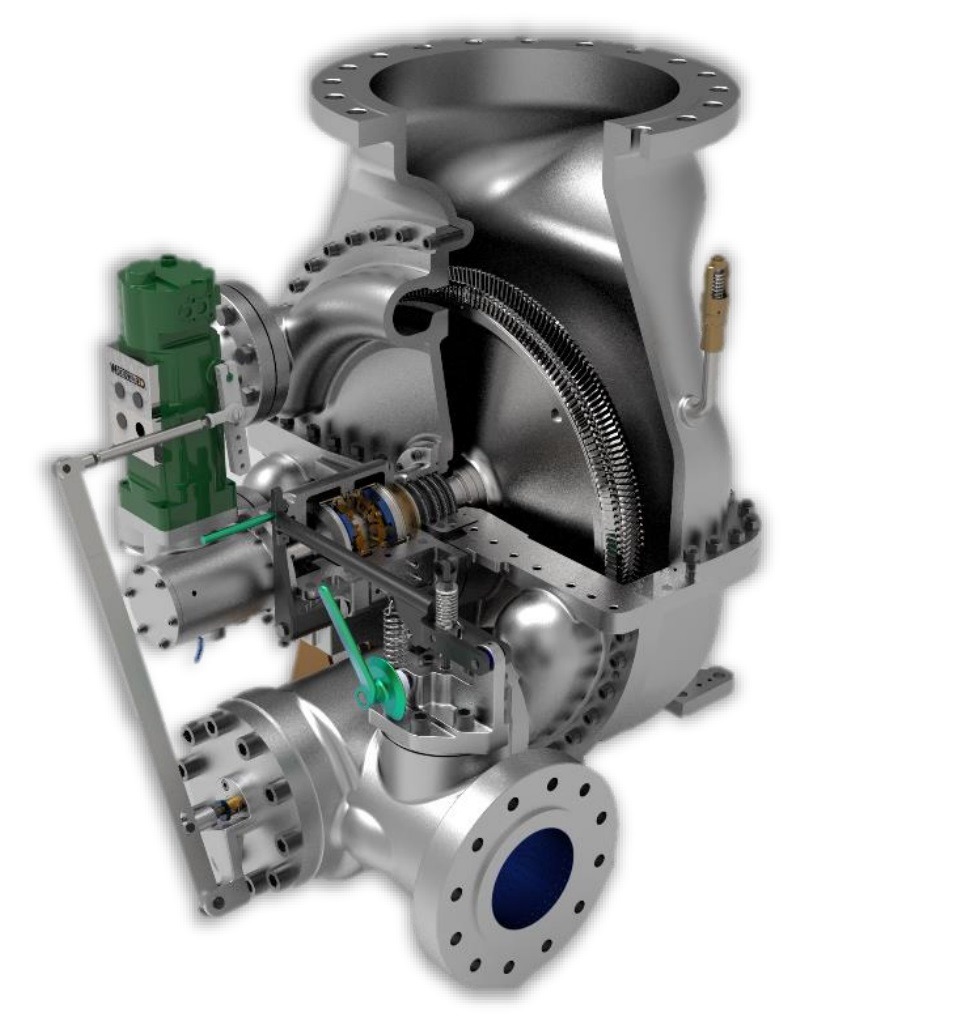
Steam turbines are mechanical devices that convert thermal energy from pressurized steam into rotational mechanical energy, which can then drive generators to produce electricity or power machinery like pumps and compressors. Invented in the late 19th century, they remain a cornerstone of power generation and industrial processes worldwide, prized for their efficiency, scalability, and adaptability to various heat sources—coal, nuclear, biomass, natural gas, or even solar thermal energy.
Basic Operating Principle
The steam turbine operates on the Rankine cycle, a thermodynamic process where heat transforms water into steam, which then expands to do work. Here’s how it unfolds:
- Heat Input: A heat source (e.g., burning fuel or nuclear fission) boils water in a boiler, creating high-pressure, high-temperature steam (typically 200-600°C, 10-300 bar).
- Expansion: This steam flows into the turbine, passing through blades mounted on a rotor. As it expands and loses pressure, it accelerates, pushing the blades and spinning the rotor.
- Work Output: The rotor’s rotation drives a connected shaft, powering a generator (for electricity) or other equipment.
- Exhaust: Spent steam exits—either to a condenser (cooling it back to water for reuse) or as usable heat (in CHP systems).
Efficiency depends on the steam’s initial conditions and the turbine’s design, typically ranging from 20% in small, simple units to over 45% in advanced power plants.
Types of Steam Turbines
Steam turbines vary widely based on design, pressure handling, and application. Here are the main categories:
- Impulse Turbines
- How They Work: Steam accelerates through stationary nozzles into high-velocity jets, striking curved blades (buckets) on the rotor. The pressure drop occurs entirely in the nozzles, not across the blades.
- Examples: The De Laval turbine (single-stage, high-speed) and Curtis turbine (velocity-compounded with multiple blade rows).
- Features: Simple, robust, and suited for high-pressure steam. Speeds can exceed 30,000 RPM in small units.
- Applications: Small-scale power generation, topping turbines in hybrid plants, or driving high-speed machinery.
- Reaction Turbines
- How They Work: Steam expands and accelerates through both fixed (stator) and moving (rotor) blades, dropping pressure gradually across each stage. The blades act like nozzles, creating a reactive force.
- Examples: The Parsons turbine, with dozens of stages for efficiency.
- Features: Complex, with airfoil-shaped blades and tight tolerances. Excels at lower pressures and high steam volumes.
- Applications: Large power plants (coal, nuclear, geothermal) needing maximum efficiency.
- Combination (Impulse-Reaction) Turbines
- How They Work: High-pressure stages use impulse designs, transitioning to reaction stages as pressure drops, optimizing energy extraction across the steam’s range.
- Features: Balances simplicity and efficiency, common in modern utility-scale turbines.
- Applications: Supercritical coal or combined-cycle gas plants.
- Condensing Turbines
- How They Work: Steam exhausts into a vacuum (0.03-0.1 bar) created by a condenser, maximizing pressure drop and energy output.
- Features: Large low-pressure (LP) blades handle high-volume steam. Requires cooling systems (water or air).
- Applications: Baseload power plants (e.g., 1,000 MW nuclear units).
- Back-Pressure Turbines
- How They Work: Steam exits at a higher pressure (e.g., 5-20 bar) for industrial use, rather than condensing.
- Features: Simpler, with dual power-and-heat output (CHP). Less efficient electrically but highly effective overall.
- Applications: Factories (e.g., paper mills) needing process steam.
- Extraction Turbines
- How They Work: Steam is tapped at intermediate stages for heating or processes, with the rest continuing to generate power.
- Features: Flexible, with control valves adjusting extraction flow.
- Applications: Refineries or district heating systems.
- Reheat Turbines
- How They Work: Steam exits the high-pressure (HP) stage, gets reheated in the boiler (e.g., to 540°C), then expands through intermediate-pressure (IP) and LP stages.
- Features: Boosts efficiency by 4-5% per reheat cycle. Complex, with extra piping.
- Applications: High-efficiency fossil-fuel plants.
- HP, IP, and LP Turbines
- How They Work: Segmented into sections on a single shaft, each optimized for its pressure range—small HP blades for high pressure, large LP blades for low pressure.
- Features: Modular, scalable, and standard in large plants.
- Applications: Gigawatt-scale power stations.
Historical Development
- Origins: Sir Charles Parsons invented the modern steam turbine in 1884, demonstrating a multi-stage reaction design that powered a ship’s lights. Gustaf de Laval’s 1880s impulse turbine complemented this, excelling at high speeds.
- Industrial Revolution: By 1900, turbines replaced steam engines in power generation, offering higher efficiency and scalability. Parsons’ designs scaled to megawatts within decades.
- 20th Century: Turbines evolved with metallurgy (e.g., chromium steels) and thermodynamics (e.g., reheat cycles), driving electrification worldwide.
Applications
- Power Generation: Over 80% of global electricity comes from steam turbines—coal (37%), nuclear (10%), gas (25%), and biomass/geothermal (small shares).
- Industrial: Drive compressors in chemical plants, pumps in refineries, or process steam in paper production.
- Marine Propulsion: Powered ships like the Titanic (29,000 hp) and warships until gas turbines took over post-WWII.
- Renewables: Biomass plants (e.g., wood-fired) and concentrated solar power use steam turbines for green energy.
Design and Materials
- Blades: HP blades (small, robust) use nickel-based superalloys (e.g., Inconel) for 600°C+ conditions. LP blades (large, lightweight) often use titanium to resist wet-steam erosion.
- Casings: Steel or alloy casings withstand pressure and thermal stress, with seals (labyrinth or brush) minimizing leaks.
- Rotor: Forged steel or alloy, balanced to handle 3,000-10,000 RPM and thermal expansion.
- Challenges: Creep (deformation at high heat), erosion (from wet steam), and fatigue (from cyclic loads) drive material innovation.
Efficiency and Performance
- Thermodynamic Limits: Ideal Rankine efficiency is ~60%, but real-world turbines hit 30-47%, depending on steam conditions (supercritical at 600°C, 300 bar pushes the upper end).
- Losses: Heat escapes via exhaust, friction, and leakage. Moisture in LP stages reduces output unless mitigated by superheating or separators.
- Enhancements: Reheat cycles, supercritical steam, and advanced blade profiles (via CFD) boost performance.
Modern Relevance
- Fossil Fuels: Coal and gas plants rely on steam turbines, though coal’s share declines with decarbonization.
- Nuclear: Essential for converting reactor heat to power, with massive LP turbines handling saturated steam.
- Renewables: Biomass (e.g., wood) and geothermal plants keep turbines relevant in green energy.
- Innovation: Ceramic blades (1,000°C tolerance), 3D-printed parts, and AI-driven controls push efficiency and durability.
Advantages and Limitations
- Advantages: High power output (up to 1,500 MW per unit), long lifespan (30-50 years), and fuel flexibility.
- Limitations: Complex infrastructure (boilers, condensers), slow startup (hours vs. minutes for gas turbines), and reliance on water for cooling.
Steam turbines are engineering marvels, blending physics, materials science, and ingenuity. From Parsons’ first 7.5 kW unit to today’s gigawatt giants, they’ve shaped our energy landscape and continue to adapt to a changing world. Whether driving a factory or lighting a city, their legacy endures.
Steam turbines are mechanical devices that transform the thermal energy stored in pressurized steam into rotational mechanical energy, which can then be used to generate electricity or power industrial equipment like pumps, compressors, or fans. They are a critical technology in modern energy production, found in power plants, factories, and even historical ships, and they operate by harnessing the expansion of steam to spin a rotor connected to a generator or machinery. Invented over a century ago, steam turbines remain vital due to their efficiency, reliability, and ability to work with diverse heat sources such as coal, natural gas, nuclear reactions, biomass like wood, or concentrated solar power. Below is a detailed exploration of how they work, their types, history, applications, design specifics, and their role today.
The basic principle behind a steam turbine is straightforward yet rooted in thermodynamics, specifically the Rankine cycle, which is a process that converts heat into work. Water is heated in a boiler using a fuel source, turning it into high-pressure steam at temperatures ranging from 200 to 600 degrees Celsius and pressures from 10 to 300 bar, depending on the system. This steam is then directed into the turbine, where it flows over a series of blades attached to a rotor. As the steam expands and loses pressure, it accelerates, pushing against the blades and causing the rotor to spin at speeds typically between 1,500 and 3,600 revolutions per minute, aligning with electrical grid frequencies of 50 or 60 Hz. The spinning rotor drives a shaft connected to a generator, producing electricity, or directly powers mechanical equipment. After passing through the turbine, the steam exits either into a condenser, where it cools back into water for reuse in a closed loop, or as usable heat for industrial processes in systems designed for combined heat and power.
Steam turbines come in several types, each designed for specific purposes based on how they handle steam and extract energy. Impulse turbines work by accelerating steam through stationary nozzles into high-speed jets that strike curved, bucket-shaped blades on the rotor. The pressure drop happens entirely in the nozzles, so the blades experience only kinetic energy transfer, not a pressure change. A famous example is the De Laval turbine, a single-stage design invented by Gustaf de Laval in the 1880s, capable of spinning at over 30,000 RPM, ideal for small, high-speed applications like pumps or early generators. Another impulse variant, the Curtis turbine, uses multiple rows of moving and stationary blades to compound velocity, reducing speed to a more manageable 3,000-6,000 RPM while extracting more energy, making it suitable for early power plants. Impulse turbines are simple, robust, and excel with high-pressure steam, but they lose efficiency at lower pressures or variable loads.
Reaction turbines, in contrast, extract energy through both pressure drop and steam expansion across the blades themselves. Steam passes through fixed stator blades that direct it onto moving rotor blades, where it accelerates and expands, creating a reactive force that drives the rotor. This design, pioneered by Sir Charles Parsons in 1884, typically features dozens of stages—alternating fixed and moving blades—to gradually extract energy, making it highly efficient for large-scale power generation. The blades are shaped like airfoils, requiring precise manufacturing to handle aerodynamic forces, and their size increases from high-pressure to low-pressure stages to accommodate expanding steam. Reaction turbines dominate in big power plants because they handle lower-pressure steam well and achieve efficiencies up to 90% of the theoretical maximum, though they’re more complex and costly than impulse designs.
Many modern turbines combine impulse and reaction principles to optimize performance across a wide pressure range. In these combination turbines, the high-pressure stages use an impulse design with nozzles and sturdy blades to manage initial conditions—say, 300 bar and 600°C—while later stages shift to reaction designs as the steam expands, improving efficiency in intermediate- and low-pressure zones. This hybrid approach is common in supercritical coal plants or combined-cycle gas plants, where steam conditions push material limits, and designers use computational fluid dynamics to fine-tune blade transitions, minimizing energy losses.
Condensing turbines are built to maximize power output by exhausting steam into a vacuum, typically 0.03 to 0.1 bar, created by a condenser cooled with water from rivers, oceans, or cooling towers, or sometimes air in dry regions. This low exhaust pressure allows the steam to expand further, extracting more energy. These turbines feature massive low-pressure blades—sometimes over 1.5 meters long—to handle the high-volume, low-density steam, often in dual-flow designs to split the exhaust and reduce axial thrust. They’re the backbone of large power plants, like a 1,200 MW nuclear unit, but require extensive cooling infrastructure, adding complexity and environmental considerations like water usage or thermal pollution.
Back-pressure turbines, on the other hand, exhaust steam at a higher pressure, such as 5 to 20 bar, so it can be used for industrial processes like heating, drying, or driving other equipment. Found in factories like paper mills or chemical plants, they sacrifice some electrical efficiency—since less energy is extracted as work—but excel in combined heat and power setups, where total energy efficiency can reach 80% or more when heat is factored in. They’re simpler than condensing turbines, lacking a condenser, and their exhaust pressure is tailored to downstream needs, making them economical for specific industries.
Extraction turbines offer flexibility by allowing steam to be tapped at intermediate stages for industrial use, while the rest continues through the turbine to generate power. For example, a refinery might extract steam at 15 bar for heating and 2 bar for feedwater preheating, with the remainder condensing for electricity. Control valves adjust the extraction flow, and the turbine’s design accounts for reduced steam volume in later stages, balancing power and process demands. These are common in facilities needing both electricity and variable steam supplies, like steel mills or district heating systems.
Reheat turbines improve efficiency by interrupting the expansion process. After passing through the high-pressure stage, steam—now at 20-50 bar—returns to the boiler to be reheated to its original temperature, say 540°C, before entering intermediate- and low-pressure stages. This increases the cycle’s average heat-addition temperature, boosting efficiency by 4-5% for a single reheat, or up to 7% with double reheat in ultra-efficient plants. Reheat designs are standard in large fossil-fuel plants, requiring extra piping and moisture separators to handle wetter steam in later stages, but the added complexity pays off in fuel savings.
In large power plants, turbines are often split into high-pressure, intermediate-pressure, and low-pressure sections, each optimized for its steam conditions and mounted on a single shaft. High-pressure turbines have small, robust blades to handle extreme conditions—up to 300 bar and 600°C—using materials like chromium-steel alloys to resist creep and corrosion. Intermediate-pressure turbines manage reheated steam at 20-50 bar, with mid-sized blades, while low-pressure turbines feature massive blades—up to 2 meters long—to process low-pressure, high-volume steam, often in twin-flow layouts. This segmentation is standard in gigawatt-scale plants, simplifying maintenance and maximizing efficiency.
The history of steam turbines begins in the late 19th century. Sir Charles Parsons built the first practical multi-stage reaction turbine in 1884, generating 7.5 kW to power a ship’s lights, proving the concept’s potential. Around the same time, Gustaf de Laval developed his impulse turbine, focusing on high-speed applications. By 1900, turbines outpaced reciprocating steam engines in power generation, offering higher efficiency and scalability. Early 20th-century advances in metallurgy—introducing heat-resistant steels—allowed turbines to handle higher temperatures and pressures, scaling to megawatts and driving the electrification of cities. During World War II, steam turbines powered massive ships like the Titanic, with 29,000 horsepower, and continued evolving with innovations like reheat cycles and supercritical steam in the mid-20th century.
Steam turbines have a vast range of applications. In power generation, they produce over 80% of the world’s electricity—37% from coal, 25% from gas in combined-cycle plants, 10% from nuclear, and smaller shares from biomass, geothermal, and solar thermal. Industrially, they drive compressors in chemical plants, pumps in oil refineries, and provide process steam in paper production. Historically, they propelled naval vessels and merchant ships until gas turbines took over post-war. Today, they’re key to renewable energy, powering biomass plants burning wood or agricultural waste, and concentrated solar power systems where mirrors heat a fluid to generate steam.
Design-wise, steam turbines are engineering marvels. Blades vary by stage—high-pressure blades are small, thick, and made of nickel-based superalloys like Inconel 718 to withstand 600°C and resist creep, while low-pressure blades are long, thin, and often titanium to combat erosion from wet steam droplets. Casings are forged steel or alloys, designed to contain high pressures and temperatures, with labyrinth or brush seals minimizing steam leakage around the rotor. The rotor itself, a massive steel forging, must be perfectly balanced to spin at thousands of RPM without vibration, expanding and contracting with heat. Challenges include creep deformation at high temperatures, blade erosion from moisture, and fatigue from years of cyclic loading, driving ongoing material research into ceramics and coatings.
Efficiency in steam turbines hinges on steam conditions and design. A basic system might convert 20-30% of heat into work, losing the rest to exhaust and friction. Advanced plants with supercritical steam—600°C, 300 bar—reach 45-47%, nearing the Rankine cycle’s ideal limit of around 60%. Losses come from heat escaping in the exhaust, turbulence in blade gaps, and moisture in low-pressure stages, which reduces energy output unless countered by superheating or moisture separators. Innovations like reheat cycles, where steam is reheated mid-process, and precise blade profiles designed with computational fluid dynamics, push performance higher.
Steam turbines remain critical today. In fossil-fuel plants, they’re the workhorse for coal and gas, though coal’s role shrinks with climate goals. Nuclear power relies on them to convert reactor heat into electricity, with huge low-pressure turbines handling saturated steam from boiling water reactors. In renewables, they power biomass plants—burning wood or waste—and geothermal facilities, while concentrated solar power uses them to turn sunlight into electricity. Modern advancements include ceramic blades tolerating 1,000°C, 3D-printed components for complex shapes, and AI-driven controls optimizing performance in real time, ensuring turbines stay relevant in a shifting energy landscape.
The advantages of steam turbines are numerous. They deliver massive power—up to 1,750 MW in nuclear units like the Arabelle turbine—run reliably for 30-50 years with maintenance, and adapt to any heat source, from fossil fuels to renewables. However, they’re not without drawbacks. They require complex infrastructure—boilers, condensers, cooling systems—take hours to start up compared to minutes for gas turbines, and need water for cooling, posing challenges in arid regions or with environmental regulations.
A standout example of their scale is the Arabelle turbine, used in French nuclear plants, producing 1,750 MW with low-pressure blades over 2 meters long, powering millions of homes. On a smaller scale, a wood-fired turbine might generate 5 kW for a cabin, burning a few kilograms of wood per hour. From these extremes, steam turbines showcase their versatility and enduring impact. Since Parsons’ first design, they’ve evolved from kilowatts to gigawatts, shaping how we generate power and likely to persist as we balance fossil fuels with sustainable energy.
Steam turbines are remarkable machines that have played a pivotal role in shaping the modern world by converting thermal energy from steam into mechanical work, primarily for generating electricity or driving industrial equipment. Their ability to harness the power of steam—produced by heating water with fuels like coal, gas, nuclear energy, biomass, or even solar heat—makes them one of the most versatile and widely used technologies in energy production. With a history stretching back to the late 19th century, steam turbines have evolved from simple experimental devices into highly efficient, complex systems that power everything from small workshops to entire cities. Let’s continue exploring their mechanics, variations, historical significance, technical details, applications, and their place in today’s energy landscape, diving deeper into each aspect.
The operation of a steam turbine is rooted in the Rankine cycle, a thermodynamic process that efficiently turns heat into usable energy. In a boiler, water is heated under pressure until it becomes steam, reaching temperatures between 200 and 600 degrees Celsius and pressures from 10 to 300 bar, depending on the system’s design and purpose. For example, a small biomass boiler might produce steam at 10 bar and 200°C, while a supercritical coal plant pushes conditions to 300 bar and 600°C for maximum efficiency. This high-pressure steam enters the turbine and flows over blades attached to a rotor—a large, cylindrical shaft. As the steam expands, its pressure and temperature drop, accelerating it into the blades and causing the rotor to spin. The rotor’s speed varies—1,500 RPM for 50 Hz grids, 3,600 RPM for 60 Hz, or even 30,000 RPM in high-speed impulse designs—driving a generator that converts the motion into electricity. After doing its work, the steam exits the turbine, either condensing into water in a closed-loop system or leaving as usable heat for industrial processes.
The blades are the heart of the turbine, and their interaction with steam defines the turbine’s type. In impulse turbines, steam passes through stationary nozzles that accelerate it into high-velocity jets—sometimes exceeding 500 meters per second—before striking curved, bucket-like blades on the rotor. The pressure drop occurs entirely in the nozzles, so the blades only convert kinetic energy into motion. Gustaf de Laval’s single-stage turbine, developed in the 1880s, is a classic impulse design, spinning at extreme speeds like 30,000 RPM to power small generators or pumps. Its simplicity made it a breakthrough, though its efficiency drops with larger steam volumes. The Curtis turbine, another impulse variant, adds velocity compounding—steam bounces between multiple rows of moving and stationary blades, extracting energy step-by-step, reducing speed to 3,000-6,000 RPM for practical use in early 20th-century power plants. Impulse turbines are rugged and ideal for high-pressure steam, but they struggle with lower-pressure conditions or fluctuating loads.
Reaction turbines take a different approach, extracting energy through continuous steam expansion across both fixed and moving blades. Fixed stator blades direct steam onto moving rotor blades, where it accelerates and drops in pressure, creating a reactive force—like a rocket’s thrust—that spins the rotor. Sir Charles Parsons pioneered this in 1884 with a multi-stage design, using dozens or even hundreds of stages to gradually extract energy. Each stage contributes a small pressure drop, with blade sizes growing from a few centimeters in high-pressure sections to over a meter in low-pressure ones, matching the steam’s expansion. The blades are airfoil-shaped, precision-crafted to minimize turbulence, and require tight tolerances—gaps as small as a millimeter—to prevent steam leakage. Reaction turbines shine in large power plants, achieving efficiencies up to 90% of the ideal Rankine cycle, but their complexity and cost make them less practical for small-scale use.
Combination turbines blend these principles for versatility. In the high-pressure stages, an impulse design handles the intense initial conditions—say, 300 bar and 600°C—with nozzles and sturdy blades, while later stages shift to reaction designs as the steam expands to 20 bar or less, optimizing energy extraction across the pressure spectrum. This hybrid setup is common in modern supercritical plants, where steam pushes material limits. Designers use computational tools to smooth the transition, ensuring minimal shock losses as steam behavior changes, making these turbines efficient and compact for utility-scale power generation.
Condensing turbines focus on maximizing power by exhausting steam into a vacuum, typically 0.03 to 0.1 bar, created by a condenser. This low pressure lets the steam expand further, extracting nearly all its energy. The low-pressure stages feature enormous blades—sometimes 1.5 to 2 meters long—spinning at tip speeds near 500 m/s, often in dual-flow configurations where steam splits to opposite sides of the turbine to balance thrust. Condensers use water from rivers or cooling towers, evaporating 1-2% of a plant’s output as waste heat, or air in water-scarce areas, though air-cooling reduces efficiency. These turbines power massive plants—coal, nuclear, or gas-fired—delivering hundreds of megawatts, but they need extensive cooling systems, raising costs and environmental concerns like thermal discharge into waterways.
Back-pressure turbines prioritize dual outputs, exhausting steam at 5 to 20 bar for industrial use—think heating pulp in a paper mill or driving a chemical reactor—rather than condensing it. The turbine’s final stage is tuned to this pressure, with fewer blades than a condensing design, and the exhaust steam retains significant heat, boosting total energy efficiency to 80% or more in combined heat and power systems. They’re simpler, skipping the condenser, and cost-effective where heat is as valuable as power, though their electrical efficiency is lower since less steam energy becomes work.
Extraction turbines add flexibility, tapping steam at intermediate points—say, 15 bar for process heat and 2 bar for boiler feedwater heating—while the rest generates power. Control valves regulate extraction, and the turbine’s staging adjusts for reduced flow in later sections, requiring sophisticated engineering to balance outputs. These are ideal for refineries or district heating, where steam demands shift, offering adaptability at the cost of added complexity.
Reheat turbines enhance efficiency by reheating steam mid-process. After the high-pressure stage drops steam to 20-50 bar, it returns to the boiler, reheating to 540-600°C, then expands through intermediate- and low-pressure stages. This raises the cycle’s average temperature, lifting efficiency by 4-5% for one reheat or 7% for two, though double-reheat adds cost and rarely justifies itself outside ultra-efficient plants. Reheat systems need extra piping and moisture separators—wet steam after reheating erodes blades—making them standard in large fossil-fuel stations chasing every percentage point of fuel savings.
Large turbines often split into high-pressure, intermediate-pressure, and low-pressure sections on one shaft, each tailored to its steam range. High-pressure turbines use small, thick blades—10-20 cm long—to handle 300 bar and 600°C, made of nickel alloys like Inconel to resist creep and corrosion. Intermediate-pressure turbines, with mid-sized blades, manage reheated steam at 20-50 bar, while low-pressure turbines have huge blades—up to 2 meters—to process low-pressure, high-volume steam, often splitting flow to twin exhausts. This modular design dominates gigawatt-scale plants, simplifying repairs and optimizing performance across the steam’s journey.
Historically, steam turbines emerged during the Second Industrial Revolution. Parsons’ 1884 reaction turbine, generating 7.5 kW, powered a ship’s lights, proving multi-stage efficiency. De Laval’s impulse design, from the same era, targeted high-speed niches. By 1900, turbines outpaced steam engines, scaling to megawatts with better steels and boiler tech. The 20th century saw them power ships—the Titanic’s 29,000 hp turbines drove it across the Atlantic—and electrify nations, with innovations like reheat cycles (1930s) and supercritical steam (1950s) pushing efficiency from 20% to over 45%.
Applications span industries. In power generation, steam turbines produce over 80% of global electricity—37% from coal, 25% from gas, 10% from nuclear, plus biomass, geothermal, and solar thermal. Industrially, they run compressors in chemical plants, pumps in oil fields, and supply steam in paper mills. They once dominated marine propulsion, powering warships and liners until gas turbines took over post-1940s. Today, they’re key to renewables—biomass plants burn wood or waste, while solar thermal systems use mirrors to heat steam—bridging fossil and green energy.
Design details reveal their complexity. High-pressure blades, small and robust, use superalloys like Inconel 718 or Rene 41, tolerating 600°C for decades, with chromium coatings fighting corrosion. Low-pressure blades, long and thin, use titanium to resist wet-steam erosion, with tips moving at supersonic speeds. Casings, forged from steel or alloys, contain pressures up to 300 bar, with labyrinth seals—layered grooves—cutting leakage. Rotors, weighing tons, are precision-balanced to spin at 3,000 RPM without vibration, expanding inches from heat. Challenges include creep (slow deformation at high heat), erosion (wet steam pitting blades), and fatigue (cracks from cyclic stress), driving research into ceramics, which handle 1,000°C, and protective coatings.
Efficiency varies widely. Small turbines hit 20-30%, losing heat to exhaust and friction. Advanced plants with supercritical steam—600°C, 300 bar—reach 45-47%, nearing the Rankine ideal of 60%. Losses stem from exhaust heat, blade-gap leaks, and moisture in low-pressure stages, which drops output unless mitigated by superheating to 540°C or separators removing water droplets. Reheat cycles, supercritical conditions, and CFD-optimized blades—curved for minimal turbulence—push performance, with top plants achieving over 90% of theoretical efficiency.
Today, steam turbines remain vital. Fossil-fuel plants—coal and gas—rely on them, though coal declines with emissions goals. Nuclear plants use them to turn reactor heat into power, with massive low-pressure turbines handling saturated steam at 70 bar. Renewables lean on them—biomass burns wood for 1-50 MW, geothermal taps underground steam, and solar thermal generates steam from sunlight. Innovations keep them competitive: ceramic blades for higher heat, 3D-printed parts for precision, and AI controls for real-time optimization, ensuring they adapt to a decarbonizing world.
Their strengths are clear—massive output (1,750 MW in nuclear units), longevity (30-50 years), and fuel flexibility. But they’re not perfect—complex setups with boilers and condensers, slow startups (hours vs. minutes for gas turbines), and water-heavy cooling limit them in dry areas or fast-response grids. The Arabelle turbine, at 1,750 MW, powers 2 million homes with 2-meter blades, while a 5 kW wood-fired unit lights a cabin—proof of their range. From Parsons’ 7.5 kW to today’s giants, steam turbines have electrified the world and will evolve as we balance legacy fuels with sustainability.
Industrial Steam Turbine
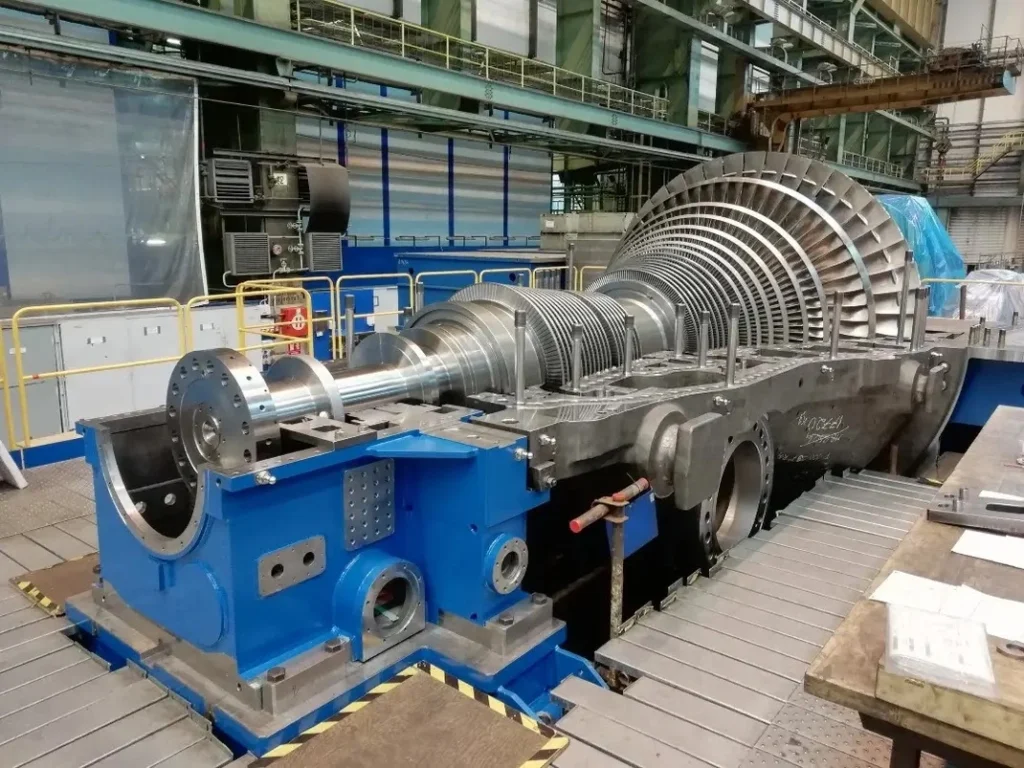
Industrial steam turbines are a specialized subset of steam turbines designed specifically for use in industrial settings, where they generate electricity, drive machinery, or provide process steam for manufacturing and production processes. Unlike utility-scale turbines found in large power plants, which focus on maximizing electrical output for the grid, industrial steam turbines are tailored to meet the unique energy demands of factories, refineries, chemical plants, and other industrial facilities. They prioritize flexibility, efficiency in combined heat and power (CHP) applications, and integration with industrial processes, often operating at smaller scales—ranging from a few kilowatts to tens of megawatts—while still leveraging the same fundamental principles as their larger counterparts. Below is a detailed exploration of industrial steam turbines, covering their mechanics, types, applications, design features, and significance in modern industry.
Industrial steam turbines work by converting thermal energy from steam into mechanical energy, typically within the framework of the Rankine cycle. Steam is produced in a boiler heated by fuels like natural gas, coal, biomass (such as wood or agricultural waste), oil, or waste heat from industrial processes. The steam, pressurized to between 5 and 100 bar and heated to 200-540°C, enters the turbine and expands across a series of blades mounted on a rotor. This expansion drives the rotor, spinning it at speeds like 3,000 RPM (for 50 Hz systems) or 3,600 RPM (60 Hz), which then powers a generator for electricity or directly drives equipment such as pumps, compressors, or fans. The steam’s exit path depends on the turbine’s purpose—some exhaust into a condenser for maximum power, while others release steam at usable pressures (e.g., 2-20 bar) for heating or process use, a hallmark of industrial applications.
The types of industrial steam turbines reflect their adaptability to factory needs. Back-pressure turbines are common, exhausting steam at a higher pressure—say, 5-20 bar—rather than condensing it, so the exhaust can heat buildings, dry materials, or power downstream processes. For example, in a paper mill, a back-pressure turbine might generate 5 MW of electricity while supplying 10 tons/hour of 10-bar steam to dry pulp, achieving total energy efficiency above 80% when heat is included, though electrical efficiency alone might be 20-30%. Extraction turbines offer more versatility, allowing steam to be tapped at intermediate stages—perhaps 15 bar for a chemical reactor and 3 bar for preheating—while the rest generates power. These turbines use control valves to adjust extraction flow, balancing electricity and steam output, making them ideal for refineries or food processing plants with variable demands.
Condensing turbines, though less frequent in industrial settings, are used when electricity is the primary goal. They exhaust steam into a vacuum (0.05-0.1 bar) via a condenser, maximizing energy extraction with efficiencies up to 35-40%. A small factory might use a 1 MW condensing turbine to power its grid, condensing steam with a water-cooled system tied to a cooling tower. Combination turbines, blending impulse and reaction designs, also appear in larger industrial setups—impulse stages handle high-pressure steam (e.g., 50 bar), transitioning to reaction stages for lower pressures, optimizing performance in plants with diverse steam conditions. Single-stage turbines, simple and compact, suit small applications like a 50 kW unit driving a pump, while multi-stage designs dominate in bigger systems needing higher efficiency, such as a 10 MW turbine in a steel mill.
Applications of industrial steam turbines are vast and tied to specific industries. In chemical plants, they generate power (e.g., 2-20 MW) while supplying steam for reactions or distillation, often using extraction turbines to match process needs. Pulp and paper mills rely on back-pressure turbines, producing 5-15 MW of electricity and steam at 5-10 bar to dry paper, leveraging wood waste as fuel for sustainability. Oil refineries use extraction or condensing turbines, delivering 10-50 MW and steam for cracking or heating, often fueled by refinery byproducts. Sugar mills burn bagasse (cane waste) to power turbines—typically 1-5 MW—while heating juice evaporators. Textile factories use small turbines (100 kW-1 MW) for power and steam dyeing, and food processing plants like breweries employ CHP turbines for electricity and sterilization heat. Mining operations drive compressors or pumps with turbines, often in remote areas using diesel or biomass.
Design features of industrial steam turbines reflect their practical demands. They’re typically smaller than utility turbines—ranging from 50 kW to 50 MW—making them compact enough to fit within factory footprints. Blades vary by stage: high-pressure blades, 5-20 cm long, use nickel alloys like Inconel to handle 540°C and 100 bar, resisting creep and corrosion, while low-pressure blades, up to 1 meter, might use stainless steel or titanium for durability against wet steam. Rotors, forged from steel, spin at 3,000-10,000 RPM, balanced to minimize vibration, with couplings or gearboxes linking to machinery. Casings, made of cast steel, withstand pressures up to 100 bar, with seals like labyrinth designs reducing steam leaks. Boilers are often integrated, sized for 1-100 tons/hour of steam, and fuel flexibility—natural gas, biomass, or waste heat—lets them adapt to local resources. Control systems, increasingly digital, adjust steam flow and extraction in real time, optimizing for shifting loads.
Efficiency in industrial steam turbines depends on their role. Electrical efficiency ranges from 20-40%, lower than utility turbines (45%+), due to smaller scale and heat-focused designs. However, in CHP setups, total efficiency—power plus usable heat—can hit 70-85%, far surpassing condensing-only systems. For instance, a 5 MW back-pressure turbine might convert 25% of fuel energy to electricity and 50% to process steam, losing only 25% to waste heat. Losses come from exhaust heat, friction, and blade-gap leaks, mitigated by superheating steam to 540°C or using moisture separators to dry low-pressure steam. Multi-stage designs with 5-20 stages extract energy gradually, boosting efficiency over single-stage units, though at higher cost.
Historically, industrial steam turbines evolved alongside the Industrial Revolution. Early 20th-century factories used steam engines, but by the 1910s, turbines—building on Parsons’ and De Laval’s work—offered better efficiency and power density. World War II saw them power factories and ships, often fueled by coal or oil. Post-war, they adapted to industrial CHP, with the 1970s energy crisis spurring biomass and waste-heat use. Today, they’re modernizing with automation and sustainable fuels, reflecting industry’s push for efficiency and lower emissions.
Their significance in modern industry is profound. They provide on-site power, reducing grid reliance and costs—critical in remote or unstable regions. CHP capability slashes energy bills; a 10 MW turbine might save a factory $1-2 million yearly by reusing steam. Fuel flexibility supports sustainability—biomass turbines burn wood chips or sawdust, cutting fossil fuel use, while waste-heat recovery harnesses exhaust from furnaces or engines. In a steel plant, a 20 MW turbine might use blast furnace gas, generating power and steam without extra fuel. Reliability is key—turbines run 20-30 years with maintenance, handling 24/7 loads in harsh conditions.
Challenges exist. Initial costs are high—a 5 MW system might run $2-5 million, including boiler and installation—though payback comes in 3-7 years with CHP savings. Maintenance involves blade inspections, seal replacements, and boiler cleaning, costing $50,000-$100,000 yearly for a mid-sized unit. Emissions—CO2, particulates—require scrubbers or filters to meet regulations, especially with biomass or coal. Space needs—boilers, turbines, condensers—limit use in cramped facilities, and startup times (30 minutes to hours) lag behind gas turbines’ responsiveness.
Examples highlight their range. A 2 MW extraction turbine in a chemical plant burns natural gas, producing 1.5 MW electricity and 5 tons/hour of 10-bar steam for reactors, with a $1 million setup cost offset by $300,000 annual savings. A 500 kW back-pressure turbine in a sawmill uses wood waste, powering tools and drying lumber, costing $200,000 but paying back in two years. A 10 MW condensing turbine in a refinery, fueled by oil residues, runs at 35% efficiency, powering pumps and compressors with a water-cooled condenser.
Industrial steam turbines bridge power and process needs, offering tailored solutions where utility turbines don’t fit. Their adaptability—handling diverse fuels, scales, and outputs—keeps them vital in manufacturing, from small workshops to sprawling plants. As industries chase efficiency and sustainability, these turbines evolve with smarter controls, tougher materials, and greener fuels, ensuring they remain a workhorse of industrial energy.
Industrial steam turbines are robust, versatile machines engineered to meet the specific energy demands of industrial facilities, converting steam’s thermal energy into mechanical work for electricity generation, machinery operation, or process steam supply. Unlike their utility-scale counterparts focused on massive grid power, industrial steam turbines are designed for flexibility, often prioritizing combined heat and power (CHP) efficiency and integration with manufacturing processes. Found in factories, refineries, and mills, they range from compact units producing a few kilowatts to larger systems delivering tens of megawatts, balancing power needs with industrial heat requirements. Let’s delve deeper into their mechanics, types, applications, design intricacies, historical context, modern role, and operational details, expanding on their significance in industry.
The core operation of an industrial steam turbine follows the Rankine cycle, a thermodynamic process tailored to industrial settings. A boiler generates steam by burning fuels like natural gas, coal, oil, biomass (e.g., wood chips, bagasse), or capturing waste heat from processes like furnace exhausts. Steam conditions vary—small systems might produce 5-20 bar at 200-300°C, while larger ones reach 50-100 bar at 500-540°C, depending on efficiency goals and fuel quality. This steam enters the turbine, flowing over blades fixed to a rotor. As it expands, its pressure drops—say, from 50 bar to 5 bar—accelerating across the blades and spinning the rotor at speeds like 3,000 RPM (50 Hz grids), 3,600 RPM (60 Hz), or higher (up to 10,000 RPM) in smaller, high-speed units. The rotor drives a generator for electricity or directly powers equipment—pumps, fans, compressors—via a shaft or gearbox. Exiting steam either condenses in a vacuum (0.05-0.1 bar) for maximum power or leaves at usable pressures (2-20 bar) for heating, drying, or chemical reactions, a key feature in industrial CHP systems.
Types of industrial steam turbines are defined by their steam handling and output priorities. Back-pressure turbines exhaust steam at elevated pressures—typically 5-20 bar—rather than condensing it, supplying heat for industrial tasks. In a textile plant, a 3 MW back-pressure turbine might burn biomass, generating 2.5 MW of electricity while providing 8 tons/hour of 10-bar steam to dye fabrics, achieving 75-85% total efficiency by reusing heat, though electrical efficiency stays at 20-30%. Extraction turbines add control, tapping steam at multiple stages—e.g., 15 bar for a distillation column, 3 bar for boiler feedwater heating—while the rest powers the rotor. A 10 MW extraction turbine in a refinery could produce 8 MW and extract 20 tons/hour of steam at two pressures, using valves to adjust flow based on demand, ideal for fluctuating process needs. Condensing turbines, less common industrially, focus on power, exhausting into a condenser’s vacuum to hit 35-40% electrical efficiency. A 5 MW condensing turbine in a mining operation might power compressors, using a water-cooled condenser tied to a nearby stream.
Combination turbines, mixing impulse and reaction designs, suit larger industrial systems. High-pressure stages (e.g., 50 bar) use impulse blades—steam jets from nozzles onto buckets—while lower-pressure stages (e.g., 10 bar) shift to reaction blades, where steam expands across moving blades for efficiency. A 15 MW combination turbine in a chemical plant might handle 540°C steam, optimizing energy extraction across stages. Single-stage turbines, with one blade set, are compact and simple—think a 100 kW unit driving a pump in a small factory—while multi-stage turbines, with 5-20 stages, boost efficiency in bigger setups like a 20 MW sugar mill turbine. Reheat turbines, rare industrially due to cost, reheat steam mid-process (e.g., from 20 bar back to 540°C), lifting efficiency by 4-5%, used in high-output plants like steelworks needing every edge.
Applications tie directly to industrial sectors. Chemical plants use 2-20 MW turbines for power and steam—e.g., a 5 MW extraction unit supplies 10-bar steam for reactors while running agitators. Pulp and paper mills favor back-pressure turbines—say, a 10 MW unit burning wood waste, producing 8 MW and 15 tons/hour of 5-bar steam to dry paper. Oil refineries deploy 10-50 MW turbines, often extraction or condensing, using byproduct fuels like petroleum coke to power pumps and supply steam for cracking. Sugar mills burn bagasse for 1-5 MW turbines, driving mills and heating evaporators—e.g., a 3 MW unit processes 500 tons of cane daily. Textile plants use 100 kW-1 MW turbines for power and steam dyeing, while food processors like dairies run 500 kW-2 MW CHP turbines for sterilization and electricity. Mining sites power compressors or hoists with 1-10 MW turbines, often in remote areas using diesel or local biomass.
Design specifics reflect industrial practicality. Sizes range from 50 kW to 50 MW—smaller than utility turbines (100 MW+)—fitting tight factory spaces. High-pressure blades, 5-20 cm long, use nickel-based superalloys (e.g., Inconel 718) to handle 540°C and 100 bar, resisting creep and oxidation, often coated with chromium carbide for durability. Low-pressure blades, up to 1 meter, use stainless steel or titanium to combat wet-steam erosion, with tips moving at 300-500 m/s. Rotors, forged from high-strength steel, weigh tons yet spin precisely, balanced to avoid vibration, with couplings or gearboxes (e.g., reducing 10,000 RPM to 1,500 RPM) linking to loads. Casings, cast steel or alloys, contain pressures up to 100 bar, with labyrinth seals—concentric grooves—cutting leakage by 5-10%. Boilers, producing 1-100 tons/hour of steam, integrate with turbines, burning diverse fuels—natural gas (clean, efficient), biomass (sustainable), or waste heat (free energy). Digital controls monitor steam flow, pressure, and extraction, adjusting in seconds for optimal output.
Efficiency balances power and heat. Electrical efficiency spans 20-40%—a 5 MW condensing turbine might hit 35%, converting 35% of fuel energy to power, losing 65% to exhaust and friction. CHP systems shine, with back-pressure or extraction turbines reaching 70-85% total efficiency—e.g., a 3 MW unit turns 25% into electricity, 50% into usable steam, losing 25% to waste. Losses stem from exhaust heat, blade turbulence, and moisture—wet steam in low-pressure stages cuts output by 5-10% unless superheated to 540°C or dried with separators. Multi-stage designs extract energy gradually—5-20 stages vs. 1—lifting efficiency by 10-15% over single-stage units, though complexity rises. Fuel quality matters—dry biomass (4.5 kWh/kg) outperforms wet wood (3 kWh/kg), reducing steam output if moisture exceeds 20%.
Historically, industrial steam turbines grew from late 19th-century innovations. Parsons’ 1884 reaction turbine and De Laval’s impulse design laid the groundwork, but by the 1910s, factories adopted turbines over steam engines for higher efficiency—10-20% vs. 5-10%. Early 20th-century mills used coal-fired turbines for power and heat, scaling to megawatts by the 1930s. WWII spurred industrial use—turbines ran factories on coal or oil when grids faltered. The 1970s energy crisis boosted CHP and biomass turbines, cutting costs as oil prices soared. Today, they align with sustainability—waste-heat turbines in steelworks or biomass units in sawmills reflect greener priorities.
Their modern role is critical. On-site power cuts grid dependence—a 5 MW turbine saves a factory $500,000-$1 million yearly vs. utility rates. CHP slashes energy costs—a 10 MW unit might recover $2 million annually in heat value. Sustainability drives adoption—biomass turbines burn wood waste (1-2 tons/hour for 5 MW), reducing fossil fuel use, while waste-heat units harness kiln exhaust, needing no extra fuel. Reliability stands out—turbines run 20-30 years, handling 8,000 hours/year in harsh conditions like dusty mines or humid mills. A 15 MW turbine in a petrochemical plant might use flare gas, producing power and steam without added emissions.
Challenges persist. Costs are steep—a 5 MW system with boiler and piping runs $2-5 million, though CHP payback hits 3-5 years. Maintenance—blade checks, seal swaps, boiler descaling—costs $50,000-$150,000 yearly, with downtime cutting output. Emissions require controls—biomass emits 50-200 mg/m³ of particulates, needing $100,000 scrubbers for compliance (e.g., U.S. EPA limits at 25 mg/m³). Space demands—10×20 meters for a 5 MW setup—clash with cramped plants, and startups take 30-60 minutes, lagging gas turbines’ 5-10 minutes. Fuel supply matters—wet biomass or inconsistent waste heat drops performance.
Examples illustrate their impact. A 1 MW back-pressure turbine in a dairy burns gas, producing 800 kW and 3 tons/hour of 5-bar steam for pasteurization, costing $500,000 but saving $150,000 yearly. A 20 MW extraction turbine in a steel mill uses blast furnace gas, delivering 15 MW and 30 tons/hour of 15-bar steam for rolling, with a $10 million cost offset by $3 million annual savings. A 200 kW condensing turbine in a remote mine, diesel-fueled, powers drills at 35% efficiency, costing $100,000 but cutting generator fuel by 50%.
Industrial steam turbines are industrial energy’s unsung heroes, blending power and heat with unmatched flexibility. Their evolution—from coal-fired mills to biomass-driven plants—mirrors industry’s shift to efficiency and sustainability. With smart controls, tough materials, and green fuels, they’ll keep factories humming, proving their worth in a changing world.
Industrial steam turbines are highly specialized machines engineered to meet the diverse energy needs of industrial environments, transforming the thermal energy of steam into mechanical work to generate electricity, power machinery, or supply process steam for manufacturing. Unlike utility-scale turbines that dominate large power plants with outputs in the hundreds of megawatts, industrial steam turbines are typically smaller—ranging from tens of kilowatts to 50 megawatts—and are designed for flexibility, often prioritizing combined heat and power (CHP) efficiency over raw electrical output. They serve as critical assets in factories, refineries, mills, and mines, integrating seamlessly with industrial processes to provide on-site power and heat. Let’s expand further on their mechanics, types, applications, design details, historical evolution, modern significance, and operational nuances, diving deeper into their role in industry.
The operation of an industrial steam turbine is based on the Rankine cycle, adapted to industrial demands. Steam is generated in a boiler using fuels such as natural gas, coal, oil, biomass like wood chips or bagasse, or waste heat from industrial processes—think exhaust from a cement kiln or refinery flare gas. Steam conditions vary widely: a small system might produce 5-20 bar at 200-300°C, suitable for a food processing plant, while a larger one reaches 50-100 bar at 500-540°C, common in chemical manufacturing. This steam enters the turbine, flowing over blades attached to a rotor—a heavy steel shaft. As it expands—dropping from, say, 50 bar to 5 bar—it accelerates, pushing the blades and spinning the rotor at speeds like 3,000 RPM for 50 Hz electrical grids, 3,600 RPM for 60 Hz, or up to 10,000 RPM in high-speed industrial units. The rotor’s motion drives a generator for electricity or directly powers equipment—pumps, compressors, fans—via a shaft, often with a gearbox to match equipment speeds. Exiting steam either condenses into a vacuum (0.05-0.1 bar) for maximum power or leaves at usable pressures (2-20 bar) for heating or process use, a defining trait of industrial turbines in CHP setups.
Types of industrial steam turbines are tailored to specific industrial roles. Back-pressure turbines exhaust steam at higher pressures—typically 5-20 bar—rather than condensing it, supplying heat for tasks like drying lumber or heating chemical vats. A 5 MW back-pressure turbine in a paper mill might burn wood waste, producing 4 MW of electricity and 10 tons/hour of 10-bar steam, hitting 80% total efficiency by reusing heat, though electrical efficiency is 25-30%. Extraction turbines provide more control, tapping steam at multiple points—e.g., 15 bar for a distillation tower, 3 bar for preheating boiler water—while the rest generates power. A 10 MW extraction turbine in a petrochemical plant could deliver 7 MW and 15 tons/hour of steam at two pressures, using automated valves to adjust flow, perfect for variable process demands. Condensing turbines, less common in industry, exhaust into a condenser’s vacuum, maximizing power at 35-40% efficiency. A 3 MW condensing turbine in a mining camp might power lighting and drills, using a water-cooled condenser tied to a local river.
Combination turbines mix impulse and reaction designs for larger systems. High-pressure stages (e.g., 50 bar, 540°C) use impulse blades—steam jets from nozzles onto buckets—while lower-pressure stages (e.g., 10 bar) shift to reaction blades, where steam expands across moving blades, boosting efficiency. A 20 MW combination turbine in a steel mill might optimize steam from blast furnace gas across 10 stages. Single-stage turbines, with one blade set, are simple and compact—say, a 200 kW unit driving a fan—while multi-stage turbines, with 5-20 stages, enhance efficiency in bigger setups like a 15 MW refinery turbine. Reheat turbines, though rare industrially, reheat steam mid-process—e.g., from 20 bar back to 540°C—adding 4-5% efficiency, used in high-value plants like fertilizer production needing top performance.
Applications span key industries. Chemical plants use 2-20 MW turbines for power and steam—e.g., a 5 MW extraction unit supplies 12-bar steam for reactors while running pumps. Pulp and paper mills rely on back-pressure turbines—say, a 10 MW unit burning sawdust, producing 8 MW and 20 tons/hour of 5-bar steam to dry pulp. Oil refineries deploy 10-50 MW turbines, often extraction or condensing, using byproduct fuels like heavy oil to power compressors and supply steam for cracking. Sugar mills burn bagasse for 1-5 MW turbines—e.g., a 2 MW unit processes 400 tons of cane daily, heating evaporators. Textile plants use 100 kW-1 MW turbines for power and steam dyeing, while food processors like breweries run 500 kW-2 MW CHP turbines for bottling and sterilization. Mining operations power hoists or ventilation with 1-10 MW turbines, often in remote sites using diesel or local wood.
Design details highlight their industrial focus. Sizes range from 50 kW to 50 MW, compact enough for factory floors—e.g., a 5 MW unit fits in a 10×20-meter space. High-pressure blades, 5-20 cm long, use nickel alloys like Inconel 718 or Hastelloy, handling 540°C and 100 bar, resisting creep and oxidation, often coated with ceramic or chromium for longevity. Low-pressure blades, up to 1 meter, use stainless steel or titanium, with tips at 300-500 m/s resisting wet-steam erosion. Rotors, forged from high-strength steel, weigh 1-10 tons, balanced to spin at 3,000-10,000 RPM, with couplings or gearboxes (e.g., 5:1 ratio) matching equipment speeds. Casings, cast steel or alloys, contain 100 bar, with labyrinth seals reducing leakage by 5-10%. Boilers, producing 1-100 tons/hour, integrate tightly—natural gas offers clean burns, biomass leverages waste, waste heat taps free energy. Digital controls—PLCs or SCADA—monitor pressure, temperature, and flow, adjusting in milliseconds for load shifts.
Efficiency balances electrical and thermal output. Electrical efficiency ranges from 20-40%—a 5 MW condensing turbine hits 35%, losing 65% to exhaust and friction, while CHP systems like back-pressure turbines reach 70-85% total efficiency. A 3 MW unit might convert 25% to power, 50% to 10-bar steam, losing 25% to waste. Losses include exhaust heat, blade turbulence, and moisture—wet steam cuts output by 5-10% unless superheated to 540°C or dried with separators. Multi-stage designs—5-20 stages—lift efficiency by 10-15% over single-stage, though costs rise. Fuel impacts performance—dry biomass (4.5 kWh/kg) beats wet (3 kWh/kg), with moisture above 20% slashing steam quality.
Historically, industrial steam turbines emerged from late 19th-century breakthroughs. Parsons’ 1884 reaction turbine and De Laval’s impulse design set the stage, but by the 1910s, factories swapped steam engines for turbines—10-20% efficiency vs. 5-10%. Early 20th-century coal-fired turbines powered mills, scaling to megawatts by the 1930s. WWII saw turbines run factories on oil or coal when grids failed. The 1970s energy crisis drove CHP adoption—biomass and waste-heat turbines cut oil reliance. Today, they align with green goals—wood-fired turbines in sawmills or waste-heat units in glassworks reduce carbon footprints.
Their modern significance is multifaceted. On-site power slashes costs—a 5 MW turbine saves $500,000-$1 million yearly vs. grid power. CHP doubles value—a 10 MW unit recovers $2-3 million in heat annually. Sustainability shines—biomass turbines burn 1-2 tons/hour of waste for 5 MW, while waste-heat units use kiln exhaust, needing no fuel. Reliability is unmatched—turbines run 20-30 years, 8,000 hours/year, in dust, heat, or humidity. A 15 MW turbine in a fertilizer plant might use ammonia process heat, producing power and steam emissions-free.
Challenges remain. Costs hit $2-5 million for a 5 MW system—boiler, turbine, piping—though CHP payback is 3-5 years. Maintenance—blade checks, seal swaps, boiler cleaning—runs $50,000-$150,000 yearly, with 1-2 days downtime. Emissions need controls—biomass emits 50-200 mg/m³ particulates, requiring $100,000 filters for compliance (e.g., EU limits at 20 mg/m³). Space—10×20 meters for 5 MW—strains tight plants, and startups (30-60 minutes) lag gas turbines (5-10 minutes). Fuel logistics—wet biomass or uneven waste heat—cut output if inconsistent.
Examples show their range. A 1 MW back-pressure turbine in a dairy burns gas, producing 800 kW and 3 tons/hour of 5-bar steam for pasteurization, costing $500,000 but saving $150,000 yearly. A 20 MW extraction turbine in a steel mill uses furnace gas, delivering 15 MW and 30 tons/hour of 15-bar steam, costing $10 million but saving $3 million annually. A 200 kW condensing turbine in a remote mine, diesel-fueled, powers drills at 35% efficiency, costing $100,000 but halving fuel use.
Industrial steam turbines are industrial energy’s backbone, merging power and heat with precision. Their evolution—from coal-fired mills to biomass-driven plants—tracks industry’s efficiency and sustainability push. With smart tech, durable materials, and green fuels, they’ll keep driving production, proving their enduring value.
Steam Turbine Generator
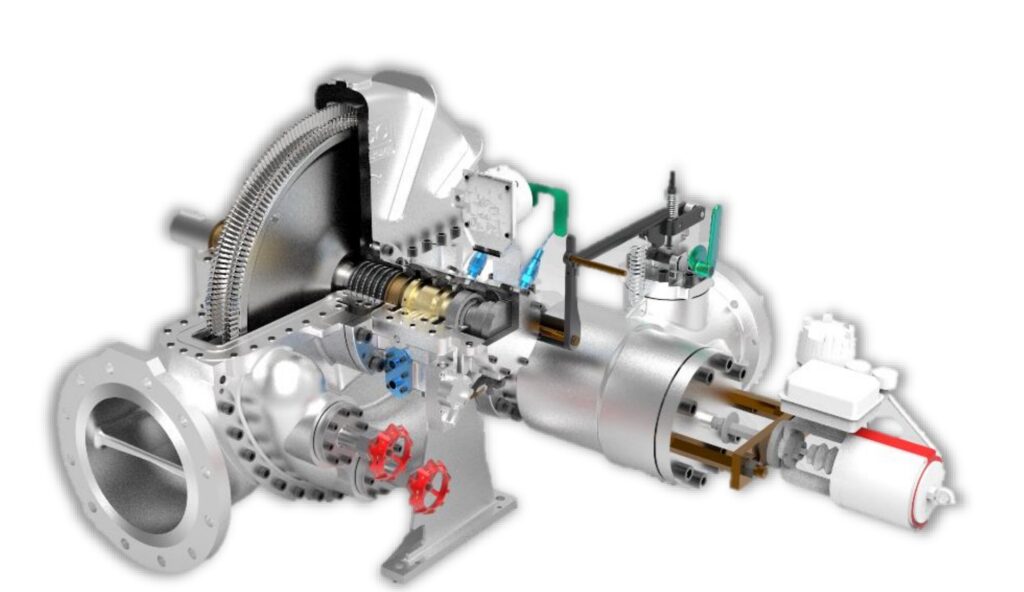
It seems like you’re asking for a detailed exploration of steam turbine generators, which are systems where a steam turbine is paired with an electric generator to produce electricity. Given our prior discussions, I’ll assume you want a general, informative overview of steam turbine generators, building on the foundation of steam turbines but focusing on their role in power generation, particularly in industrial and utility contexts. I’ll cover their mechanics, types, applications, design specifics, historical development, and modern significance, providing a comprehensive picture without tying it strictly to previous topics unless relevant. Here’s an in-depth look at steam turbine generators.
Steam turbine generators are integrated systems that convert thermal energy from steam into electrical energy, combining a steam turbine’s mechanical power with a generator’s ability to produce electricity. They are a cornerstone of modern power generation, used in industrial plants, utility-scale power stations, and even smaller off-grid setups, harnessing heat from fuels like coal, natural gas, nuclear reactions, biomass, or solar thermal sources. The steam turbine spins a rotor, which drives the generator to produce electricity, typically synchronized with electrical grids at 50 Hz (3,000 RPM) or 60 Hz (3,600 RPM). These systems range from small units generating a few kilowatts to massive installations producing over 1,000 megawatts, making them vital to both industrial self-sufficiency and global energy supply.
The mechanics of a steam turbine generator begin with the Rankine cycle. A boiler heats water using a heat source—say, burning coal at 1,200°C or nuclear fission at 300°C—producing high-pressure steam at 10-300 bar and 200-600°C, depending on the system. This steam enters the turbine, flowing over blades on a rotor. As it expands—dropping from, say, 100 bar to 0.05 bar—it accelerates, pushing the blades and spinning the rotor at speeds like 3,000-10,000 RPM. The rotor connects to a generator via a shaft, often with a gearbox in smaller units to adjust speed. Inside the generator, the rotor’s motion—surrounded by a stator with copper windings—induces an electromagnetic field, generating alternating current (AC) electricity. Steam exits either into a condenser (cooling it back to water for reuse) or at a usable pressure for industrial processes in CHP setups, with the condenser path maximizing electrical output.
Types of steam turbine generators reflect their turbine designs and applications. Condensing steam turbine generators exhaust steam into a vacuum (0.05-0.1 bar) via a condenser, optimizing power output at 35-45% efficiency. A 500 MW coal plant might use a multi-stage condensing turbine with high-pressure (HP), intermediate-pressure (IP), and low-pressure (LP) sections, each with blades tailored to steam conditions—small HP blades for 300 bar, huge LP blades for 0.05 bar. Back-pressure steam turbine generators exhaust at 5-20 bar for industrial heat, common in factories. A 5 MW unit in a paper mill might produce 4 MW and 10 tons/hour of 10-bar steam, hitting 80% total efficiency in CHP mode. Extraction steam turbine generators tap steam at intermediate pressures—e.g., 15 bar and 3 bar—while generating power, ideal for refineries needing both. A 10 MW extraction unit might deliver 8 MW and 20 tons/hour of steam.
Combination steam turbine generators blend impulse and reaction designs. High-pressure stages use impulse blades—steam jets from nozzles onto buckets—while lower stages use reaction blades, where steam expands across moving blades, common in industrial or mid-sized utility systems like a 50 MW biomass plant. Reheat steam turbine generators reheat steam mid-process—e.g., from 20 bar back to 540°C—boosting efficiency by 4-5%, used in large fossil-fuel plants like a 1,000 MW coal unit. Single-stage units, simple and compact, suit small setups—say, a 100 kW generator—while multi-stage designs with 5-50 stages dominate larger systems for efficiency, like a 200 MW nuclear generator.
Applications span scales and sectors. Utility power plants use massive steam turbine generators—coal (37% of global power), gas (25%), nuclear (10%)—producing 100-1,750 MW. A 1,200 MW nuclear plant might power 1.5 million homes, with three LP turbines exhausting to condensers. Industrial facilities deploy smaller units—50 kW to 50 MW—for on-site power and heat. A 5 MW CHP generator in a chemical plant burns gas, supplying 4 MW and steam for reactors. Biomass plants burn wood or waste—e.g., a 10 MW unit uses 2 tons/hour of wood chips—supporting rural grids or factories. Geothermal and solar thermal plants use steam turbine generators—5-100 MW—converting earth or sun heat into power. Off-grid systems, like a 50 kW unit in a remote mine, provide backup or standalone electricity.
Design specifics tie the turbine and generator into a cohesive unit. Turbine blades vary—HP blades (5-20 cm) use nickel alloys like Inconel 718 for 600°C and 300 bar, resisting creep, while LP blades (up to 2 meters) use titanium to handle wet steam at 500 m/s tip speeds. Rotors, forged steel, spin at 3,000-10,000 RPM, balanced to avoid vibration, with couplings or gearboxes (e.g., 5:1 ratio) linking to generators. Generators use synchronous designs—rotors with magnetic poles spin within stator windings—producing AC at 50/60 Hz, with outputs from 400 V (small units) to 13.8 kV (large plants). Casings, steel or alloys, contain 300 bar, with seals like labyrinths cutting leakage. Boilers, producing 1-1,000 tons/hour of steam, integrate with fuel systems—coal, gas, biomass—or heat recovery units. Cooling systems—water towers or air fans—support condensing designs, rejecting 50-60% of heat as waste.
Efficiency hinges on turbine design and steam conditions. Condensing units hit 35-45%—a 1,000 MW coal generator converts 40% of fuel energy to electricity, losing 60% to exhaust and friction. CHP units reach 70-85% total efficiency—e.g., a 5 MW back-pressure generator turns 25% into power, 50% into steam, losing 25%. Losses include exhaust heat, blade turbulence, and moisture—wet steam cuts output by 5-10% unless superheated to 540°C or dried. Multi-stage turbines (10-50 stages) extract energy gradually, boosting efficiency by 10-15% over single-stage. Supercritical steam (600°C, 300 bar) in large units pushes electrical efficiency to 47%, nearing the Rankine ideal of 60%.
Historically, steam turbine generators trace to the late 19th century. Sir Charles Parsons’ 1884 turbine, paired with a dynamo, generated 7.5 kW, proving the concept. Gustaf de Laval’s impulse design followed, powering early generators. By 1900, turbines outpaced steam engines, scaling to megawatts with better steels. The 20th century saw growth—coal-fired units electrified cities, nuclear turbines emerged in the 1950s, and reheat cycles (1930s) lifted efficiency. WWII used them in ships and factories, while the 1970s energy crisis spurred industrial CHP adoption.
Today, steam turbine generators remain essential. Utility plants—coal, gas, nuclear—rely on them for baseload power, though coal declines with emissions rules. Industrial CHP units save costs—a 10 MW generator cuts a factory’s $2 million yearly energy bill by half. Renewables use them—biomass (1-50 MW), geothermal (5-100 MW), and solar thermal (10-200 MW)—supporting green grids. Innovations enhance them—ceramic blades handle 1,000°C, 3D-printed parts improve precision, and AI optimizes performance. The Arabelle generator, at 1,750 MW, powers 2 million homes with 2-meter LP blades, while a 50 kW biomass unit lights a village.
Advantages include high output (up to 1,750 MW), longevity (30-50 years), and fuel flexibility. Challenges are cost ($2-10 million for 5-50 MW), complexity (boilers, condensers), and slow startups (hours vs. minutes for gas turbines). Steam turbine generators bridge past and future, driving industry and grids with proven tech, evolving for sustainability.
Below is a detailed exploration of steam turbine generators, broken into distinct sections for clarity. Each section expands on the mechanics, types, applications, design specifics, historical context, and modern significance of these systems, which pair steam turbines with electric generators to convert thermal energy into electricity. This format provides a comprehensive, structured overview of steam turbine generators, used in industrial plants, utility power stations, and renewable energy systems.
What Are Steam Turbine Generators?
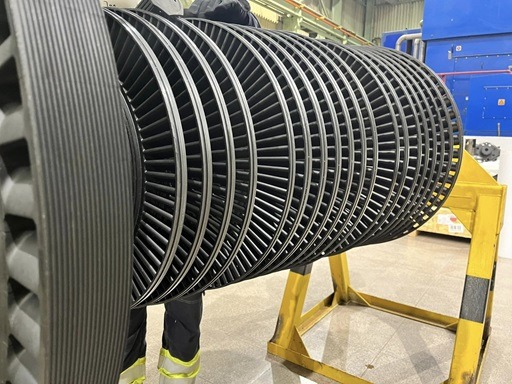
Steam turbine generators are integrated systems that transform the thermal energy of steam into electrical energy by combining a steam turbine’s mechanical power with a generator’s electrical output. They are foundational to power generation, converting heat from diverse sources—coal, natural gas, nuclear reactions, biomass, or solar thermal—into electricity for industrial, utility, or off-grid use. The steam turbine spins a rotor, driving a generator that produces alternating current (AC), typically synchronized with electrical grids at 50 Hz (3,000 RPM) or 60 Hz (3,600 RPM). These systems scale from small units generating a few kilowatts to massive installations exceeding 1,000 megawatts, making them versatile for factories, power plants, and sustainable energy projects.
How They Work
The operation of a steam turbine generator follows the Rankine cycle, a thermodynamic process optimized for power production. A boiler heats water using a fuel source—coal burning at 1,200°C, nuclear fission at 300°C, or biomass at 800-1,000°C—producing steam at pressures of 10-300 bar and temperatures of 200-600°C. This steam enters the turbine, flowing over blades mounted on a rotor. As it expands—say, from 100 bar to 0.05 bar—it accelerates to speeds like 500 m/s, pushing the blades and spinning the rotor at 3,000-10,000 RPM, depending on design. The rotor connects to a generator via a shaft, often with a gearbox in smaller units to adjust speed. Inside the generator, the rotor—fitted with magnetic poles—spins within a stator’s copper windings, inducing an electromagnetic field that generates AC electricity at voltages from 400 V to 13.8 kV. Steam exits into a condenser, cooling to water for reuse in condensing systems, or at usable pressures (e.g., 5-20 bar) for industrial heat in CHP setups, with condensing maximizing electrical output.
Types of Steam Turbine Generators
Steam turbine generators vary by turbine design and purpose, tailored to specific energy needs:
- Condensing Steam Turbine Generators: Exhaust steam into a vacuum (0.05-0.1 bar) via a condenser, maximizing power at 35-45% efficiency. A 500 MW coal unit might use HP (300 bar), IP (50 bar), and LP (0.05 bar) stages, with LP blades up to 2 meters long handling high-volume steam.
- Back-Pressure Steam Turbine Generators: Exhaust at 5-20 bar for industrial heat, common in CHP. A 5 MW unit in a paper mill produces 4 MW and 10 tons/hour of 10-bar steam, achieving 80% total efficiency.
- Extraction Steam Turbine Generators: Tap steam at intermediate pressures—e.g., 15 bar and 3 bar—while generating power. A 10 MW refinery unit delivers 8 MW and 20 tons/hour of steam, adjustable via valves.
- Combination Steam Turbine Generators: Blend impulse (high-pressure jets) and reaction (expansion across blades) designs. A 50 MW biomass unit optimizes steam across 10-20 stages.
- Reheat Steam Turbine Generators: Reheat steam mid-process—e.g., from 20 bar back to 540°C—adding 4-5% efficiency. A 1,000 MW coal unit uses this for fuel savings.
- Single-Stage vs. Multi-Stage: Single-stage suits small units (100 kW), multi-stage (5-50 stages) boosts efficiency in larger systems (200 MW).
Applications
Steam turbine generators serve diverse sectors:
- Utility Power Plants: Generate 100-1,750 MW for grids—coal (37% of global power), gas (25%), nuclear (10%). A 1,200 MW nuclear unit powers 1.5 million homes.
- Industrial Facilities: Produce 50 kW-50 MW for on-site power and heat. A 5 MW CHP unit in a chemical plant supplies 4 MW and steam for reactors.
- Biomass Plants: Burn wood or waste—e.g., a 10 MW unit uses 2 tons/hour of wood chips—for rural grids or factories.
- Geothermal and Solar Thermal: Convert earth or sun heat into 5-100 MW. A 50 MW solar thermal unit uses mirrors to heat steam.
- Off-Grid Systems: Provide backup or standalone power—e.g., a 50 kW unit in a remote mine runs on diesel or biomass.
Design Specifics
Design integrates turbine and generator components:
- Turbine Blades: HP blades (5-20 cm) use nickel alloys (Inconel 718) for 600°C, 300 bar, with coatings resisting creep. LP blades (up to 2 meters) use titanium for wet steam at 500 m/s tip speeds.
- Rotor: Forged steel, 1-20 tons, spins at 3,000-10,000 RPM, balanced to avoid vibration, with couplings or gearboxes (e.g., 5:1 ratio) linking to generators.
- Generator: Synchronous design—rotor poles spin in stator windings—produces AC at 50/60 Hz, 400 V to 13.8 kV, with cooling (air or hydrogen) for heat dissipation.
- Casings: Steel or alloys contain 300 bar, with labyrinth seals cutting leakage by 5-10%.
- Boilers: Produce 1-1,000 tons/hour of steam, fueled by coal, gas, biomass, or waste heat recovery.
- Cooling Systems: Water towers or air fans reject 50-60% of heat in condensing units, using 1-2% of output as waste.
Efficiency and Performance
Efficiency depends on design and steam conditions:
- Condensing Units: 35-45% electrical efficiency—e.g., a 1,000 MW unit converts 40% of fuel energy, losing 60% to exhaust and friction.
- CHP Units: 70-85% total efficiency—e.g., a 5 MW unit turns 25% into power, 50% into steam, losing 25%.
- Losses: Exhaust heat, blade turbulence, moisture (5-10% output drop unless superheated to 540°C or dried).
- Enhancements: Multi-stage (10-50 stages) adds 10-15% efficiency; supercritical steam (600°C, 300 bar) hits 47%, nearing Rankine’s 60% ideal.
Historical Development
Steam turbine generators trace to the late 19th century:
- Origins: Parsons’ 1884 turbine (7.5 kW) and De Laval’s impulse design pioneered the concept, paired with early dynamos.
- Early 20th Century: Outpaced steam engines by 1900, scaling to megawatts with steel advances.
- Mid-20th Century: Coal and nuclear units grew, with reheat cycles (1930s) and supercritical steam (1950s) lifting efficiency.
- WWII and Beyond: Powered ships and factories; 1970s energy crisis spurred industrial CHP.
Modern Significance
Steam turbine generators remain vital:
- Utility Role: Coal, gas, nuclear plants rely on them, though coal fades with emissions goals.
- Industrial Impact: CHP units save $1-3 million yearly—e.g., a 10 MW unit halves a factory’s energy costs.
- Renewables: Biomass (1-50 MW), geothermal (5-100 MW), solar thermal (10-200 MW) support green grids.
- Innovations: Ceramic blades (1,000°C), 3D-printed parts, AI controls enhance performance.
Advantages and Challenges
- Advantages: High output (1,750 MW max), 30-50 year lifespan, fuel flexibility.
- Challenges: High cost ($2-10 million for 5-50 MW), complex infrastructure (boilers, condensers), slow startups (hours vs. minutes).
Examples
- Utility: The Arabelle (1,750 MW) powers 2 million homes with 2-meter LP blades.
- Industrial: A 5 MW CHP unit in a mill burns wood, producing 4 MW and 10 tons/hour of steam.
- Off-Grid: A 50 kW biomass unit lights a village, using 20 kg/hour of wood.
Steam turbine generators bridge heat and electricity, evolving from Parsons’ 7.5 kW to today’s gigawatt giants, driving industry and grids with adaptability and innovation.
Here’s a continued exploration of steam turbine generators, organized into sections for clarity and depth. Each section builds on the previous discussion, diving deeper into their mechanics, types, applications, design specifics, historical context, modern significance, and operational details. Steam turbine generators pair a steam turbine’s mechanical power with a generator’s electrical output to convert thermal energy from steam into electricity, serving industrial plants, utility power stations, and renewable energy systems with remarkable versatility.
What Are Steam Turbine Generators?
Steam turbine generators are sophisticated systems that transform thermal energy from steam into electrical energy, integrating a steam turbine’s rotational force with a generator’s ability to produce alternating current (AC). They harness heat from sources like coal, natural gas, nuclear fission, biomass (e.g., wood or agricultural waste), or solar thermal energy, making them essential for power generation across scales—from small industrial units producing a few kilowatts to utility-scale systems exceeding 1,000 megawatts. The turbine spins a rotor, driving a generator typically synchronized with electrical grids at 50 Hz (3,000 RPM) or 60 Hz (3,600 RPM), delivering electricity for factories, cities, or remote sites. Their adaptability and efficiency have made them a backbone of modern energy infrastructure.
How They Work
Steam turbine generators operate on the Rankine cycle, a thermodynamic process tailored for efficient power production. A boiler heats water using a heat source—coal combusting at 1,200°C, nuclear reactions at 300°C, or biomass at 800-1,000°C—generating steam at pressures from 10 to 300 bar and temperatures from 200 to 600°C. This steam flows into the turbine, passing over blades mounted on a rotor. As it expands—dropping from, say, 100 bar to 0.05 bar—it accelerates to speeds like 500 meters per second, pushing the blades and spinning the rotor at 3,000-10,000 RPM, depending on design and load. The rotor connects to a generator via a shaft, often with a gearbox in smaller systems to adjust speed—e.g., reducing 10,000 RPM to 1,500 RPM for a pump. Inside the generator, the rotor’s magnetic poles spin within stator windings, inducing an electromagnetic field that produces AC electricity at voltages from 400 V (small units) to 13.8 kV (large plants). Steam exits into a condenser, cooling to water at 30-50°C for reuse in condensing systems, or at usable pressures like 5-20 bar for industrial heat in combined heat and power (CHP) setups, with condensing maximizing electrical yield.
Types of Steam Turbine Generators
Steam turbine generators vary by turbine design and application, each suited to specific needs:
- Condensing Steam Turbine Generators: Exhaust steam into a vacuum (0.05-0.1 bar) via a condenser, optimizing power output at 35-45% efficiency. A 500 MW coal unit uses multi-stage turbines—high-pressure (HP) at 300 bar, intermediate-pressure (IP) at 50 bar, low-pressure (LP) at 0.05 bar—with LP blades up to 2 meters long handling expanded steam.
- Back-Pressure Steam Turbine Generators: Exhaust at 5-20 bar for industrial heat, ideal for CHP. A 5 MW unit in a sugar mill produces 4 MW and 10 tons/hour of 10-bar steam, achieving 80% total efficiency.
- Extraction Steam Turbine Generators: Tap steam at intermediate pressures—e.g., 15 bar for heating, 3 bar for preheating—while generating power. A 10 MW refinery unit delivers 8 MW and 20 tons/hour of steam, adjustable with control valves.
- Combination Steam Turbine Generators: Blend impulse (high-pressure jets onto buckets) and reaction (expansion across blades) designs. A 50 MW biomass unit optimizes steam across 10-20 stages.
- Reheat Steam Turbine Generators: Reheat steam mid-process—e.g., from 20 bar back to 540°C—adding 4-5% efficiency. A 1,000 MW coal unit uses this for fuel economy.
- Single-Stage vs. Multi-Stage: Single-stage suits small units (100 kW, simple), multi-stage (5-50 stages) enhances efficiency in larger systems (200 MW).
Applications
Steam turbine generators serve a wide range of purposes:
- Utility Power Plants: Generate 100-1,750 MW for national grids—coal (37% of global power), gas (25% in combined-cycle), nuclear (10%). A 1,200 MW nuclear unit powers 1.5 million homes with three LP turbines.
- Industrial Facilities: Produce 50 kW-50 MW for on-site power and heat. A 5 MW CHP unit in a chemical plant supplies 4 MW and steam for distillation.
- Biomass Plants: Burn wood or waste—e.g., a 10 MW unit uses 2 tons/hour of wood chips—for rural grids or factory self-sufficiency.
- Geothermal and Solar Thermal: Convert natural or solar heat into 5-100 MW. A 50 MW geothermal unit taps underground steam, while a solar thermal unit uses mirrors.
- Off-Grid Systems: Provide standalone power—e.g., a 50 kW unit in a remote mine runs on diesel or local biomass, supporting critical loads.
Design Specifics
The design integrates turbine and generator components for seamless operation:
- Turbine Blades: HP blades (5-20 cm) use nickel alloys like Inconel 718, handling 600°C and 300 bar, with coatings (e.g., chromium carbide) resisting creep and corrosion. LP blades (up to 2 meters) use titanium, enduring wet steam at 500 m/s tip speeds.
- Rotor: Forged steel, 1-20 tons, spins at 3,000-10,000 RPM, precision-balanced to avoid vibration, with couplings or gearboxes (e.g., 5:1 ratio) linking to generators.
- Generator: Synchronous design—rotor poles spin in stator windings—produces AC at 50/60 Hz, 400 V to 13.8 kV. Cooling (air for small units, hydrogen for large) dissipates heat from 50-60% losses.
- Casings: Steel or alloys contain 300 bar, with labyrinth seals reducing leakage by 5-10%, critical for efficiency.
- Boilers: Produce 1-1,000 tons/hour of steam, fueled by coal, gas, biomass, or waste heat recovery from industrial exhausts.
- Cooling Systems: Water towers or air fans reject 50-60% of heat in condensing units, evaporating 1-2% of output as waste, with water needs of 1-2 liters/kWh.
Efficiency and Performance
Efficiency varies by design and steam conditions:
- Condensing Units: 35-45% electrical efficiency—e.g., a 1,000 MW unit converts 40% of fuel energy, losing 60% to exhaust, friction, and turbulence.
- CHP Units: 70-85% total efficiency—e.g., a 5 MW unit turns 25% into power, 50% into steam, losing 25% to waste heat.
- Losses: Exhaust heat (50-60%), blade-gap leaks (2-5%), moisture (5-10% output drop unless superheated to 540°C or dried with separators).
- Enhancements: Multi-stage (10-50 stages) adds 10-15% efficiency; supercritical steam (600°C, 300 bar) hits 47%; reheat cycles boost 4-5%.
Historical Development
Steam turbine generators evolved over a century:
- Origins: Parsons’ 1884 turbine (7.5 kW) and De Laval’s impulse design paired with dynamos, proving the concept.
- Early 20th Century: By 1900, turbines scaled to megawatts, outpacing steam engines with better steels and boilers.
- Mid-20th Century: Coal and nuclear units grew—reheat cycles (1930s), supercritical steam (1950s)—lifting efficiency from 20% to 45%.
- WWII and Beyond: Powered ships and factories; 1970s energy crisis drove industrial CHP adoption with biomass and waste heat.
Modern Significance
Steam turbine generators remain critical:
- Utility Role: Coal, gas, nuclear plants rely on them—e.g., a 1,000 MW gas unit supports baseload, though coal declines with emissions rules.
- Industrial Impact: CHP units save costs—a 10 MW unit cuts a factory’s $2-3 million yearly energy bill by half.
- Renewables: Biomass (1-50 MW), geothermal (5-100 MW), solar thermal (10-200 MW) align with green goals—e.g., a 20 MW wood-fired unit powers a rural grid.
- Innovations: Ceramic blades (1,000°C tolerance), 3D-printed parts, AI-driven controls enhance efficiency and durability.
Advantages and Challenges
- Advantages: High output (1,750 MW max), 30-50 year lifespan, fuel flexibility (fossil, nuclear, renewable).
- Challenges: High cost ($2-10 million for 5-50 MW), complex setups (boilers, condensers), slow startups (hours vs. minutes for gas turbines), water-heavy cooling (1-2 liters/kWh).
Examples
- Utility: The Arabelle (1,750 MW) powers 2 million homes with 2-meter LP blades in nuclear plants.
- Industrial: A 5 MW CHP unit in a mill burns wood, producing 4 MW and 10 tons/hour of steam, saving $1 million yearly.
- Off-Grid: A 50 kW biomass unit in a village uses 20 kg/hour of wood, powering lights and pumps.
Steam turbine generators are a testament to engineering ingenuity, evolving from kilowatt-scale pioneers to gigawatt powerhouses, driving industry, grids, and sustainability with unmatched versatility.
Micro Steam Turbines
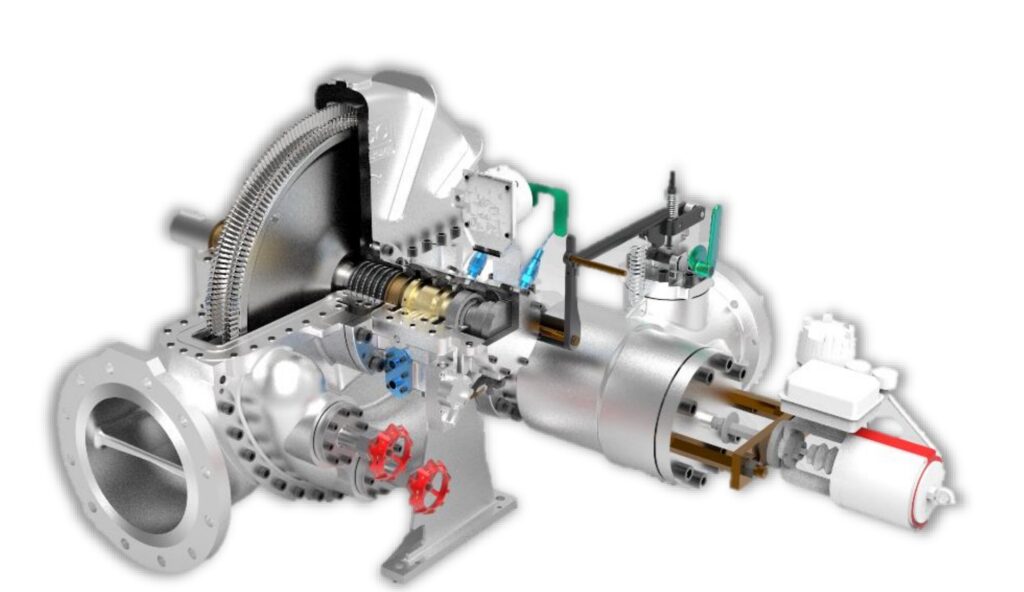
Micro steam turbines are compact, small-scale versions of traditional steam turbines, designed to generate electricity or mechanical power in applications where large turbines are impractical. Typically ranging from a few watts to about 1 megawatt (MW) in capacity, with most falling between 1 kilowatt (kW) and 500 kW, these turbines cater to niche markets such as off-grid power, small industrial operations, rural electrification, and renewable energy systems like biomass or solar thermal setups. They operate on the same fundamental principles as their larger counterparts—converting thermal energy from steam into mechanical work—but are engineered for simplicity, portability, and efficiency at lower outputs. Micro steam turbines are particularly appealing in settings where fuel sources like wood, agricultural waste, or waste heat are abundant, offering a sustainable, decentralized energy solution. Below is a detailed exploration of micro steam turbines, covering their mechanics, design, applications, advantages, challenges, and relevance in today’s energy landscape.
The operation of a micro steam turbine mirrors the Rankine cycle used in larger systems, scaled down for smaller heat inputs and power needs. A heat source—often a small boiler or heat exchanger—burns fuel like biomass (wood chips, pellets, or straw), biogas, or fossil fuels (diesel, natural gas), or captures waste heat from engines or industrial processes. This heats water to produce steam, typically at pressures of 5 to 20 bar and temperatures of 200 to 300°C, though some advanced designs reach 50 bar and 400°C for higher efficiency. The steam enters the turbine, flowing over blades attached to a rotor. As it expands—dropping from, say, 10 bar to 1 bar—it accelerates, pushing the blades and spinning the rotor at speeds ranging from 3,000 to 30,000 revolutions per minute (RPM), depending on size and design. The rotor connects to a generator via a shaft, often with a gearbox to adjust speed—for example, reducing 10,000 RPM to 3,000 RPM for a 50 Hz grid—or directly drives small machinery like pumps or fans. After expansion, the steam either exhausts to the atmosphere (in open systems), condenses into water for reuse (in closed systems with a condenser), or exits at a usable pressure (e.g., 2-5 bar) for heating in combined heat and power (CHP) setups.
The design of micro steam turbines emphasizes compactness and simplicity. Most are single-stage or dual-stage, unlike the multi-stage designs of large turbines, with a single set of blades or two sets (one fixed, one moving) to extract energy from steam. Impulse designs, inspired by Gustaf de Laval’s 19th-century turbine, are common—steam accelerates through nozzles into high-speed jets (up to 500 m/s) that strike curved, bucket-shaped blades, spinning the rotor at high speeds like 20,000-30,000 RPM. Reaction designs, based on Charles Parsons’ concept, are less frequent but used in slightly larger micro turbines (e.g., 100-500 kW), where steam expands across moving blades for gradual energy extraction, operating at lower speeds like 3,000-10,000 RPM. Blades are small—typically 2-10 centimeters long—made of stainless steel or lightweight alloys like aluminum for cost and durability, though high-end units use titanium or nickel alloys for better heat resistance. Rotors, often forged steel or aluminum, weigh a few kilograms, balanced to minimize vibration at high speeds. Casings, cast iron or steel, contain pressures up to 50 bar, with basic seals (e.g., packing glands) to reduce steam leakage, though efficiency suffers compared to the labyrinth seals of big turbines.
Micro steam turbine generators pair the turbine with a small alternator or dynamo, producing AC or DC electricity. A 10 kW unit might generate 230 V AC for household use or 12-48 V DC for battery charging, with outputs scaled to match load—e.g., 1 kW for lights, 50 kW for a workshop. Boilers are compact, producing 10-500 kg/hour of steam, fueled by wood (4.5 kWh/kg dry), biogas (5-6 MJ/m³), or waste heat (free energy). Cooling in condensing systems uses air fans or small water loops—e.g., a 5 kW unit might need 10 liters/hour of water—while CHP setups skip condensers, piping exhaust steam to radiators or dryers. Control systems are basic—manual valves or simple electronic governors—keeping costs low, though advanced units add microcontrollers for automation.
Applications of micro steam turbines are diverse and practical. In off-grid settings, a 5 kW turbine powered by wood might run a cabin’s lights, fridge, and pump, burning 2-3 kg/hour of logs, ideal for remote areas with forest access. Small industries use them for localized power—a 50 kW unit in a sawmill burns wood waste (20 kg/hour) to drive tools and dry lumber, cutting grid reliance. Biomass systems leverage them for rural electrification—e.g., a 100 kW turbine in an Indian village burns rice husks, powering 50 homes and a water pump. Waste heat recovery is a growing niche—a 20 kW turbine in a factory captures exhaust from a diesel engine (200-300°C), generating power without extra fuel. Educational labs and hobbyists use tiny units—1-10 kW—for experiments or DIY projects, often paired with solar concentrators or small stoves. Marine use exists in small boats—a 10 kW turbine might replace a diesel generator, running on biofuel.
Efficiency in micro steam turbines is lower than in large systems, typically 10-20% for electrical output, due to scale and simplicity. A 10 kW unit burning 5 kg/hour of wood (22.5 kWh thermal) might produce 2 kW (9% efficiency), losing 80% to exhaust heat, friction, and leaks. CHP boosts total efficiency to 50-70%—e.g., a 5 kW turbine generates 1 kW and 3 kW of heat via 2-bar steam, losing 30% to waste. Losses stem from small blade sizes (less energy extraction), poor sealing (5-10% steam escapes), and low steam quality—wet steam (10-20% moisture) cuts output unless superheated to 300°C. Multi-stage designs or higher pressures (20-50 bar) can hit 25-30% electrical efficiency, but complexity rises. Fuel quality matters—dry wood outperforms wet (50% moisture halves energy), and consistent heat input prevents steam fluctuations.
Historically, micro steam turbines trace to early 20th-century innovations. De Laval’s 1880s impulse turbine, spinning at 30,000 RPM, inspired small-scale designs for pumps and generators. Parsons’ multi-stage concept scaled down by the 1920s for factories, though steam engines dominated until turbines shrank further. WWII saw micro turbines in portable units—e.g., 5-10 kW systems powered field hospitals on wood gas. Post-war, interest waned with cheap oil, but the 1970s energy crisis revived them for biomass and off-grid use. Today’s designs build on this, with 3D printing and microelectronics enabling affordable, efficient units.
Advantages of micro steam turbines include their small size—e.g., a 10 kW unit fits in a 1×1-meter space—making them portable or factory-friendly. Fuel flexibility is key—they burn wood, waste, or biogas, or use waste heat, ideal where fossil fuels are scarce. Low cost appeals to niche users—a 5 kW DIY turbine might cost $1,000-$3,000 vs. $5,000 for solar. CHP capability doubles value—e.g., a 10 kW unit powers a home and heats it, slashing energy bills. Sustainability shines—biomass units are near carbon-neutral with regrowth, and waste heat recovery cuts emissions. Reliability is decent—simple designs run 5-10 years with basic upkeep, handling 1,000-5,000 hours/year.
Challenges are significant. Low efficiency (10-20%) means high fuel use—a 5 kW unit needs 2-3 kg/hour of wood vs. 0.5 liters/hour of diesel for a generator. Labor is intensive—chopping wood, feeding boilers (every 1-2 hours), and removing ash take 1-2 hours daily. Maintenance includes blade cleaning, seal replacement, and boiler descaling—$100-$500 yearly for a 10 kW unit, with downtime cutting output. Emissions—smoke, particulates (50-200 mg/m³)—need filters ($200-$500) for compliance (e.g., U.S. EPA limits at 25 mg/m³). Complexity rises with condensing or multi-stage designs, pushing costs to $5,000-$20,000 for 50-100 kW units. Scale limits competitiveness—solar or wind often beat them below 100 kW on cost ($1-2/W vs. $3-5/W).
Modern relevance ties to decentralization and renewables. Off-grid demand grows—a 10 kW turbine powers a homestead where solar fails in winter. Biomass adoption rises—e.g., a 50 kW unit in a Brazilian farm burns sugarcane waste, supporting microgrids. Waste heat recovery gains traction—a 20 kW turbine in a glass factory uses kiln exhaust, saving $10,000 yearly. Innovations help—3D-printed blades cut costs, microcontrollers boost efficiency, and ceramic coatings handle 400°C, though adoption lags behind solar’s simplicity. Examples include a 1 kW turbine on a wood stove (50 W power, 500 W heat) for cabins, or a 100 kW unit in a Thai village burning coconut shells for 80 homes.
Micro steam turbines fill a unique niche, blending old-school steam tech with modern needs. They’re not grid-scale giants but excel where small, flexible power meets abundant local fuel. As energy diversifies, their role in off-grid, biomass, and waste-heat systems keeps them relevant, a pint-sized echo of steam’s industrial legacy.
Here’s a continued exploration of micro steam turbines, presented as a long, detailed plain text that builds on the previous discussion. Micro steam turbines are small-scale power generation devices that convert thermal energy from steam into mechanical work, typically driving a generator to produce electricity or powering small machinery in applications where larger turbines are impractical. Ranging from a few watts to about 1 megawatt, with most units falling between 1 kilowatt and 500 kilowatts, they serve niche roles in off-grid setups, small industries, rural communities, and renewable energy systems like biomass or waste heat recovery. Their compact size, fuel flexibility, and ability to integrate with local resources make them a compelling option for decentralized energy, despite their challenges. Let’s dive deeper into their mechanics, design variations, applications, operational details, historical context, modern relevance, advantages, and limitations.
Micro steam turbines operate on the same Rankine cycle principle as larger turbines, scaled down for lower power outputs and simpler setups. A heat source—typically a small boiler or heat exchanger—heats water to produce steam, using fuels like biomass (wood logs, pellets, or agricultural waste such as rice husks), biogas from anaerobic digesters, fossil fuels like diesel or propane, or waste heat from engines, kilns, or industrial exhausts. Steam conditions are modest compared to utility turbines—pressures range from 5 to 20 bar, with temperatures of 200 to 300°C, though some advanced micro turbines push to 50 bar and 400°C to improve efficiency. This steam enters the turbine, flowing over blades attached to a rotor, a lightweight steel or aluminum shaft typically weighing a few kilograms. As the steam expands—dropping from, say, 10 bar to 1 bar or even atmospheric pressure—it accelerates to speeds of 300-500 meters per second, striking or flowing past the blades to spin the rotor at high speeds, often between 3,000 and 30,000 RPM, depending on the turbine’s size and design. The rotor connects to a generator—either directly or through a gearbox that adjusts speed, such as reducing 20,000 RPM to 3,000 RPM for a 50 Hz grid—to produce electricity, or it drives small mechanical loads like pumps, fans, or mills. After expansion, the steam exhausts in one of three ways: to the atmosphere in open systems (simplest but least efficient), into a condenser where it cools back to water at 30-50°C for reuse in closed loops, or at a usable pressure like 2-5 bar for heating in combined heat and power (CHP) configurations, a common feature in micro systems aiming to maximize energy use.
The design of micro steam turbines prioritizes compactness, affordability, and ease of use over the complexity of large-scale turbines. Most are single-stage, featuring one set of blades, or dual-stage, with a fixed stator directing steam onto a moving rotor, unlike the dozens of stages in utility turbines. Impulse designs dominate smaller units—steam accelerates through nozzles into high-velocity jets that hit curved, bucket-shaped blades, spinning the rotor at speeds like 20,000-30,000 RPM, a concept rooted in Gustaf de Laval’s 1880s turbine. Reaction designs, inspired by Charles Parsons’ multi-stage approach, appear in larger micro turbines (100-500 kW), where steam expands across moving blades, extracting energy gradually at lower speeds like 3,000-10,000 RPM for better generator compatibility. Blades are tiny—2 to 10 centimeters long—crafted from stainless steel or aluminum for cost and corrosion resistance, though high-performance units use titanium for lightweight strength or nickel alloys for heat tolerance up to 400°C. Rotors, often just a few kilograms, are precision-balanced to handle high speeds without vibration, a critical factor given their small scale. Casings, made of cast iron or low-grade steel, contain pressures up to 50 bar, with basic seals like packing glands or simple labyrinths to minimize steam leakage, though these are less effective than the advanced seals in big turbines, leading to 5-10% efficiency losses.
Micro steam turbine generators pair the turbine with a small alternator or dynamo to produce electricity, tailored to the application. A 5 kW unit might generate 230 V AC for household appliances or 12-48 V DC for battery charging, while a 50 kW unit could produce 400 V AC for a small factory. Generators are often synchronous, with a rotor spinning inside stator windings to produce AC at 50 or 60 Hz, cooled by air to manage heat from 50-60% energy losses. Boilers are compact, producing 10 to 500 kg/hour of steam—e.g., a 10 kW unit needs 20-30 kg/hour—fueled by wood (4.5 kWh/kg dry), biogas (5-6 MJ/m³), or waste heat (200-400°C exhaust). Condensing systems use air fans or small water loops—a 5 kW unit might need 10-20 liters/hour of cooling water—while CHP setups pipe exhaust steam to radiators, dryers, or water heaters, skipping the condenser for simplicity. Controls are basic—manual valves or mechanical governors adjust steam flow—though modern units add microcontrollers or sensors for automated startups and load matching, keeping costs between $1,000 and $20,000 depending on size and features.
Applications for micro steam turbines are practical and varied, excelling where small-scale, localized power is needed. In off-grid homes or cabins, a 5 kW turbine burns 2-3 kg/hour of wood to power lights, a fridge, and a well pump, perfect for forested areas where solar falters in winter—e.g., a Canadian homestead might use local birch, adding heat for a greenhouse. Small industries adopt them for self-sufficiency—a 50 kW turbine in a sawmill burns 20 kg/hour of sawdust, driving tools and drying lumber, saving $10,000-$20,000 yearly on grid power. Biomass-driven rural electrification uses them widely—a 100 kW turbine in an Indonesian village burns coconut shells, powering 50-80 homes and a rice mill, supporting microgrids where grid extension costs millions. Waste heat recovery is a key niche—a 20 kW turbine in a bakery captures oven exhaust (300°C), generating power without extra fuel, cutting costs by $5,000-$10,000 annually. Educational settings and hobbyists favor tiny units—1-10 kW—for experiments or prototypes, often paired with solar concentrators (mirrors heating a boiler) or wood stoves. Small boats use them too—a 10 kW turbine might replace a diesel generator, running on biofuel for quiet, eco-friendly power.
Efficiency in micro steam turbines is modest, typically 10-20% for electrical output, far below the 35-45% of large turbines, due to their small size and simplified design. A 10 kW unit burning 5 kg/hour of wood (22.5 kWh thermal input) might produce 2 kW of electricity (9% efficiency), losing 80% to exhaust heat, friction, and steam leaks. CHP configurations improve this to 50-70% total efficiency—e.g., a 5 kW turbine generates 1 kW of power and 3 kW of heat via 2-bar steam, losing 30% to waste. Losses come from small blades (less surface for energy transfer), basic seals (5-10% steam escapes), and wet steam (10-20% moisture unless superheated to 300°C), which erodes blades and cuts output. Higher pressures (20-50 bar) or dual-stage designs can reach 25-30% electrical efficiency, but added cost and complexity often outweigh gains. Fuel quality is critical—dry wood (10% moisture) yields 4.5 kWh/kg, while wet wood (50% moisture) drops to 3 kWh/kg, halving steam production if not pre-dried, a common issue in humid climates.
The historical roots of micro steam turbines stretch back to the late 19th century. De Laval’s 1880s impulse turbine, spinning at 30,000 RPM, pioneered small-scale steam power for pumps and early generators, inspiring micro designs. Parsons’ 1884 multi-stage turbine scaled down by the 1920s for small factories, though reciprocating steam engines ruled until turbines shrank further. World War II boosted their use—portable 5-10 kW units powered field stations or hospitals on wood or coal when fuel was scarce. Post-war, cheap oil sidelined them, but the 1970s energy crisis revived interest, with biomass and waste heat driving micro turbine development for off-grid and industrial use. Modern designs leverage this legacy, enhanced by 3D printing for cheap blades and electronics for better control, though they remain a niche compared to solar or wind.
Advantages of micro steam turbines are compelling for their context. Their small size—a 10 kW unit fits in a 1×1-meter space—makes them portable or easy to install in tight spots, unlike solar arrays needing acres. Fuel flexibility is a strength—they burn wood, waste, or biogas, or tap waste heat, thriving where diesel is costly or unavailable—e.g., a 20 kW unit in a remote mine uses local scrub wood, saving $5,000 yearly on fuel transport. Low cost appeals to budget users—a 5 kW DIY turbine costs $1,000-$3,000 vs. $5,000-$7,000 for solar with batteries. CHP doubles their value—a 10 kW unit powers a workshop and heats it, cutting energy bills by 50-70%. Sustainability is a draw—biomass units offset CO2 with regrowth, and waste heat recovery slashes emissions, aligning with green goals. Reliability suits rugged use—simple designs run 5-10 years, 1,000-5,000 hours/year, with minimal parts to fail.
Challenges limit their reach. Low efficiency (10-20%) demands high fuel input—a 5 kW unit burns 2-3 kg/hour of wood vs. 0.5 liters/hour of diesel for a generator, tripling fuel mass. Labor is a burden—chopping wood, feeding boilers every 1-2 hours, and clearing ash take 1-2 hours daily, vs. solar’s near-zero upkeep. Maintenance—blade cleaning, seal swaps, boiler descaling—costs $100-$500 yearly for a 10 kW unit, with 1-2 days downtime cutting power. Emissions—smoke, particulates (50-200 mg/m³)—require filters ($200-$500) to meet limits like the EU’s 20 mg/m³, adding cost and complexity. Initial investment scales poorly—$5,000-$20,000 for 50-100 kW units vs. $1-2/W for solar, losing competitiveness above 10 kW. Wet fuel or inconsistent heat (e.g., variable wood moisture) disrupts steam, dropping output by 20-30% if unmanaged.
Modern relevance hinges on niche energy needs. Off-grid growth fuels demand—a 10 kW turbine powers an Alaskan cabin where solar struggles in dark winters, burning local spruce. Biomass adoption rises—a 50 kW unit in a Kenyan farm burns maize stalks, supporting a microgrid for 30 homes. Waste heat recovery expands—a 20 kW turbine in a ceramic kiln uses 300°C exhaust, saving $8,000 yearly. Innovations push progress—3D-printed blades cut costs by 20-30%, microcontrollers lift efficiency to 25%, and ceramic coatings handle 400°C, though solar’s simplicity ($1/W installed) often wins. Examples include a 1 kW turbine on a stove (50 W power, 500 W heat) for rural charging, or a 100 kW unit in a Philippine village burning palm waste for 90 homes.
Micro steam turbines are small but mighty, merging steam’s legacy with modern ingenuity. They excel where fuel is local, power needs are modest, and flexibility matters—off-grid homes, small factories, or waste-heat sites. As energy decentralizes, their role grows, a compact testament to steam’s enduring utility.
Here’s another detailed expansion on micro steam turbines, presented as a long plain text that builds on the prior discussions while offering fresh depth. Micro steam turbines are small-scale devices that convert thermal energy from steam into mechanical work, typically driving a generator for electricity or powering machinery in applications where larger turbines are impractical or oversized. With power outputs ranging from a few watts to about 1 megawatt, though most commonly between 1 kilowatt and 500 kilowatts, they cater to specialized needs like off-grid power, small industrial processes, rural electrification, and renewable energy systems using biomass, biogas, or waste heat. These compact turbines balance simplicity and functionality, making them a viable option for decentralized energy in resource-rich settings. Let’s explore their mechanics, design nuances, applications, operational intricacies, historical evolution, modern significance, advantages, and challenges in greater detail.
The mechanics of a micro steam turbine follow the Rankine cycle, scaled down for modest power demands. A heat source—often a small boiler, stove, or heat exchanger—generates steam by burning fuels such as biomass (wood logs, sawdust, crop residues like corn stalks), biogas from manure or organic waste, fossil fuels like kerosene or natural gas, or by capturing waste heat from engines, ovens, or industrial exhausts at 200-400°C. Steam is produced at pressures of 5 to 20 bar and temperatures of 200 to 300°C in basic setups, though advanced micro turbines push to 50 bar and 400°C for better performance. This steam enters the turbine, flowing over blades fixed to a rotor—a lightweight shaft made of steel or aluminum, typically weighing 1-5 kilograms. As the steam expands—say, from 15 bar to 2 bar—it accelerates to 300-500 meters per second, striking or passing through the blades to spin the rotor at speeds between 3,000 and 30,000 RPM, depending on the turbine’s size and configuration. The rotor drives a generator through a direct shaft connection or a gearbox—for instance, stepping down 15,000 RPM to 3,000 RPM for a 50 Hz grid—or powers small mechanical loads like water pumps or grain mills. Post-expansion, steam exits either to the atmosphere in open-cycle systems (simplest but wasteful), into a condenser where it cools to water at 30-50°C for reuse in closed cycles, or at a usable pressure like 2-5 bar for heating in combined heat and power (CHP) setups, a feature that enhances their utility in small-scale applications.
Design-wise, micro steam turbines prioritize portability and cost over the elaborate staging of large turbines. Most are single-stage, with one set of blades, or dual-stage, pairing a fixed stator with a moving rotor, contrasting with the 10-50 stages of utility turbines. Impulse designs are prevalent, especially in smaller units—steam accelerates through nozzles into high-speed jets that hit curved, bucket-like blades, spinning the rotor at 20,000-30,000 RPM, echoing Gustaf de Laval’s high-speed turbine from the 1880s. Reaction designs appear in larger micro turbines (100-500 kW), where steam expands across moving blades, extracting energy more gradually at speeds like 3,000-10,000 RPM, inspired by Charles Parsons’ multi-stage approach. Blades are compact—2 to 10 centimeters long—made of stainless steel or aluminum for affordability and resistance to corrosion, though premium units use titanium for strength or nickel alloys for heat tolerance up to 400°C, especially in high-pressure models. Rotors, weighing a few kilograms, are carefully balanced to handle high speeds, minimizing vibration that could wear bearings or misalign shafts. Casings, typically cast iron or mild steel, withstand pressures up to 50 bar, using simple seals like packing glands or basic labyrinths to curb steam leakage, though these allow 5-10% loss, a trade-off for lower cost compared to the precision seals of industrial turbines.
Micro steam turbine generators couple the turbine with a small alternator or dynamo to produce electricity, sized for the task. A 5 kW unit might deliver 230 V AC for home use or 12-48 V DC for battery banks, while a 50 kW unit generates 400 V AC for a workshop. Generators are usually synchronous—rotor poles spin within stator windings to produce AC at 50 or 60 Hz—cooled by air to manage heat from 50-60% energy losses, with outputs adjustable via voltage regulators. Boilers are small, producing 10 to 500 kg/hour of steam—a 10 kW unit needs 20-30 kg/hour—fueled by wood (4.5 kWh/kg dry), biogas (5-6 MJ/m³), or waste heat (free if consistent). Condensing systems rely on air fans or minimal water loops—a 5 kW unit might use 10-20 liters/hour of water—while CHP setups pipe exhaust steam to heat sinks like radiators or dryers, avoiding condensers for simplicity. Controls range from manual valves and mechanical governors in basic models to microcontrollers or sensors in modern units, enabling automated operation and load tracking, with costs spanning $1,000 for a 1 kW DIY kit to $20,000 for a 100 kW commercial system.
Applications highlight their versatility in small-scale settings. Off-grid homes use them for reliable power—a 5 kW turbine burns 2-3 kg/hour of wood to run lights, a fridge, and a pump, thriving in forested regions like the Pacific Northwest where solar struggles in cloudy months. Small industries benefit from localized energy—a 50 kW turbine in a rice mill burns 20 kg/hour of husks, powering grinders and drying grain, saving $15,000-$25,000 yearly on grid costs. Biomass-driven rural electrification is a growing use—a 100 kW turbine in a Peruvian village burns sugarcane bagasse, supplying 60-100 homes and a school, supporting microgrids where grid lines cost $50,000 per kilometer. Waste heat recovery shines in efficiency—a 20 kW turbine in a brewery uses 300°C oven exhaust, generating power without fuel, cutting bills by $7,000-$12,000 annually. Hobbyists and educators use tiny units—1-10 kW—for projects or labs, pairing them with solar dishes or wood stoves, while small boats adopt 5-10 kW turbines on biofuel, replacing noisy diesel generators with quieter steam power.
Efficiency in micro steam turbines lags behind larger systems, typically 10-20% for electrical output, reflecting their small scale and simplified construction. A 10 kW unit burning 5 kg/hour of wood (22.5 kWh thermal) might produce 2 kW (9% efficiency), losing 80% to exhaust heat, friction, and leaks, compared to 35-45% in utility turbines. CHP boosts total efficiency to 50-70%—a 5 kW turbine generates 1 kW of power and 3 kW of heat via 2-bar steam, losing 30% to waste. Losses arise from small blades (less energy capture), basic seals (5-10% steam escapes), and wet steam (10-20% moisture unless superheated to 300°C), which erodes blades and reduces output. Higher pressures (20-50 bar) or dual-stage designs can reach 25-30% electrical efficiency, but costs jump from $2,000 to $10,000 for a 10 kW unit. Fuel quality impacts performance—dry wood (10% moisture) delivers 4.5 kWh/kg, while wet wood (50% moisture) falls to 3 kWh/kg, cutting steam by 30-50% if not dried, a frequent hurdle in damp climates.
Historically, micro steam turbines emerged from late 19th-century innovations. De Laval’s 1880s impulse turbine, with its 30,000 RPM speed, set the stage for small-scale steam power, initially driving pumps and early dynamos. Parsons’ 1884 multi-stage design scaled down by the 1920s for small factories, though steam engines held sway until turbines miniaturized further. World War II saw them in portable 5-10 kW units—e.g., powering remote bases on coal or wood when fuel was tight. Post-war, cheap oil dimmed their appeal, but the 1970s oil crisis sparked a revival, with biomass and waste heat driving micro turbine use in off-grid and industrial niches. Today’s designs refine this heritage—3D printing slashes blade costs by 20-30%, and electronics improve control, though they remain a specialty tool.
Advantages make them attractive in context. Their small footprint—a 10 kW unit fits in a 1×1-meter space—suits tight installations or portability, unlike solar needing large panels. Fuel flexibility excels—they burn wood, waste, or biogas, or use waste heat, perfect where diesel costs $1-$2/liter or grids fail—e.g., a 20 kW unit in an African village uses crop waste, avoiding $6,000 yearly fuel imports. Low cost draws users—a 5 kW turbine at $1,000-$3,000 undercuts $5,000-$8,000 solar-plus-battery setups. CHP enhances value—a 10 kW unit powers a farm and heats a barn, saving 60-80% on energy costs. Sustainability aligns with green trends—biomass units offset CO2 with regrowth, and waste heat recovery cuts fuel use, appealing to eco-conscious users. Reliability fits tough conditions—simple designs run 5-10 years, 1,000-5,000 hours/year, with few moving parts to break.
Challenges temper their appeal. Low efficiency (10-20%) means high fuel demand—a 5 kW unit burns 2-3 kg/hour of wood vs. 0.5 liters/hour of diesel, a 5-6x mass penalty. Labor is demanding—chopping fuel, feeding boilers every 1-2 hours, and clearing ash take 1-2 hours daily, vs. wind’s near-zero effort. Maintenance—blade cleaning, seal swaps, boiler descaling—costs $100-$500 yearly for a 10 kW unit, with downtime disrupting power. Emissions—smoke, particulates (50-200 mg/m³)—need filters ($200-$500) to meet limits like the EU’s 20 mg/m³, raising complexity. Costs scale poorly—$5,000-$20,000 for 50-100 kW units vs. $1-2/W for solar, losing ground above 10 kW. Fuel inconsistency—wet wood or uneven heat—drops output by 20-40% if unmanaged.
Modern significance reflects niche energy trends. Off-grid needs grow—a 10 kW turbine powers a Montana ranch where solar fades in snow. Biomass use expands—a 50 kW unit in a Thai farm burns palm fronds, supporting a microgrid for 40 homes. Waste heat recovery rises—a 20 kW turbine in a pottery kiln uses 300°C exhaust, saving $9,000 yearly. Innovations aid adoption—3D-printed blades cut costs, sensors lift efficiency to 25%, and coatings handle 400°C, though solar’s $1/W simplicity often prevails. Examples include a 1 kW turbine on a stove (50 W power, 500 W heat) for rural cabins, or a 100 kW unit in a Colombian village burning coffee husks for 70 homes.
Micro steam turbines are small-scale powerhouses, merging steam’s heritage with practical ingenuity. They thrive where fuel is local, power is modest, and flexibility counts—off-grid sites, biomass-rich areas, or waste-heat zones—offering a tailored energy fix as the world diversifies beyond centralized grids.
Non Condensing Steam Turbine
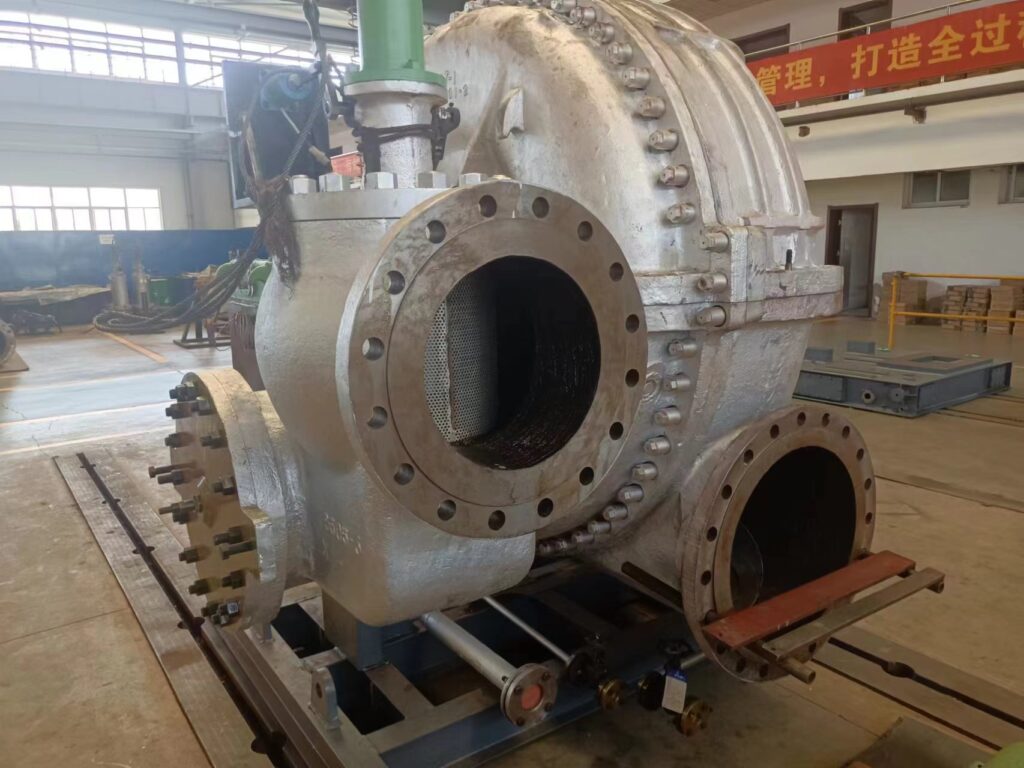
Here’s a detailed exploration of non-condensing steam turbines, presented as a long plain text. Non-condensing steam turbines, also known as back-pressure turbines, are a type of steam turbine where the steam exits at a pressure higher than atmospheric, rather than being condensed into a vacuum as in condensing turbines. These turbines are designed to produce mechanical work—typically to generate electricity or drive machinery—while also supplying usable exhaust steam for industrial processes, heating, or other thermal applications. They are widely used in settings where both power and heat are needed, such as industrial plants, small power stations, and combined heat and power (CHP) systems, with power outputs ranging from a few kilowatts to tens of megawatts. Non-condensing steam turbines are valued for their simplicity, efficiency in CHP applications, and ability to leverage steam as a dual-purpose resource. Let’s dive into their mechanics, design features, applications, operational details, historical context, modern relevance, advantages, and challenges.
The operation of a non-condensing steam turbine follows the Rankine cycle, adapted to prioritize both mechanical power and usable exhaust steam over maximum electrical efficiency. A boiler generates steam by burning fuels like coal, natural gas, oil, biomass (e.g., wood chips, bagasse), or capturing waste heat from industrial processes—say, furnace exhaust at 400-600°C. Steam enters the turbine at pressures typically between 10 and 100 bar and temperatures of 200-540°C, depending on the system’s scale and fuel source. This steam flows over blades attached to a rotor—a steel shaft weighing from a few kilograms in small units to tons in larger ones. As the steam expands—dropping from, say, 50 bar to 5-20 bar—it accelerates to speeds of 300-500 meters per second, pushing the blades and spinning the rotor at speeds like 3,000 RPM for 50 Hz grids, 3,600 RPM for 60 Hz, or up to 10,000 RPM in high-speed designs. The rotor drives a generator to produce electricity or powers machinery like pumps, compressors, or fans via a shaft, often with a gearbox to match equipment speeds. Unlike condensing turbines, the steam does not exhaust into a vacuum (0.05-0.1 bar); instead, it exits at a higher pressure—typically 2-20 bar—determined by downstream needs, such as heating a building, drying materials, or feeding a process like chemical distillation. This exhaust steam retains significant thermal energy, making non-condensing turbines ideal for CHP setups where total energy efficiency is the goal.
The design of non-condensing steam turbines reflects their dual-purpose role and simpler construction compared to condensing systems. They can be single-stage for small applications—say, a 50 kW unit with one blade set—or multi-stage with 5-20 stages for larger outputs like 10 MW, extracting energy gradually across multiple blade rows. Impulse designs are common, especially in smaller units—steam accelerates through nozzles into high-velocity jets that strike curved, bucket-shaped blades, spinning the rotor at high speeds like 10,000-20,000 RPM, a nod to Gustaf de Laval’s 1880s turbine. Reaction designs, inspired by Charles Parsons’ multi-stage concept, dominate in larger non-condensing turbines—steam expands across fixed stator and moving rotor blades, spinning at 3,000-6,000 RPM for efficiency and generator compatibility. Combination designs blend impulse and reaction stages—impulse for high-pressure entry (e.g., 50 bar), reaction for lower-pressure exhaust (e.g., 10 bar)—optimizing energy extraction in mid-sized systems. Blades are sized for the pressure range—5-20 cm in high-pressure sections, up to 50 cm in low-pressure stages—made of stainless steel or nickel alloys (e.g., Inconel) for heat resistance up to 540°C, with coatings like chromium to combat corrosion. Rotors, forged steel, are balanced to handle high speeds, while casings—cast steel or alloys—contain pressures up to 100 bar, with seals like labyrinths or packing glands minimizing leakage, though less critical than in condensing turbines since exhaust pressure is higher.
Non-condensing steam turbine generators pair the turbine with an alternator or dynamo to produce electricity, tailored to the system’s scale. A 1 MW unit might generate 400 V AC for industrial use, while a 50 kW unit produces 230 V AC for a small facility, synchronized at 50 or 60 Hz. Generators are synchronous—rotor poles spin within stator windings—cooled by air or water to manage heat from 50-70% energy losses, with voltage adjustable via regulators. Boilers, producing 10-100 tons/hour of steam, integrate with fuels like natural gas (clean, efficient), biomass (sustainable), or waste heat (cost-free). Unlike condensing systems, non-condensing turbines skip the condenser and cooling towers, reducing complexity—exhaust steam pipes directly to process equipment, radiators, or heat exchangers. Controls vary—manual valves in basic setups, digital systems with sensors in modern units—adjusting steam flow to balance power and heat output, with costs from $10,000 for a 50 kW unit to $1 million for a 10 MW system.
Applications of non-condensing steam turbines center on industries needing both power and heat. Pulp and paper mills use them extensively—a 5 MW turbine burns wood waste, producing 4 MW of electricity and 10 tons/hour of 5-bar steam to dry pulp, leveraging abundant biomass. Chemical plants deploy them for process steam—a 10 MW unit generates 8 MW and 20 tons/hour of 15-bar steam for reactors or distillation, often fueled by natural gas. Sugar mills rely on them with bagasse—a 3 MW turbine powers milling equipment and supplies 8 tons/hour of 10-bar steam for juice evaporation, using cane waste. Textile factories use smaller units—a 500 kW turbine produces 400 kW and 2 tons/hour of 3-bar steam for dyeing or heating, often coal-fired. District heating systems adopt them in cold regions—a 2 MW turbine generates 1.5 MW and heats a small town with 5-bar steam, burning local biomass. Small power plants or cogeneration units use them for grid support—a 20 MW turbine supplies 15 MW and steam for nearby factories, fueled by oil or gas. Off-grid setups benefit too—a 50 kW turbine in a remote farm burns wood, powering tools and heating barns.
Efficiency in non-condensing steam turbines varies by focus. Electrical efficiency is lower than condensing turbines—typically 20-30%—since less steam energy converts to work. A 5 MW unit burning 20 tons/hour of steam (50 bar, 540°C) might produce 1.5 MW (25% efficiency), with 70% of energy exiting as usable 10-bar steam, losing 5-10% to friction and leaks. In CHP mode, total efficiency—power plus heat—reaches 70-85%, far exceeding condensing systems’ 35-45%. For example, a 3 MW turbine converts 25% to electricity (750 kW), 55% to 5-bar steam (1.65 MW thermal), losing 20% to waste. Losses stem from incomplete expansion (exhaust at 5-20 bar vs. 0.05 bar), blade turbulence, and leakage (5-10% with basic seals). Multi-stage designs or superheating to 540°C boost electrical efficiency to 30-35%, but exhaust pressure limits gains—lower exhaust (e.g., 2 bar) increases power but reduces usable heat. Fuel quality affects output—dry biomass (4.5 kWh/kg) outperforms wet (3 kWh/kg), with moisture above 20% cutting steam by 30%.
Historically, non-condensing steam turbines evolved from early steam power needs. Parsons’ 1884 multi-stage turbine and De Laval’s impulse design laid the groundwork, but by the early 20th century, industries adopted them for dual power-and-heat roles, replacing steam engines (5-10% efficiency) with turbines (15-25%). Pre-WWII factories used coal-fired units for machinery and heating, scaling to megawatts by the 1930s. WWII saw them in small plants or ships—e.g., a 1 MW unit powered a factory and warmed it on limited fuel. Post-war, condensing turbines dominated large grids, but the 1970s energy crisis revived non-condensing designs for CHP, especially with biomass and waste heat. Modern units refine this—automation and materials like nickel alloys enhance performance, though they remain niche vs. condensing giants.
Modern relevance ties to energy efficiency and sustainability. Industrial CHP thrives—a 10 MW turbine saves a factory $2-3 million yearly by reusing steam, vs. separate power and heat systems costing 50% more. Biomass use grows—a 5 MW unit burns 2 tons/hour of wood waste, cutting fossil fuel reliance in rural mills. Waste heat recovery expands—a 2 MW turbine uses 400°C exhaust from a steel furnace, generating power and steam emissions-free. District heating in Europe leans on them—a 3 MW turbine heats 500 homes and powers local loads, fueled by gas or biomass. Decentralized power benefits—off-grid sites use 50-500 kW units where grids falter. Innovations help—digital controls optimize steam use, coatings handle 540°C, and modular designs cut costs, though condensing turbines lead in raw power output.
Advantages of non-condensing steam turbines include simplicity—no condenser or cooling towers slashes setup costs ($500-$1,000/kW vs. $1,500-$2,000/kW for condensing). High CHP efficiency (70-85%) maximizes energy use—a 5 MW unit delivers 4 MW and 10 tons/hour of steam, beating standalone generators (30-40%). Fuel flexibility suits local resources—wood, waste, or gas work seamlessly. Compactness fits tight spaces—a 1 MW unit needs 5×10 meters vs. 10×20 for condensing. Reliability endures—20-30 years with maintenance, running 5,000-8,000 hours/year. Sustainability aligns with green goals—biomass units offset CO2, and waste heat cuts fuel bills.
Challenges limit their scope. Lower electrical efficiency (20-30%) caps power output—a 5 MW unit yields 1.5 MW vs. 2 MW from a condensing turbine, needing more fuel (e.g., 2 tons/hour of wood vs. 1.5 tons). Exhaust pressure trade-offs constrain design—5-20 bar balances heat and power but sacrifices electrical gains. Maintenance—blade wear, seal leaks, boiler scaling—costs $10,000-$50,000 yearly for a 5 MW unit, with downtime cutting production. Emissions from biomass (50-200 mg/m³ particulates) need filters ($10,000-$50,000) for compliance (e.g., U.S. EPA 25 mg/m³). Initial costs—$500,000 for 1 MW—lag solar ($1-2/W) for small scales. Fuel logistics—wet biomass or inconsistent heat—drop output by 20-30%.
Examples illustrate their role. A 1 MW turbine in a textile plant burns coal, producing 800 kW and 3 tons/hour of 5-bar steam for dyeing, saving $200,000 yearly. A 10 MW unit in a paper mill uses wood waste, generating 8 MW and 20 tons/hour of 10-bar steam, cutting costs by $2 million. A 50 kW off-grid turbine burns logs, powering a farm and heating it with 2-bar steam, costing $10,000 but saving $5,000 annually.
Non-condensing steam turbines excel where power and heat intertwine, offering a practical, efficient solution for industry and small-scale energy. Their legacy endures, adapting to modern needs with a focus on resourcefulness and sustainability.
Here’s a continued exploration of non-condensing steam turbines, presented as a detailed plain text that builds on the previous discussion while offering deeper insights. Non-condensing steam turbines, often referred to as back-pressure turbines, are a specialized type of steam turbine where the exhaust steam exits at a pressure above atmospheric levels, typically between 2 and 20 bar, rather than being condensed into a vacuum as in condensing turbines. These turbines are engineered to produce mechanical work—usually driving a generator for electricity or powering machinery—while simultaneously providing usable exhaust steam for heating, industrial processes, or other thermal applications. They are particularly suited for combined heat and power (CHP) systems, small industrial plants, and settings where both electrical and thermal energy are valuable, with power outputs ranging from a few kilowatts to tens of megawatts. Non-condensing steam turbines stand out for their simplicity, high total energy efficiency in CHP configurations, and adaptability to diverse fuel sources. Let’s expand further on their mechanics, design characteristics, applications, operational specifics, historical evolution, modern significance, advantages, and challenges.
The mechanics of a non-condensing steam turbine are rooted in the Rankine cycle, tailored to balance mechanical power and thermal output rather than maximizing electrical efficiency alone. A boiler generates steam by combusting fuels such as coal, natural gas, oil, or biomass like wood chips, sawdust, or agricultural residues (e.g., rice husks, sugarcane bagasse), or by harnessing waste heat from industrial processes—imagine exhaust from a kiln at 400-600°C or a diesel engine at 300°C. Steam enters the turbine at pressures typically ranging from 10 to 100 bar and temperatures from 200 to 540°C, depending on the system’s size and fuel quality. This steam flows over blades mounted on a rotor—a forged steel shaft that can weigh a few kilograms in small units or several tons in larger ones. As the steam expands—say, from 50 bar to 10 bar—it accelerates to speeds of 300-500 meters per second, pushing the blades and spinning the rotor at speeds like 3,000 RPM for 50 Hz electrical grids, 3,600 RPM for 60 Hz, or up to 10,000 RPM in high-speed industrial designs. The rotor’s motion drives a generator to produce electricity or powers mechanical equipment such as pumps, compressors, fans, or mills through a shaft, often paired with a gearbox to adjust speed—for example, stepping down 10,000 RPM to 1,500 RPM for a pump. Unlike condensing turbines, which exhaust into a vacuum (0.05-0.1 bar) to extract maximum energy, non-condensing turbines release steam at a higher pressure—2 to 20 bar—set by the needs of downstream applications like heating a factory, drying lumber, or supplying steam for chemical reactions. This exhaust steam retains significant thermal energy, often 50-70% of the input, making these turbines ideal for CHP systems where total energy utilization is prioritized over electrical output alone.
The design of non-condensing steam turbines reflects their practical focus on dual-purpose energy production and reduced complexity compared to condensing systems. They can be single-stage for small-scale use—such as a 50 kW unit with one blade set extracting energy in a single pass—or multi-stage with 5 to 20 stages for larger capacities like 10 MW, allowing progressive energy extraction across multiple blade rows. Impulse designs are frequent in smaller units—steam accelerates through nozzles into high-velocity jets that strike curved, bucket-shaped blades, spinning the rotor at high speeds like 10,000-20,000 RPM, a design echoing Gustaf de Laval’s 1880s impulse turbine. Reaction designs, drawing from Charles Parsons’ multi-stage approach, are more common in larger non-condensing turbines—steam expands across alternating fixed stator and moving rotor blades, spinning at 3,000-6,000 RPM for better efficiency and compatibility with standard generators. Combination designs integrate impulse and reaction stages—impulse for the high-pressure entry (e.g., 50 bar) and reaction for the lower-pressure exhaust (e.g., 10 bar)—optimizing performance in mid-sized systems. Blades vary in size—5-20 cm in high-pressure sections, up to 50 cm in low-pressure stages—crafted from stainless steel for durability or nickel-based alloys like Inconel for heat resistance up to 540°C, often coated with chromium or ceramic to resist corrosion and erosion from wet steam. Rotors, made of forged steel, are precision-balanced to handle high speeds without vibration, while casings—cast steel or alloys—contain pressures up to 100 bar, using seals like labyrinths or packing glands to minimize leakage, though sealing is less critical here since exhaust pressure exceeds atmospheric levels, reducing the pressure differential that drives leaks.
Non-condensing steam turbine generators couple the turbine with an alternator or dynamo to produce electricity, scaled to the system’s needs. A 1 MW unit might generate 400 V AC for industrial machinery, while a 50 kW unit produces 230 V AC for a small facility, synchronized at 50 or 60 Hz for grid or local use. Generators are typically synchronous—rotor poles spin within stator windings—cooled by air or water to dissipate heat from 50-70% energy losses, with voltage adjustable via regulators to match loads. Boilers, producing 10 to 100 tons/hour of steam, integrate with diverse fuels—natural gas offers clean combustion, biomass leverages local waste, and waste heat taps free energy from processes like steelmaking or cement production. Unlike condensing turbines, non-condensing systems eliminate the need for a condenser and associated cooling infrastructure—exhaust steam is piped directly to heat exchangers, process equipment, or heating systems, simplifying installation and maintenance. Control systems range from manual valves and mechanical governors in basic setups to digital controls with sensors in advanced units, adjusting steam flow to optimize the balance between power and heat output, with costs ranging from $10,000 for a 50 kW unit to $1-2 million for a 10 MW system, depending on features and capacity.
Applications of non-condensing steam turbines are centered in industries and settings where both electrical power and thermal energy are in demand. Pulp and paper mills are a prime example—a 5 MW turbine burns wood waste from sawmill operations, producing 4 MW of electricity to run machinery and 10 tons/hour of 5-bar steam to dry paper pulp, capitalizing on abundant biomass to offset grid costs by $1-2 million annually. Chemical plants use them for process steam—a 10 MW turbine generates 8 MW of power and 20 tons/hour of 15-bar steam for reactors, distillation columns, or heating, often fueled by natural gas for consistent output. Sugar mills rely heavily on them with bagasse—a 3 MW turbine powers milling equipment and delivers 8 tons/hour of 10-bar steam to evaporate cane juice, using cane waste to achieve near-self-sufficiency during harvest season. Textile factories employ smaller units—a 500 kW turbine produces 400 kW and 2 tons/hour of 3-bar steam for dyeing fabrics or heating dye vats, often coal-fired in regions with cheap coal access. District heating systems in colder climates adopt them—a 2 MW turbine generates 1.5 MW of electricity and heats a small town with 5-bar steam piped to radiators, burning local biomass like wood pellets for sustainability. Small cogeneration plants use them for grid support—a 20 MW turbine supplies 15 MW to the grid and steam to nearby factories, fueled by oil or gas in urban areas. Off-grid applications benefit as well—a 50 kW turbine in a remote farm burns wood, powering tools and heating livestock barns with 2-bar steam, providing energy independence where grid extension is impractical.
Efficiency in non-condensing steam turbines depends on their operational goals. Electrical efficiency is typically lower than that of condensing turbines—ranging from 20-30%—because the steam expansion stops at a higher exhaust pressure, leaving much of its energy as usable heat. A 5 MW turbine burning 20 tons/hour of steam (50 bar, 540°C) might produce 1.5 MW of electricity (25% efficiency), with 70% of the energy exiting as 10-bar steam (3.5 MW thermal equivalent), and 5-10% lost to friction, turbulence, and leakage. In CHP mode, total efficiency—combining power and heat—reaches 70-85%, far surpassing the 35-45% electrical efficiency of condensing systems. For instance, a 3 MW turbine might convert 25% to electricity (750 kW), 55% to 5-bar steam (1.65 MW thermal), and lose 20% to waste heat. Losses come from incomplete expansion (exhaust at 5-20 bar vs. 0.05 bar in condensing units), blade inefficiencies (less staging than large turbines), and leakage (5-10% with basic seals). Multi-stage designs with 5-20 stages or superheating steam to 540°C can boost electrical efficiency to 30-35%, but lowering exhaust pressure (e.g., to 2 bar) increases power at the expense of usable heat, a trade-off that CHP systems carefully balance. Fuel quality impacts performance—dry biomass at 4.5 kWh/kg outperforms wet biomass at 3 kWh/kg, with moisture levels above 20% reducing steam output by 30% if not pre-dried, a common challenge in biomass-heavy applications.
The historical development of non-condensing steam turbines traces back to the early days of steam power. Charles Parsons’ 1884 multi-stage turbine and Gustaf de Laval’s impulse design provided the foundation, but by the early 20th century, industries adopted non-condensing turbines for their ability to supply both power and heat, outpacing less efficient steam engines (5-10% efficiency) with turbines offering 15-25%. Pre-World War II factories used coal-fired units to drive machinery and heat workspaces, scaling to megawatts by the 1930s as metallurgy improved—chromium steels allowed higher temperatures and pressures. During WWII, non-condensing turbines powered small plants or military facilities—e.g., a 1 MW unit ran a factory and warmed it on rationed fuel, proving their resilience. Post-war, condensing turbines took over large-scale power generation, but the 1970s energy crisis revived non-condensing designs for CHP, particularly with biomass and waste heat as oil prices soared. Modern units enhance this legacy—digital automation optimizes steam use, and materials like nickel alloys withstand 540°C, though they remain a specialized tool compared to condensing systems.
The modern significance of non-condensing steam turbines lies in their efficiency and adaptability in energy-conscious applications. Industrial CHP systems benefit greatly—a 10 MW turbine saves a factory $2-3 million annually by reusing steam for processes, far more cost-effective than separate power generation (30-40% efficiency) and heating systems (50-60% fuel loss). Biomass utilization is on the rise—a 5 MW turbine burns 2 tons/hour of wood waste, reducing fossil fuel dependence in rural industries like sawmills or paper plants, aligning with carbon reduction goals. Waste heat recovery expands their reach—a 2 MW turbine uses 400°C exhaust from a glass furnace, generating power and steam without additional fuel, cutting emissions and costs by $500,000 yearly. District heating systems in Europe and North America rely on them—a 3 MW turbine heats 500 homes and powers local loads with 5-bar steam, often fueled by gas or biomass for reliability and sustainability. Decentralized power applications grow too—a 50-500 kW turbine serves off-grid communities or farms where grid access costs exceed $10,000 per kilometer. Innovations bolster their role—digital controls with real-time sensors balance power and heat, advanced coatings handle higher temperatures, and modular designs reduce installation costs, though condensing turbines dominate in pure power generation.
The advantages of non-condensing steam turbines are numerous and context-specific. Their simplicity—no need for a condenser or cooling towers—reduces initial costs to $500-$1,000 per kW, compared to $1,500-$2,000/kW for condensing systems, and cuts maintenance by eliminating complex cooling infrastructure. High CHP efficiency (70-85%) maximizes energy use—a 5 MW turbine delivers 4 MW of electricity and 10 tons/hour of usable steam, outperforming standalone generators or boilers in total energy output. Fuel flexibility accommodates local resources—wood, agricultural waste, gas, or waste heat work effectively, making them viable in diverse settings like forested regions or industrial zones. Their compact size fits constrained spaces—a 1 MW unit requires a 5×10-meter footprint versus 10×20 meters for a condensing setup, easing installation in factories or small plants. Reliability is strong—units run 20-30 years with regular maintenance, operating 5,000-8,000 hours/year in harsh conditions like dusty mills or humid climates. Sustainability appeals to green initiatives—biomass-driven units achieve near carbon neutrality with regrowth, and waste heat recovery minimizes fuel use, supporting eco-friendly operations.
Challenges, however, temper their broader adoption. Their lower electrical efficiency (20-30%) limits power output—a 5 MW turbine yields 1.5 MW of electricity compared to 2 MW from a condensing turbine with the same input, requiring more fuel (e.g., 2 tons/hour of wood versus 1.5 tons) for equivalent power. The trade-off between exhaust pressure and power constrains design—higher exhaust pressures (10-20 bar) preserve heat but reduce electrical yield, while lower pressures (2-5 bar) boost power at the cost of thermal utility, a delicate balance for CHP optimization. Maintenance needs—blade wear from wet steam, seal leakage, and boiler scaling—cost $10,000-$50,000 annually for a 5 MW unit, with downtime interrupting power or heat supply, a concern in continuous operations. Emissions from biomass fuels—particulates at 50-200 mg/m³ and CO—require filters or scrubbers costing $10,000-$50,000 to meet regulations like the U.S. EPA’s 25 mg/m³ limit, adding expense and complexity. Initial costs, while lower than condensing systems, remain significant—$500,000 for a 1 MW unit can’t compete with solar’s $1-2/W for small-scale power. Fuel logistics pose risks—wet biomass (e.g., 50% moisture) or inconsistent waste heat reduces steam quality, cutting output by 20-30% if not managed with drying or buffering systems.
Examples showcase their practical impact. A 1 MW non-condensing turbine in a textile plant burns coal, producing 800 kW of electricity and 3 tons/hour of 5-bar steam for dyeing, saving $200,000 annually on energy bills with a $400,000 setup cost. A 10 MW turbine in a paper mill uses wood waste, generating 8 MW and 20 tons/hour of 10-bar steam for drying, reducing costs by $2 million yearly against a $2 million investment. A 50 kW off-grid turbine in a rural farm burns logs, powering tools with 40 kW and heating a barn with 2-bar steam, costing $10,000 but saving $5,000 annually over diesel generators.
Non-condensing steam turbines shine where power and heat converge, offering a streamlined, efficient solution for industries, small plants, and off-grid sites. Their evolution—from early 20th-century factories to modern CHP systems—reflects a focus on resource efficiency and adaptability, ensuring they remain relevant in a world balancing energy needs with sustainability.
Condensing Steam Turbine
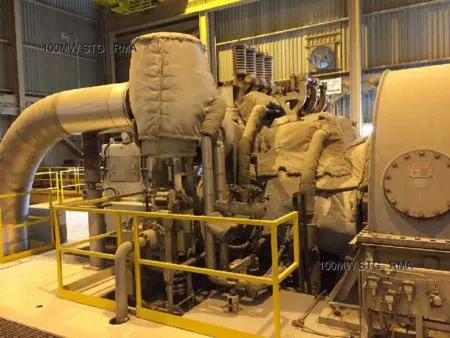
Here’s a detailed exploration of condensing steam turbines, presented as a long plain text. Condensing steam turbines are a type of steam turbine designed to maximize electrical power generation by exhausting steam into a vacuum, typically at 0.05 to 0.1 bar, rather than at a higher pressure as in non-condensing turbines. This low exhaust pressure allows the steam to expand fully, extracting the maximum possible energy to drive a rotor, which in turn powers a generator or machinery. Widely used in utility-scale power plants, large industrial facilities, and some renewable energy systems, condensing steam turbines range in capacity from a few kilowatts to over 1,000 megawatts, with a focus on achieving high electrical efficiency—typically 35-45%—at the expense of rejecting significant heat as waste. They are complex systems requiring boilers, condensers, and cooling infrastructure, making them suited for applications where electricity is the primary goal. Let’s dive into their mechanics, design features, applications, operational details, historical context, modern relevance, advantages, and challenges.
The mechanics of a condensing steam turbine are based on the Rankine cycle, optimized to convert as much thermal energy as possible into mechanical work. A boiler generates steam by burning fuels like coal, natural gas, oil, or biomass (e.g., wood chips, agricultural waste), or using heat from nuclear fission or concentrated solar power, producing steam at high pressures—typically 50 to 300 bar—and temperatures of 400-600°C, depending on the system’s scale and technology. This steam enters the turbine, flowing over blades attached to a rotor—a massive forged steel shaft weighing tons in large units or kilograms in smaller ones. As the steam expands—dropping from, say, 100 bar to 0.05 bar—it accelerates to speeds of 500-700 meters per second, pushing the blades and spinning the rotor at speeds like 3,000 RPM for 50 Hz grids, 3,600 RPM for 60 Hz, or up to 10,000 RPM in smaller, high-speed designs. The rotor drives a generator to produce electricity or, less commonly, powers machinery like pumps or compressors via a shaft, often with a gearbox to adjust speed. Unlike non-condensing turbines, which exhaust at 2-20 bar for heat use, condensing turbines release steam into a condenser—a heat exchanger that cools the steam back to water at 30-50°C using cold water from rivers, oceans, or cooling towers, or air in dry regions—creating a vacuum that maximizes the pressure drop and energy extraction. This condensed water is then pumped back to the boiler, closing the cycle, while the cooling process rejects 50-60% of the input energy as waste heat.
The design of condensing steam turbines is complex and tailored for high efficiency across a wide pressure range. They are typically multi-stage, with 10 to 50 stages grouped into high-pressure (HP), intermediate-pressure (IP), and low-pressure (LP) sections, each optimized for its steam conditions. HP stages handle initial conditions—e.g., 300 bar, 600°C—with small, robust blades (5-20 cm) made of nickel-based alloys like Inconel to withstand extreme heat and pressure, often coated with chromium or ceramics to resist creep and corrosion. IP stages manage reheated steam—say, 50 bar, 540°C—while LP stages process low-pressure, high-volume steam (0.05 bar) with massive blades up to 2 meters long, made of titanium or stainless steel to resist erosion from wet steam at tip speeds of 500-700 m/s. Impulse designs dominate HP stages—steam jets from nozzles strike buckets—while reaction designs prevail in LP stages, where steam expands across moving blades, blending both in combination turbines for optimal energy extraction. Rotors, weighing 10-100 tons in large units, are precision-balanced to spin without vibration, connected to generators via direct shafts or couplings. Casings, forged steel or alloys, contain pressures up to 300 bar, with labyrinth seals minimizing leakage across stages—critical given the huge pressure drop to vacuum. Condensers, often shell-and-tube or surface types, use 1-2 liters of cooling water per kWh, rejecting heat to maintain the vacuum, supported by cooling towers or air fans that evaporate 1-2% of output as waste.
Condensing steam turbine generators pair the turbine with a synchronous generator to produce electricity, scaled to the system’s capacity. A 500 MW unit generates 13.8 kV AC for grid transmission, while a 5 MW industrial unit produces 400 V AC, synchronized at 50 or 60 Hz. Generators feature rotor poles spinning within stator windings, cooled by hydrogen (large units) or air (smaller ones) to dissipate heat from 50-60% losses, with transformers stepping up voltage for distribution. Boilers, producing 100-1,000 tons/hour of steam, integrate with fuels like coal (high energy), gas (clean), or biomass (sustainable), or heat from nuclear reactors or solar mirrors. Cooling systems are extensive—water towers process millions of liters daily, while air-cooled condensers suit arid areas, though less efficient. Controls are sophisticated—digital systems with sensors monitor pressure, temperature, and vacuum, adjusting steam flow and cooling to optimize output, with costs from $50,000 for a 5 MW unit to $50 million for a 500 MW plant.
Applications of condensing steam turbines are primarily in power generation. Utility power plants dominate—coal-fired units (37% of global power) range from 100-1,000 MW, gas-fired combined-cycle plants (25%) hit 500-1,500 MW, and nuclear plants (10%) reach 1,000-1,750 MW, powering millions of homes—e.g., a 1,200 MW nuclear turbine serves 1.5 million households. Large industrial facilities use smaller units—a 5-50 MW turbine powers a steel mill or refinery, fueled by gas or waste heat, supplying all onsite needs. Biomass plants adopt them for renewables—a 10-50 MW unit burns 2-10 tons/hour of wood, supporting rural grids or factories. Geothermal systems use them—a 5-100 MW turbine taps underground steam at 10-50 bar, common in Iceland or the U.S. West. Solar thermal plants employ them—a 50-200 MW unit uses mirrors to heat steam, as in Spain’s solar farms. Off-grid or backup systems use small units—a 50 kW turbine in a remote mine runs on diesel, powering critical loads. Their focus is electricity, not heat, making them less common in CHP compared to non-condensing turbines.
Efficiency in condensing steam turbines is higher than non-condensing types, typically 35-45% for electrical output, thanks to the large pressure drop to vacuum. A 500 MW turbine with supercritical steam (600°C, 300 bar) might convert 45% of fuel energy to electricity, losing 55% to condenser heat, friction, and leaks. Subcritical units (540°C, 100 bar) hit 35-40%, while small units (5 MW, 20 bar) drop to 25-30%. Losses include condenser heat (50-60%), blade turbulence (2-5%), and moisture in LP stages (5-10% output drop unless superheated or dried with separators). Enhancements like reheat—returning steam to the boiler at 20-50 bar for reheating to 540°C—add 4-5% efficiency, common in coal plants. Supercritical or ultra-supercritical steam (600-700°C, 300 bar) pushes efficiency to 47-50%, nearing the Rankine ideal of 60%. Fuel quality matters—coal (30 MJ/kg) outperforms wet biomass (10-15 MJ/kg), with moisture reducing steam output by 20-30% if not managed.
Historically, condensing steam turbines emerged with the rise of centralized power. Parsons’ 1884 turbine pioneered multi-stage design, scaling to megawatts by 1900 as electricity demand grew, outpacing steam engines (5-10% efficiency) with 20-30%. Early 20th-century coal plants used them, with metallurgy advances—nickel steels—enabling higher pressures by the 1930s. WWII saw them in naval ships and power stations, while post-war nuclear and gas plants adopted them, with reheat (1930s) and supercritical steam (1950s) lifting efficiency to 40%+. The 1970s energy crisis spurred efficiency focus, though renewables later diversified their use. Modern designs leverage CFD for blade optimization and AI for control, maintaining their dominance in power generation.
Modern relevance centers on large-scale electricity. Utility plants rely on them—coal, gas, and nuclear units like the 1,750 MW Arabelle power grids, though coal declines with emissions rules (e.g., Paris Agreement). Industrial self-generation grows—a 20 MW turbine cuts a factory’s $5 million yearly grid bill. Renewables expand their role—biomass (10-50 MW), geothermal (5-100 MW), and solar thermal (50-200 MW) support green energy, with a 20 MW wood-fired unit powering 10,000 homes. Innovations enhance them—ceramic blades handle 1,000°C, 3D-printed parts cut costs, and AI optimizes vacuum, though gas turbines challenge them in flexibility. Their scale—1,000 MW+—keeps them vital for baseload power.
Advantages include high electrical efficiency (35-45%), maximizing power—a 500 MW unit outproduces a non-condensing twin by 50%. Large capacity (up to 1,750 MW) suits grids, with a single turbine powering cities. Fuel flexibility spans coal, gas, nuclear, and renewables, adapting to local resources. Longevity—30-50 years—ensures reliability, running 8,000 hours/year. Challenges are complexity—condensers and cooling towers raise costs to $1,500-$2,000/kW vs. $500-$1,000/kW for non-condensing, with $100,000-$1 million yearly maintenance. Water use (1-2 liters/kWh) strains dry regions, and heat rejection (50-60%) wastes energy, unfit for CHP. Slow startups (hours vs. minutes for gas turbines) limit responsiveness.
Examples highlight their impact. A 1,000 MW coal turbine produces 800 MW, powering 1 million homes with a $50 million cost. A 10 MW biomass unit burns 2 tons/hour of wood, generating 8 MW for a rural grid, costing $5 million. A 50 kW diesel-fired turbine in a mine delivers 40 kW, costing $50,000.
Condensing steam turbines are power generation titans, excelling where electricity reigns supreme, their complexity offset by unmatched output and efficiency in a grid-driven world.
Here’s a continued exploration of condensing steam turbines, presented as a detailed plain text that builds on the previous discussion while offering deeper insights. Condensing steam turbines are engineered to maximize electrical power generation by expanding steam to a low-pressure vacuum, typically 0.05 to 0.1 bar, allowing for the greatest possible energy extraction from steam before it is condensed back into water. Unlike non-condensing turbines, which exhaust at higher pressures for heat use, condensing turbines prioritize electricity over thermal output, making them a cornerstone of utility-scale power plants, large industrial facilities, and certain renewable energy systems. With capacities ranging from a few kilowatts to over 1,000 megawatts, they achieve electrical efficiencies of 35-45%, or even higher with advanced designs, at the cost of rejecting significant waste heat. Their complexity—requiring boilers, condensers, and extensive cooling systems—suits applications where power generation is the primary goal. Let’s delve further into their mechanics, design intricacies, applications, operational specifics, historical evolution, modern significance, advantages, and challenges.
The mechanics of a condensing steam turbine are rooted in the Rankine cycle, optimized to extract maximum mechanical work from steam. A boiler generates steam by combusting fuels like coal, natural gas, oil, or biomass (e.g., wood pellets, straw), or harnessing heat from nuclear reactors, geothermal wells, or concentrated solar power, producing steam at pressures of 50 to 300 bar and temperatures of 400-600°C, with supercritical designs reaching 700°C. This high-pressure steam enters the turbine, flowing over blades mounted on a rotor—a massive forged steel shaft that can weigh 10-100 tons in large units or just a few kilograms in smaller ones. As the steam expands—dropping from, say, 100 bar to 0.05 bar—it accelerates to speeds of 500-700 meters per second, pushing the blades and spinning the rotor at speeds like 3,000 RPM for 50 Hz electrical grids, 3,600 RPM for 60 Hz, or up to 10,000 RPM in smaller, high-speed configurations. The rotor drives a generator to produce electricity or, less commonly, powers machinery such as pumps or compressors through a shaft, often with a gearbox to adjust speed—for instance, reducing 10,000 RPM to 1,500 RPM for industrial equipment. The steam then exhausts into a condenser—a heat exchanger that cools it back to water at 30-50°C using cold water from rivers, oceans, or cooling towers, or air in water-scarce areas—creating a vacuum that maximizes the pressure differential and energy yield. This condensed water is pumped back to the boiler, completing the closed cycle, while the cooling process discards 50-60% of the input energy as waste heat, a trade-off for high electrical output.
The design of condensing steam turbines is intricate, built to handle a broad pressure range and optimize efficiency. They are almost always multi-stage, featuring 10 to 50 stages divided into high-pressure (HP), intermediate-pressure (IP), and low-pressure (LP) sections, each tailored to its steam conditions. HP stages manage the initial high-pressure, high-temperature steam—e.g., 300 bar, 600°C—with small, sturdy blades (5-20 cm) made of nickel-based superalloys like Inconel 718 or Rene 41, designed to resist creep and corrosion at extreme conditions, often coated with chromium carbide or ceramics for durability. IP stages process reheated steam—say, 50 bar, 540°C—while LP stages handle low-pressure, high-volume steam (0.05 bar) with enormous blades, sometimes 1.5-2 meters long, made of titanium or stainless steel to withstand erosion from wet steam droplets at tip speeds of 500-700 m/s. Impulse designs are typical in HP stages—steam accelerates through nozzles into jets that strike bucket-shaped blades—while reaction designs dominate LP stages, where steam expands across moving blades, with combination turbines blending both for efficiency across stages. Rotors, weighing tons, are precision-balanced to spin without vibration, connected to generators via direct shafts or couplings. Casings, forged from high-strength steel or alloys, contain pressures up to 300 bar, with labyrinth seals or brush seals minimizing leakage—a critical feature given the steep drop to vacuum. Condensers, often shell-and-tube or surface designs, use 1-2 liters of cooling water per kWh or air fans, maintaining the vacuum while rejecting heat, supported by cooling towers that evaporate 1-2% of output as waste or air systems that reduce water use at a 5-10% efficiency cost.
Condensing steam turbine generators pair the turbine with a synchronous generator to produce electricity, sized for the application. A 500 MW utility unit generates 13.8 kV AC for grid transmission, stepped up to 220-500 kV, while a 5 MW industrial unit produces 400 V AC for local use, synchronized at 50 or 60 Hz. Generators feature rotor poles spinning within stator windings, cooled by hydrogen in large units (better heat transfer) or air in smaller ones, dissipating heat from 50-60% energy losses, with transformers and regulators adjusting output. Boilers, producing 100-1,000 tons/hour of steam, integrate with fuels like coal (30 MJ/kg), natural gas (clean, 50 MJ/kg), or biomass (10-20 MJ/kg), or heat from nuclear reactors (saturated steam at 70 bar) or solar mirrors (400-540°C). Cooling systems are extensive—water-based condensers process millions of liters daily, with cooling towers rejecting heat via evaporation, while air-cooled condensers suit arid regions, though they lower efficiency by 5-10%. Controls are advanced—digital systems with sensors monitor pressure, temperature, vacuum levels, and rotor speed, adjusting steam flow and cooling water to maximize output, with costs ranging from $50,000 for a 5 MW unit to $50-100 million for a 500-1,000 MW plant.
Applications of condensing steam turbines focus on power generation where electricity is the priority. Utility power plants are their stronghold—coal-fired units (37% of global power) range from 100-1,000 MW, gas-fired combined-cycle plants (25%) reach 500-1,500 MW with gas turbine integration, and nuclear plants (10%) hit 1,000-1,750 MW, powering millions—e.g., a 1,200 MW nuclear turbine serves 1.5-2 million homes. Large industrial facilities use them for self-generation—a 5-50 MW turbine powers a chemical plant or steel mill, fueled by gas, coal, or waste heat, meeting onsite demand without grid reliance. Biomass plants leverage them for renewable energy—a 10-50 MW unit burns 2-10 tons/hour of wood or agricultural waste, supplying rural grids or industrial parks, common in Scandinavia or North America. Geothermal systems depend on them—a 5-100 MW turbine uses steam from underground reservoirs at 10-50 bar, as in New Zealand or the U.S. Geothermal Belt. Solar thermal plants employ them—a 50-200 MW unit uses concentrated sunlight to heat steam, as in California’s solar farms. Off-grid or backup systems use smaller units—a 50 kW turbine in a remote mine runs on diesel or biomass, powering critical equipment. Unlike non-condensing turbines, they rarely serve CHP, as their heat is discarded, not reused.
Efficiency in condensing steam turbines is among the highest for steam-based systems, typically 35-45% for electrical output, driven by the large pressure drop to vacuum. A 500 MW turbine with supercritical steam (600°C, 300 bar) might achieve 45% efficiency, converting 45% of fuel energy to electricity and losing 55% to condenser heat, friction, and leaks. Subcritical units (540°C, 100 bar) reach 35-40%, while small units (5 MW, 20 bar) drop to 25-30% due to scale and simpler staging. Losses include condenser heat rejection (50-60%), blade turbulence (2-5%), and moisture in LP stages (5-10% output reduction unless superheated to 540-600°C or dried with moisture separators). Reheat cycles—returning steam to the boiler at 20-50 bar for reheating to 540°C—boost efficiency by 4-5%, standard in coal and nuclear plants, while double reheat adds another 2-3% in ultra-efficient designs. Supercritical (600°C, 300 bar) and ultra-supercritical (700°C, 350 bar) steam push efficiency to 47-50%, nearing the Rankine ideal of 60%, though material limits (creep, corrosion) cap gains. Fuel quality impacts output—coal (30 MJ/kg) or gas (50 MJ/kg) outperforms wet biomass (10-15 MJ/kg), with high moisture (20-50%) cutting steam production by 20-30% unless pre-dried.
Historically, condensing steam turbines emerged as electricity demand surged. Charles Parsons’ 1884 multi-stage turbine laid the foundation, generating 7.5 kW and proving the concept, scaling to megawatts by 1900 as grids expanded, surpassing steam engines (5-10% efficiency) with 20-30%. Early 20th-century coal plants adopted them, with metallurgy advances—nickel and chromium steels—enabling higher pressures and temperatures by the 1930s. World War II saw them in naval ships (e.g., 29,000 hp on the Titanic) and power stations, while post-war nuclear plants (1950s) and gas combined-cycle systems (1970s) embraced them, with reheat (1930s) and supercritical steam (1950s) lifting efficiency to 40%+. The 1970s energy crisis drove efficiency improvements, and renewables later diversified their fuel sources. Modern designs use computational fluid dynamics (CFD) for blade optimization, advanced alloys for durability, and AI for real-time control, cementing their role in power generation.
Modern significance reflects their dominance in electricity production. Utility plants depend on them—coal, gas, and nuclear units like the 1,750 MW Arabelle turbine power grids, though coal’s share shrinks under emissions policies (e.g., net-zero by 2050 goals). Industrial self-generation rises—a 20 MW turbine saves a refinery $5-10 million yearly vs. grid power, fueled by gas or waste heat. Renewables expand their scope—biomass (10-50 MW), geothermal (5-100 MW), and solar thermal (50-200 MW) align with green energy, with a 20 MW biomass unit powering 10,000 homes on 4 tons/hour of wood. Innovations keep them competitive—ceramic blades tolerate 1,000°C, 3D-printed components reduce costs by 10-20%, and AI maintains vacuum efficiency, though gas turbines (faster startups) and solar (lower cost/W) challenge them. Their scale—500-1,000 MW+—ensures they remain critical for baseload power in a decarbonizing world.
Advantages include high electrical efficiency (35-45%), outpacing non-condensing turbines (20-30%)—a 500 MW unit produces 225 MW vs. 150 MW from a non-condensing peer. Massive capacity (up to 1,750 MW) powers entire regions—a single turbine supports 2 million homes. Fuel flexibility spans coal, gas, nuclear, biomass, and solar, adapting to local conditions. Longevity—30-50 years with maintenance—delivers reliability, running 8,000 hours/year. Challenges are significant—complexity drives costs to $1,500-$2,000/kW vs. $500-$1,000/kW for non-condensing, with $100,000-$1 million yearly maintenance for condensers, blades, and boilers. Water demand (1-2 liters/kWh) strains arid areas—e.g., a 500 MW plant uses 2 million liters/hour—while air cooling cuts efficiency by 5-10%. Heat rejection (50-60%) wastes energy, making them unfit for CHP, and slow startups (hours vs. minutes for gas turbines) limit flexibility.
Examples illustrate their scope. A 1,000 MW coal turbine generates 800 MW, powering 1 million homes with a $50-70 million cost. A 10 MW biomass unit burns 2 tons/hour of wood, producing 8 MW for a rural grid, costing $5-7 million. A 50 kW diesel-fired turbine in a mine delivers 40 kW, costing $50,000 but saving $20,000 yearly vs. generators.
Condensing steam turbines are power generation heavyweights, excelling where electricity is king, their intricate design and high output anchoring grids and industries despite a shifting energy landscape.
Automatic Extraction Non Condensing Turbine
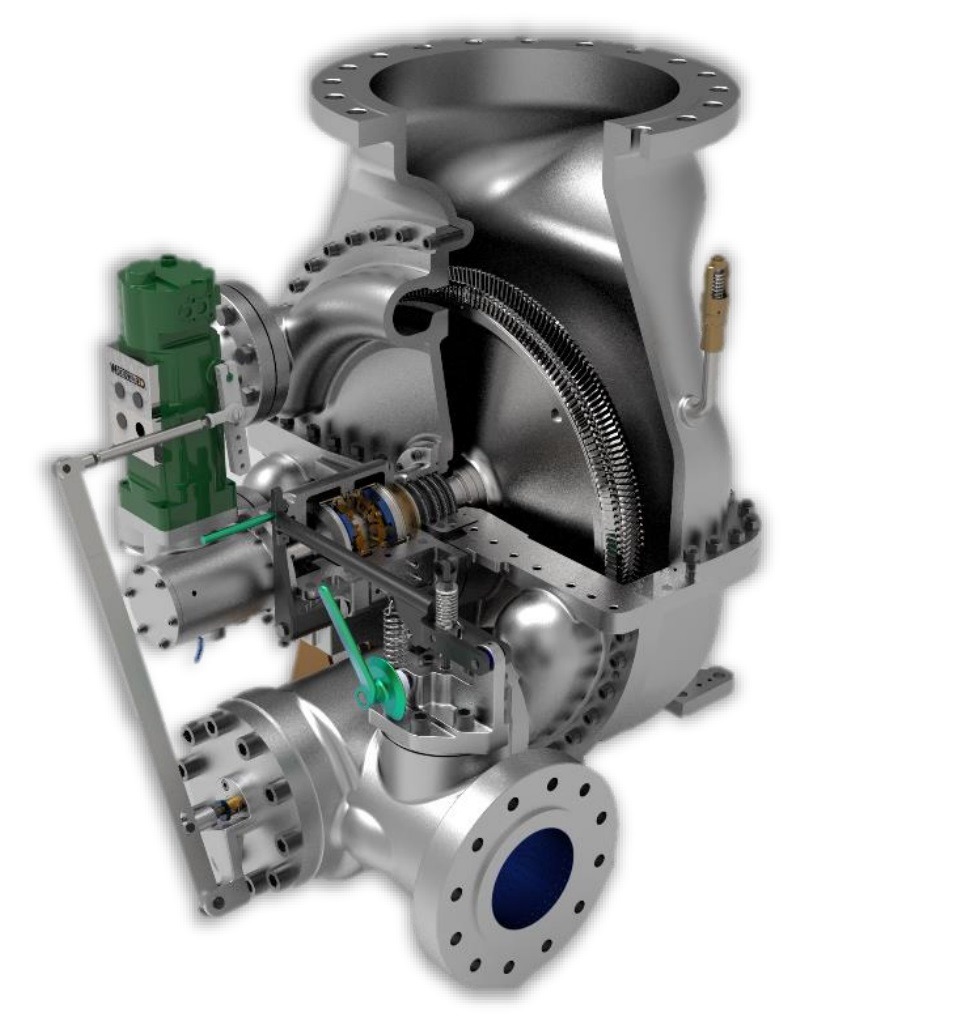
Here’s a detailed exploration of automatic extraction non-condensing steam turbines, presented as a long plain text. Automatic extraction non-condensing steam turbines, also known as extraction back-pressure turbines, are a specialized type of steam turbine designed to produce mechanical power—typically for electricity generation or driving machinery—while simultaneously extracting steam at one or more intermediate pressures for industrial processes or heating, with the final exhaust exiting at a pressure above atmospheric levels, usually 2-20 bar. Unlike condensing turbines, which exhaust into a vacuum to maximize power, these turbines prioritize flexibility, balancing electrical output with the delivery of usable steam at specific pressures tailored to downstream needs. They are widely used in industrial settings like chemical plants, refineries, and paper mills, as well as in combined heat and power (CHP) systems, with capacities ranging from a few kilowatts to tens of megawatts. The “automatic” feature refers to sophisticated control systems that adjust extraction flows in real time, ensuring optimal performance under varying demands. Let’s dive into their mechanics, design features, applications, operational details, historical context, modern relevance, advantages, and challenges.
The mechanics of an automatic extraction non-condensing steam turbine are based on the Rankine cycle, modified to allow controlled steam extraction and a non-condensing exhaust. A boiler generates steam by burning fuels such as coal, natural gas, oil, or biomass (e.g., wood chips, bagasse), or capturing waste heat from industrial processes—say, exhaust from a furnace at 400-600°C. Steam enters the turbine at high pressures, typically 20-100 bar, and temperatures of 200-540°C, depending on the system’s scale and fuel source. This steam flows over blades mounted on a rotor—a forged steel shaft weighing from a few kilograms in small units to several tons in larger ones. As the steam expands—dropping from, say, 50 bar to an extraction point at 15 bar, then to a final exhaust at 5 bar—it accelerates to speeds of 300-500 meters per second, pushing the blades and spinning the rotor at speeds like 3,000 RPM for 50 Hz grids, 3,600 RPM for 60 Hz, or up to 10,000 RPM in high-speed designs. The rotor drives a generator to produce electricity or powers machinery like pumps or compressors via a shaft, often with a gearbox to adjust speed. Steam is extracted at one or more intermediate stages—e.g., 15 bar for a chemical process, 3 bar for heating—through extraction ports controlled by automatic valves, with the remaining steam exhausting at a higher-than-atmospheric pressure (2-20 bar) for additional thermal use, such as drying or district heating. This dual-purpose operation, managed by automated controls, distinguishes them from simpler non-condensing turbines.
The design of automatic extraction non-condensing steam turbines is complex, blending flexibility with efficiency. They are multi-stage, typically with 5-20 stages divided into sections: an initial high-pressure (HP) section before the first extraction, intermediate sections for each extraction point, and a final low-pressure (LP) section before exhaust. HP stages handle entry conditions—e.g., 50 bar, 540°C—with small blades (5-20 cm) made of nickel alloys like Inconel to resist heat and pressure, coated with chromium for durability. Extraction stages manage mid-range pressures—e.g., 15 bar—with blades sized accordingly (10-30 cm), while LP stages process the final expansion to 5-20 bar with larger blades (up to 50 cm), often stainless steel to handle wetter steam. Impulse designs are used in HP stages—steam jets strike buckets—while reaction designs dominate LP stages, with combination turbines blending both for efficiency across pressure drops. Extraction ports, fitted with throttling valves or nozzles, divert steam to external piping, with flow rates adjusted by automatic control systems—e.g., PLCs or DCS—responsive to downstream demand signals. Rotors, forged steel, are balanced for high speeds, while casings—cast steel or alloys—contain 100 bar, with labyrinth seals minimizing leakage, less critical here than in condensing turbines due to higher exhaust pressure. No condenser is needed, simplifying the system—exhaust steam pipes directly to process or heating loads.
Automatic extraction non-condensing steam turbine generators pair the turbine with a synchronous generator, sized for the application. A 10 MW unit might produce 400 V or 13.8 kV AC, synchronized at 50 or 60 Hz, while a 500 kW unit generates 230 V AC for local use. Generators feature rotor poles spinning in stator windings, cooled by air or water, with voltage regulators adjusting output as extraction varies power. Boilers, producing 10-100 tons/hour, use fuels like natural gas (clean), biomass (local), or waste heat (free). Controls are the heart of the “automatic” feature—sensors monitor steam pressure, temperature, and flow at extraction points and exhaust, feeding data to a control system that adjusts valves in real time, ensuring stable power and steam delivery under fluctuating loads. Costs range from $50,000 for a 500 kW unit to $2-5 million for a 10-20 MW system, reflecting added complexity.
Applications center on industries needing variable steam and power. Chemical plants use them extensively—a 10 MW turbine extracts 15-bar steam (10 tons/hour) for reactors and 3-bar steam (5 tons/hour) for heating, producing 7 MW of electricity, fueled by gas or waste heat. Refineries rely on them—a 20 MW unit extracts 20-bar steam for cracking and 5-bar steam for distillation, generating 15 MW, often burning refinery byproducts. Paper mills adopt them—a 5 MW turbine extracts 10-bar steam (8 tons/hour) to dry pulp and exhausts at 2 bar for heating, producing 4 MW from wood waste. Sugar mills use them with bagasse—a 3 MW turbine extracts 15-bar steam for evaporators and exhausts at 5 bar, generating 2 MW. Food processing plants employ smaller units—a 1 MW turbine extracts 5-bar steam for sterilization and exhausts at 2 bar, producing 800 kW. District heating with power generation uses them—a 5 MW turbine extracts 10-bar steam for industrial use and exhausts at 3 bar for homes, generating 4 MW. Off-grid setups benefit too—a 100 kW turbine extracts 5-bar steam for a farm process and exhausts at 2 bar, powering 80 kW.
Efficiency balances power and heat. Electrical efficiency is 20-35%, lower than condensing turbines (35-45%) due to extraction and higher exhaust pressure. A 10 MW turbine (50 bar, 540°C) might produce 3 MW (30% efficiency), with 40% as extracted steam (15 bar), 25% as exhaust steam (5 bar), and 5-10% lost to leaks and friction. Total CHP efficiency hits 70-85%, excelling in energy use—e.g., a 5 MW unit yields 1.5 MW power, 2 MW extracted steam, and 1 MW exhaust heat. Losses include incomplete expansion (5-20 bar exhaust vs. 0.05 bar), blade inefficiencies, and leakage (5-10%). Multi-stage designs and superheating to 540°C boost electrical efficiency to 35%, but extraction reduces available steam for power. Fuel quality matters—dry biomass (4.5 kWh/kg) outperforms wet (3 kWh/kg), with 20%+ moisture cutting output by 30%.
Historically, these turbines evolved from early 20th-century needs for flexible steam use. Parsons’ 1884 multi-stage design and De Laval’s impulse turbine enabled extraction concepts, adopted by industries by the 1920s over steam engines (5-10% efficiency) for 15-25% efficiency plus heat. Pre-WWII plants used coal-fired units for power and process steam, scaling up by the 1930s. WWII saw them in factories needing adaptability, while post-war automation (1950s-60s) added control sophistication. The 1970s energy crisis boosted CHP use, with biomass and waste heat rising. Modern designs leverage digital controls and alloys for efficiency.
Modern relevance lies in industrial flexibility and CHP. Chemical and refining sectors save $1-3 million yearly—a 10 MW turbine meets variable steam needs cost-effectively. Biomass use grows—a 5 MW unit burns 2 tons/hour of waste, cutting fossil fuel costs. Waste heat recovery expands—a 2 MW turbine uses 400°C exhaust, saving $500,000 annually. District heating pairs them with power—a 5 MW unit heats 1,000 homes and generates 4 MW. Automation—PLCs, sensors—optimizes performance, while coatings handle 540°C, though condensing turbines lead in pure power.
Advantages include flexibility—automatic extraction meets shifting demands, e.g., 15-bar to 5-bar steam on the fly. High CHP efficiency (70-85%) maximizes energy—a 5 MW unit delivers 4 MW total output. Simplicity—no condenser—cuts costs to $500-$1,000/kW vs. $1,500-$2,000/kW for condensing. Fuel flexibility suits coal, biomass, or waste heat. Reliability lasts 20-30 years, running 5,000-8,000 hours/year. Challenges are lower electrical efficiency (20-35%)—a 10 MW unit yields 3 MW vs. 4-5 MW condensing. Complexity of controls raises costs—$50,000-$100,000 for automation. Maintenance—valves, blades, seals—costs $10,000-$50,000 yearly. Biomass emissions (50-200 mg/m³) need filters ($10,000-$50,000). Fuel inconsistency cuts output by 20-30%.
Examples show their value. A 10 MW refinery turbine extracts 20-bar and 5-bar steam, producing 7 MW, saving $2 million yearly. A 1 MW food plant unit extracts 5-bar steam, generates 800 kW, costing $100,000 but saving $50,000 annually. A 5 MW paper mill turbine extracts 10-bar steam, exhausts at 2 bar, producing 4 MW from wood, saving $1 million.
Automatic extraction non-condensing turbines excel in balancing power and steam, their adaptability and efficiency making them vital for industries needing both, bridging legacy steam tech with modern demands.
Here’s a continued exploration of automatic extraction non-condensing steam turbines, presented as a detailed plain text that builds on the previous discussion with added depth and nuance. Automatic extraction non-condensing steam turbines, often called extraction back-pressure turbines, are advanced steam turbines designed to generate mechanical power—typically for electricity or machinery—while extracting steam at one or more intermediate pressures for industrial processes or heating, with the final exhaust exiting at a pressure above atmospheric levels, usually 2-20 bar. Unlike condensing turbines that exhaust into a vacuum for maximum power, these turbines offer a flexible balance between electrical output and thermal energy delivery, with automatic control systems adjusting extraction flows to meet real-time demands. They are ideal for industrial applications like refineries, chemical plants, and pulp mills, as well as combined heat and power (CHP) setups, with capacities ranging from a few kilowatts to tens of megawatts. Their ability to adapt to variable steam needs while maintaining efficiency makes them a standout choice in complex energy systems. Let’s delve deeper into their mechanics, design intricacies, applications, operational specifics, historical evolution, modern significance, advantages, and challenges.
The mechanics of an automatic extraction non-condensing steam turbine follow the Rankine cycle, tailored to provide both power and controlled steam output. A boiler produces steam by combusting fuels such as coal, natural gas, oil, or biomass (e.g., wood pellets, rice husks, or sugarcane bagasse), or by capturing waste heat from sources like industrial exhausts at 400-600°C or engine tailpipes at 300-400°C. Steam enters the turbine at high pressures—typically 20-100 bar—and temperatures of 200-540°C, depending on the system’s design and fuel. This steam flows over blades mounted on a rotor—a forged steel shaft ranging from a few kilograms in small units to several tons in larger ones. As the steam expands—say, from 50 bar to an extraction point at 15 bar, then to a final exhaust at 5 bar—it accelerates to speeds of 300-500 meters per second, driving the blades and spinning the rotor at speeds like 3,000 RPM for 50 Hz grids, 3,600 RPM for 60 Hz, or up to 10,000 RPM in high-speed configurations. The rotor powers a generator for electricity or drives machinery like pumps, fans, or compressors via a shaft, often with a gearbox—e.g., reducing 10,000 RPM to 1,500 RPM for a pump. Steam is extracted at intermediate stages—e.g., 15 bar for a process, 3 bar for preheating—through ports with automatic valves, while the remaining steam exhausts at 2-20 bar for additional thermal use, such as drying or heating. Automated controls—sensors and actuators tied to a programmable logic controller (PLC) or distributed control system (DCS)—adjust extraction flows dynamically, ensuring stable power and steam delivery as demands shift, a key feature setting them apart from manual extraction turbines.
The design of these turbines is sophisticated, engineered for flexibility and multi-stage operation. They feature 5-20 stages, segmented into high-pressure (HP) sections before the first extraction, intermediate sections for each extraction point, and a low-pressure (LP) section before exhaust. HP stages handle inlet steam—e.g., 50 bar, 540°C—with small blades (5-20 cm) made of nickel-based alloys like Inconel 718 or Hastelloy, resistant to 540°C and 100 bar, often coated with chromium carbide or ceramics to combat creep and corrosion. Extraction stages manage mid-range pressures—e.g., 15 bar or 5 bar—with blades (10-30 cm) sized for flow, while LP stages process the final drop to 5-20 bar with larger blades (up to 50 cm), typically stainless steel to handle wetter steam. Impulse designs dominate HP stages—steam jets from nozzles strike buckets—while reaction designs prevail in LP stages, with steam expanding across moving blades; combination turbines blend both for efficiency across the pressure range. Extraction ports, equipped with throttling valves or adjustable nozzles, divert steam to external piping, controlled by servo-motors linked to the automation system, which responds to pressure, temperature, or flow signals from downstream processes. Rotors, forged steel, are balanced for high speeds, while casings—cast steel or alloys—contain up to 100 bar, with labyrinth seals or packing glands reducing leakage, though less critical than in condensing turbines due to the higher exhaust pressure. No condenser is required—exhaust steam feeds directly into process lines or heat exchangers, simplifying the setup compared to condensing systems.
Automatic extraction non-condensing steam turbine generators couple the turbine with a synchronous generator, scaled to the system’s needs. A 10 MW unit might produce 400 V or 13.8 kV AC for industrial or grid use, synchronized at 50 or 60 Hz, while a 500 kW unit generates 230 V AC for smaller loads. Generators feature rotor poles spinning within stator windings, cooled by air (smaller units) or water (larger ones) to manage heat from 50-70% energy losses, with voltage regulators adjusting output as extraction alters power. Boilers, producing 10-100 tons/hour, integrate with fuels like natural gas (high efficiency), biomass (local sustainability), or waste heat (cost-free energy). The automation system is central—sensors at extraction points and exhaust monitor conditions, feeding data to a PLC or DCS that adjusts valves in milliseconds, balancing power and steam under variable loads—e.g., increasing 15-bar extraction when a reactor demands more, reducing it when power is prioritized. Costs reflect this complexity—$50,000-$100,000 for a 500 kW unit, $2-5 million for a 10-20 MW system, driven by controls and staging.
Applications focus on industries with fluctuating steam and power needs. Chemical plants are a key user—a 10 MW turbine extracts 15-bar steam (10 tons/hour) for reactors and 3-bar steam (5 tons/hour) for preheating, generating 7 MW, fueled by gas or process waste heat. Oil refineries depend on them—a 20 MW turbine extracts 20-bar steam for cracking units and 5-bar steam for distillation, producing 15 MW, often using byproduct fuels like petroleum coke. Pulp and paper mills leverage them—a 5 MW turbine extracts 10-bar steam (8 tons/hour) to dry pulp and exhausts at 2 bar for mill heating, generating 4 MW from wood waste. Sugar mills use them with bagasse—a 3 MW turbine extracts 15-bar steam for evaporators and exhausts at 5 bar, producing 2 MW during harvest. Food processing benefits—a 1 MW turbine extracts 5-bar steam for sterilization and exhausts at 2 bar for drying, generating 800 kW, often gas-fired. District heating with cogeneration employs them—a 5 MW turbine extracts 10-bar steam for industrial clients and exhausts at 3 bar for residential heating, producing 4 MW. Off-grid sites use smaller units—a 100 kW turbine extracts 5-bar steam for a farm process and exhausts at 2 bar, generating 80 kW from wood or biogas.
Efficiency reflects their dual role. Electrical efficiency ranges from 20-35%, lower than condensing turbines (35-45%) due to extraction and higher exhaust pressure. A 10 MW turbine (50 bar, 540°C) might produce 3 MW (30% efficiency), with 40% as extracted steam (15 bar, 4 MW thermal), 25% as exhaust steam (5 bar, 2.5 MW thermal), and 5-10% lost to friction, turbulence, and leaks. Total CHP efficiency reaches 70-85%, optimizing energy use—e.g., a 5 MW unit yields 1.5 MW power, 2 MW extracted steam, and 1 MW exhaust heat. Losses stem from incomplete expansion (5-20 bar exhaust vs. 0.05 bar), blade inefficiencies (fewer stages than condensing units), and leakage (5-10% with basic seals). Multi-stage designs (10-20 stages) and superheating to 540°C lift electrical efficiency to 35%, but extraction reduces steam available for power, a deliberate trade-off. Fuel quality impacts output—dry biomass (4.5 kWh/kg) outperforms wet (3 kWh/kg), with moisture above 20% cutting steam by 30% unless pre-dried, a frequent issue in biomass systems.
Historically, these turbines emerged from early 20th-century industrial demands for flexible steam. Charles Parsons’ 1884 multi-stage turbine enabled extraction concepts, with De Laval’s impulse design adding speed, adopted by factories by the 1920s for 15-25% efficiency plus heat, outpacing steam engines (5-10%). Pre-WWII plants used coal-fired units for power and process steam, scaling up by the 1930s with better steels. WWII saw them in adaptable factory setups—e.g., a 1 MW unit powered and heated a site on limited fuel. Post-war automation (1950s-60s) introduced valve controls, while the 1970s energy crisis boosted CHP use with biomass and waste heat. Modern designs enhance this—digital systems optimize extraction, and alloys handle 540°C, refining their niche.
Modern significance lies in industrial adaptability and CHP efficiency. Chemical and refining industries save $1-3 million yearly—a 10 MW turbine adjusts steam on demand, cutting costs vs. separate systems. Biomass adoption rises—a 5 MW unit burns 2 tons/hour of waste, reducing fossil fuel use in rural mills. Waste heat recovery grows—a 2 MW turbine uses 400°C exhaust, saving $500,000 annually emissions-free. District heating pairs power and heat—a 5 MW unit serves 1,000 homes and generates 4 MW. Automation ensures precision—sensors and PLCs adapt to load shifts, while coatings and modular designs improve durability and cost, though condensing turbines dominate pure power output.
Advantages include unmatched flexibility—automatic extraction adjusts steam from 20 bar to 2 bar instantly, meeting variable needs. High CHP efficiency (70-85%) maximizes energy—a 5 MW unit delivers 4 MW total output vs. 1.5 MW from a standalone generator. Simplicity—no condenser—cuts costs to $500-$1,000/kW vs. $1,500-$2,000/kW for condensing, easing maintenance. Fuel flexibility spans coal, biomass, and waste heat, fitting local resources. Reliability endures—20-30 years, 5,000-8,000 hours/year. Challenges include lower electrical efficiency (20-35%)—a 10 MW unit yields 3 MW vs. 4-5 MW condensing. Control complexity adds $50,000-$100,000 to costs. Maintenance—valves, blades, seals—runs $10,000-$50,000 yearly. Biomass emissions (50-200 mg/m³) need filters ($10,000-$50,000). Fuel variability cuts output by 20-30% if inconsistent.
Examples highlight their role. A 10 MW refinery turbine extracts 20-bar and 5-bar steam, produces 7 MW, saving $2 million yearly. A 1 MW food plant unit extracts 5-bar steam, generates 800 kW, costing $100,000 but saving $50,000 annually. A 5 MW paper mill turbine extracts 10-bar steam, exhausts at 2 bar, produces 4 MW from wood, saving $1 million.
Automatic extraction non-condensing turbines bridge power and process needs with precision, their adaptability and efficiency anchoring complex industrial energy systems in a versatile, sustainable way.
Automatic Extraction Condensing Turbine
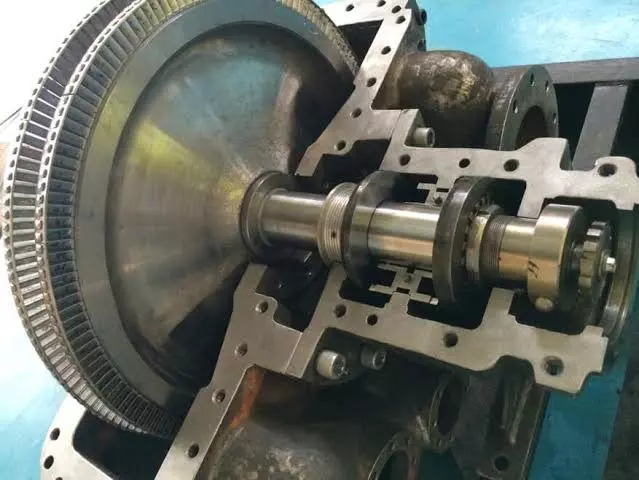
Here’s a detailed exploration of automatic extraction condensing steam turbines, presented as a long plain text. Automatic extraction condensing steam turbines are a sophisticated hybrid of steam turbine designs, combining the ability to extract steam at one or more intermediate pressures for industrial processes or heating with the capability to exhaust the remaining steam into a vacuum, typically 0.05 to 0.1 bar, to maximize electrical power generation. These turbines are engineered to provide both mechanical power—usually driving a generator—and controlled steam output, with automatic systems adjusting extraction flows to meet fluctuating demands, making them highly versatile. They are commonly used in large industrial facilities like refineries, chemical plants, and power plants with CHP (combined heat and power) needs, with capacities ranging from a few megawatts to hundreds of megawatts. Their dual focus on high electrical efficiency and process steam delivery sets them apart from simpler condensing or non-condensing turbines. Let’s dive into their mechanics, design features, applications, operational details, historical context, modern relevance, advantages, and challenges.
The mechanics of an automatic extraction condensing steam turbine are based on the Rankine cycle, adapted to balance maximum power extraction with controlled steam delivery. A boiler generates steam by combusting fuels like coal, natural gas, oil, or biomass (e.g., wood chips, bagasse), or using heat from nuclear reactors, waste heat recovery (e.g., 400-600°C industrial exhaust), or solar thermal systems, producing steam at pressures of 50-300 bar and temperatures of 400-600°C, with advanced units reaching 700°C in supercritical designs. This steam enters the turbine, flowing over blades on a rotor—a forged steel shaft weighing tons in large units or kilograms in smaller ones. As the steam expands—say, from 100 bar to an extraction point at 15 bar, then to a final exhaust at 0.05 bar—it accelerates to speeds of 500-700 meters per second, driving the blades and spinning the rotor at speeds like 3,000 RPM for 50 Hz grids, 3,600 RPM for 60 Hz, or up to 10,000 RPM in smaller setups. The rotor powers a generator for electricity or drives machinery via a shaft, often with a gearbox—e.g., reducing 10,000 RPM to 1,500 RPM. Steam is extracted at intermediate stages—e.g., 15 bar for a process, 3 bar for heating—through ports with automatic valves, while the remaining steam exhausts into a condenser, cooling back to water at 30-50°C under vacuum conditions using water from rivers or cooling towers, or air in dry regions. This vacuum maximizes the pressure drop and power output, while automated controls—PLCs or DCS—adjust extraction flows in real time, responding to changes in steam or power demand, ensuring stability and efficiency.
The design of these turbines is complex, integrating multi-stage expansion with extraction and condensing features. They feature 10-50 stages, divided into high-pressure (HP) sections before the first extraction, intermediate sections for extraction points, and low-pressure (LP) sections leading to the condenser. HP stages handle inlet steam—e.g., 100 bar, 600°C—with small blades (5-20 cm) made of nickel-based superalloys like Inconel or Rene 41, resistant to extreme conditions, coated with chromium or ceramics for durability. Extraction stages manage mid-range pressures—e.g., 15 bar or 5 bar—with blades (10-30 cm) sized for flow, while LP stages process the final drop to 0.05 bar with large blades (up to 2 meters), typically titanium or stainless steel to resist wet steam erosion at tip speeds of 500-700 m/s. Impulse designs dominate HP stages—steam jets strike buckets—while reaction designs prevail in LP stages, with combination turbines blending both for efficiency. Extraction ports, fitted with throttling valves or nozzles, divert steam to external piping, controlled by servo-motors tied to an automation system that adjusts based on pressure, temperature, or flow signals. Rotors, weighing 10-100 tons in large units, are balanced for high speeds, while casings—forged steel or alloys—contain up to 300 bar, with labyrinth seals minimizing leakage across stages, critical due to the steep pressure drop to vacuum. Condensers—shell-and-tube or surface types—use 1-2 liters of cooling water per kWh or air fans, maintaining the vacuum and rejecting 50-60% of heat as waste.
Automatic extraction condensing steam turbine generators pair the turbine with a synchronous generator, scaled to the system’s capacity. A 50 MW unit might produce 13.8 kV AC for grid transmission, while a 5 MW unit generates 400 V AC for industrial use, synchronized at 50 or 60 Hz. Generators feature rotor poles spinning in stator windings, cooled by hydrogen (large units) or air (smaller ones), with voltage regulators adjusting output as extraction alters power. Boilers, producing 10-1,000 tons/hour, use fuels like coal (30 MJ/kg), gas (50 MJ/kg), or biomass (10-20 MJ/kg), or heat from nuclear or solar sources. Cooling systems are extensive—water towers process millions of liters daily, air-cooled condensers suit arid areas at a 5-10% efficiency cost. Automation is key—sensors monitor extraction points, exhaust vacuum, and rotor speed, feeding data to a PLC or DCS that adjusts valves in milliseconds, optimizing power and steam under variable loads, with costs from $1-2 million for a 5 MW unit to $50-100 million for a 50-100 MW system.
Applications focus on large industrial and power generation settings with dual needs. Refineries use them extensively—a 50 MW turbine extracts 20-bar steam (20 tons/hour) for cracking and 5-bar steam (10 tons/hour) for distillation, generating 40 MW, fueled by gas or byproduct fuels. Chemical plants rely on them—a 20 MW turbine extracts 15-bar steam (15 tons/hour) for reactors and 3-bar steam (5 tons/hour) for heating, producing 15 MW, often using waste heat. Pulp and paper mills adopt them—a 10 MW turbine extracts 10-bar steam (10 tons/hour) to dry pulp, generating 8 MW from wood waste, with exhaust to a condenser. Power plants with CHP use them—a 100 MW turbine extracts 15-bar steam for industrial clients and generates 80 MW for the grid, fueled by coal or gas. Food processing benefits—a 5 MW turbine extracts 5-bar steam for sterilization, producing 4 MW, often gas-fired. Biomass plants employ them—a 20 MW turbine extracts 10-bar steam for factory use and generates 15 MW from wood, supporting rural grids. Their ability to serve both power and steam makes them versatile, though less common in pure power plants than straight condensing turbines.
Efficiency balances power and extraction. Electrical efficiency is 30-40%, lower than pure condensing turbines (35-45%) due to steam diverted for extraction. A 20 MW turbine (100 bar, 540°C) might produce 8 MW (40% efficiency without extraction), but with 15-bar and 5-bar extraction, it yields 6 MW (30% efficiency), with 40% as extracted steam (8 MW thermal) and 20-25% lost to condenser heat and friction. Total CHP efficiency reaches 70-80%, leveraging steam use—e.g., a 10 MW unit yields 3 MW power and 6 MW thermal. Losses include condenser heat (50-60% without extraction), blade turbulence (2-5%), and moisture in LP stages (5-10% unless superheated to 540°C). Reheat—returning steam to 540°C at 20-50 bar—boosts efficiency by 4-5%, common in large units. Fuel quality affects output—coal outperforms wet biomass, with 20%+ moisture cutting steam by 30%.
Historically, these turbines evolved from early 20th-century multi-stage designs. Parsons’ 1884 turbine enabled extraction, scaled up by the 1930s for industrial power and steam, surpassing steam engines (5-10% efficiency) with 20-30%. WWII used them in flexible plants, while post-war automation (1950s-60s) added control precision. The 1970s energy crisis drove CHP adoption, with modern designs using digital systems and alloys for efficiency.
Modern relevance ties to industrial versatility. Refineries and chemical plants save $2-5 million yearly—a 20 MW turbine meets variable steam and power needs cost-effectively. Biomass and waste heat use grows—a 10 MW unit burns 2 tons/hour of wood or uses 400°C exhaust, cutting emissions. Power plants with CHP benefit—a 50 MW unit powers grids and supplies steam. Automation optimizes performance—PLCs adjust extraction instantly—while coatings handle 600°C, though pure condensing turbines lead in grid-scale power.
Advantages include flexibility—automatic extraction adjusts steam from 15 bar to 5 bar on demand. High electrical efficiency (30-40%) beats non-condensing (20-35%), with CHP at 70-80%. Large capacity (up to 100 MW) suits big loads. Fuel flexibility spans coal, gas, biomass, and waste heat. Challenges are complexity—condensers and controls raise costs to $1,500-$2,000/kW vs. $500-$1,000/kW for non-condensing, with $50,000-$200,000 yearly maintenance. Water use (1-2 liters/kWh) strains dry areas. Heat rejection (20-25%) limits thermal reuse vs. non-condensing systems.
Examples show their impact. A 20 MW refinery turbine extracts 20-bar and 5-bar steam, generates 15 MW, saving $3 million yearly. A 5 MW chemical unit extracts 10-bar steam, produces 4 MW, costing $1 million but saving $500,000 annually. A 50 MW power plant turbine extracts 15-bar steam, generates 40 MW, powering 20,000 homes.
Automatic extraction condensing turbines excel where power and steam demands intersect, their adaptability and efficiency making them a powerhouse in industrial and CHP applications.
Here’s a continued exploration of automatic extraction condensing steam turbines, presented as a detailed plain text that builds on the previous discussion with deeper insights and expanded perspectives. Automatic extraction condensing steam turbines are advanced systems that integrate the ability to extract steam at one or more intermediate pressures for industrial or heating purposes with the capability to exhaust the remaining steam into a vacuum, typically 0.05 to 0.1 bar, to maximize electrical power generation. These turbines are designed to deliver mechanical power—most often driving a generator—while providing controlled steam output, with sophisticated automatic control systems dynamically adjusting extraction flows to meet fluctuating process or power demands. They are widely deployed in large industrial complexes such as refineries, chemical plants, and power stations with combined heat and power (CHP) requirements, offering capacities from a few megawatts to hundreds of megawatts. Their hybrid design balances high electrical efficiency with flexible steam delivery, making them a versatile choice for complex energy needs. Let’s delve further into their mechanics, design intricacies, applications, operational specifics, historical evolution, modern significance, advantages, and challenges.
The mechanics of an automatic extraction condensing steam turbine are grounded in the Rankine cycle, optimized to extract maximum energy from steam while accommodating intermediate extraction points. A boiler generates steam by combusting fuels like coal, natural gas, oil, or biomass (e.g., wood pellets, rice husks, or sugarcane bagasse), or harnessing heat from nuclear fission, waste heat recovery (e.g., 400-600°C exhaust from kilns or engines), or concentrated solar power, producing steam at pressures of 50-300 bar and temperatures of 400-600°C, with supercritical or ultra-supercritical units reaching 700°C. This high-pressure steam enters the turbine, flowing over blades mounted on a rotor—a forged steel shaft that can weigh from a few kilograms in smaller units to 10-100 tons in large-scale systems. As the steam expands—say, from 100 bar to an extraction point at 15 bar, then to another at 5 bar, and finally to 0.05 bar—it accelerates to speeds of 500-700 meters per second, driving the blades and spinning the rotor at speeds such as 3,000 RPM for 50 Hz electrical grids, 3,600 RPM for 60 Hz, or up to 10,000 RPM in smaller, high-speed designs. The rotor powers a generator to produce electricity or drives machinery like pumps or compressors through a shaft, often with a gearbox to adjust speed—e.g., stepping down 10,000 RPM to 1,500 RPM for industrial equipment. Steam is extracted at intermediate stages—e.g., 15 bar for a chemical reactor, 5 bar for preheating boiler feedwater—via ports equipped with automatic valves, while the remaining steam exhausts into a condenser, where it cools back to water at 30-50°C under vacuum conditions using cooling water from rivers, oceans, or towers, or air in arid regions. This vacuum maximizes the pressure differential and power output, while the automation system—typically a programmable logic controller (PLC) or distributed control system (DCS)—uses sensors to monitor and adjust extraction flows in real time, ensuring a stable balance between power generation and steam supply as demands shift.
The design of automatic extraction condensing steam turbines is intricate, combining multi-stage expansion, extraction capabilities, and condensing technology. They feature 10-50 stages, organized into high-pressure (HP) sections before the first extraction, intermediate sections for each extraction point, and low-pressure (LP) sections leading to the condenser. HP stages manage the initial steam conditions—e.g., 100 bar, 600°C—with small blades (5-20 cm) crafted from nickel-based superalloys like Inconel 718 or Nimonic, designed to withstand extreme heat and pressure, often coated with chromium carbide or ceramic layers to resist creep, corrosion, and oxidation. Extraction stages handle mid-range pressures—e.g., 15 bar or 5 bar—with blades (10-30 cm) sized for controlled flow, while LP stages process the final expansion to 0.05 bar with massive blades (up to 1.5-2 meters), made of titanium or stainless steel to endure wet steam erosion at tip speeds of 500-700 m/s. Impulse designs are prevalent in HP stages—steam accelerates through nozzles into jets that strike bucket-shaped blades—while reaction designs dominate LP stages, where steam expands across moving blades, with combination turbines blending both approaches for optimal efficiency across the pressure gradient. Extraction ports, equipped with throttling valves or adjustable nozzles, divert steam to external piping, controlled by servo-motors linked to the automation system, which responds to real-time signals from pressure, temperature, or flow sensors. Rotors, weighing tons in large units, are precision-balanced to minimize vibration, while casings—forged from high-strength steel or alloys—contain pressures up to 300 bar, with labyrinth seals or brush seals reducing leakage across stages, a critical feature given the steep drop to vacuum. Condensers—typically shell-and-tube or surface designs—use 1-2 liters of cooling water per kWh or air fans to maintain the vacuum, rejecting 50-60% of heat as waste, supported by cooling towers that
Back Pressure Steam Turbine
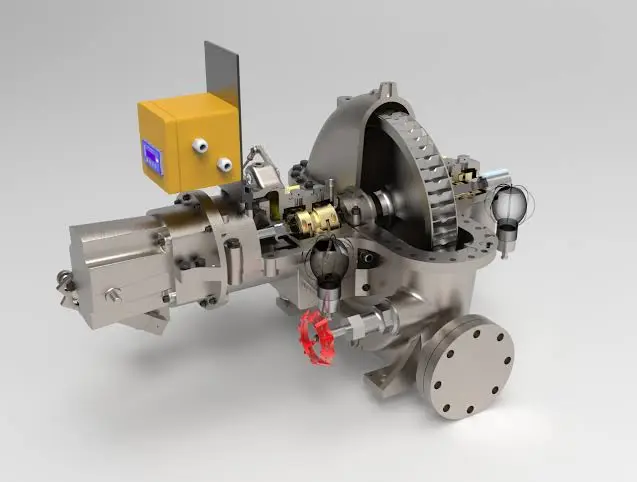
Here’s a detailed exploration of back-pressure steam turbines, presented as a long plain text. Back-pressure steam turbines, also known as non-condensing steam turbines, are a type of steam turbine where the exhaust steam exits at a pressure higher than atmospheric, typically between 2 and 20 bar, rather than being condensed into a vacuum as in condensing turbines. These turbines are designed to produce mechanical power—usually to drive a generator for electricity or to power machinery—while simultaneously supplying usable exhaust steam for industrial processes, heating, or other thermal applications. They are widely utilized in industrial settings such as pulp and paper mills, chemical plants, sugar mills, and combined heat and power (CHP) systems, with power outputs ranging from a few kilowatts to tens of megawatts. Back-pressure steam turbines are valued for their simplicity, high total energy efficiency in CHP applications, and ability to make dual use of steam as both a power source and a heat source. Let’s dive into their mechanics, design features, applications, operational details, historical context, modern relevance, advantages, and challenges.
The mechanics of a back-pressure steam turbine are based on the Rankine cycle, adapted to prioritize both mechanical power and usable exhaust steam over maximizing electrical efficiency alone. A boiler generates steam by burning fuels such as coal, natural gas, oil, or biomass (e.g., wood chips, bagasse, or rice husks), or by capturing waste heat from industrial processes—imagine exhaust from a furnace at 400-600°C or a gas engine at 300-400°C. Steam enters the turbine at pressures typically ranging from 10 to 100 bar and temperatures from 200 to 540°C, depending on the system’s scale and fuel source. This steam flows over blades mounted on a rotor—a forged steel shaft that can weigh from a few kilograms in small units to several tons in larger ones. As the steam expands—dropping from, say, 50 bar to an exhaust pressure of 5 bar—it accelerates to speeds of 300-500 meters per second, pushing the blades and spinning the rotor at speeds like 3,000 RPM for 50 Hz electrical grids, 3,600 RPM for 60 Hz, or up to 10,000 RPM in high-speed industrial designs. The rotor drives a generator to produce electricity or powers mechanical equipment such as pumps, compressors, fans, or mills through a shaft, often with a gearbox to adjust speed—for example, stepping down 10,000 RPM to 1,500 RPM for a pump. Unlike condensing turbines, which exhaust into a vacuum (0.05-0.1 bar) to extract maximum energy, back-pressure turbines release steam at a higher pressure—2 to 20 bar—determined by downstream thermal needs, such as heating a building, drying materials in a factory, or supplying steam for chemical reactions. This exhaust steam retains significant thermal energy, often 50-70% of the input, making back-pressure turbines ideal for CHP systems where the goal is to maximize total energy utilization rather than electrical output alone.
The design of back-pressure steam turbines reflects their dual-purpose role and simpler construction compared to condensing systems. They can be single-stage for small applications—say, a 50 kW unit with one set of blades extracting energy in a single pass—or multi-stage with 5 to 20 stages for larger outputs like 10 MW, allowing progressive energy extraction across multiple blade rows. Impulse designs are common, especially in smaller units—steam accelerates through nozzles into high-velocity jets that strike curved, bucket-shaped blades, spinning the rotor at high speeds like 10,000-20,000 RPM, a design rooted in Gustaf de Laval’s 1880s impulse turbine. Reaction designs, drawing from Charles Parsons’ multi-stage concept, are more typical in larger back-pressure turbines—steam expands across alternating fixed stator and moving rotor blades, spinning at 3,000-6,000 RPM for better efficiency and compatibility with standard generators. Combination designs may integrate impulse and reaction stages—impulse for the high-pressure entry (e.g., 50 bar) and reaction for the lower-pressure exhaust (e.g., 10 bar)—optimizing energy extraction in mid-sized systems. Blades vary in size—5-20 cm in high-pressure sections, up to 50 cm in low-pressure stages—made of stainless steel for durability or nickel-based alloys like Inconel for heat resistance up to 540°C, often coated with chromium or ceramic to resist corrosion and erosion from wet steam. Rotors, forged steel, are precision-balanced to handle high speeds without vibration, while casings—cast steel or alloys—contain pressures up to 100 bar, with seals like labyrinths or packing glands minimizing leakage, though sealing is less critical here than in condensing turbines since the exhaust pressure is higher than atmospheric, reducing the pressure differential that drives leaks.
Back-pressure steam turbine generators couple the turbine with an alternator or dynamo to produce electricity, scaled to the system’s needs. A 1 MW unit might generate 400 V AC for industrial machinery, while a 50 kW unit produces 230 V AC for a small facility, synchronized at 50 or 60 Hz for grid or local use. Generators are typically synchronous—rotor poles spin within stator windings—cooled by air or water to dissipate heat from 50-70% energy losses, with voltage adjustable via regulators to match loads. Boilers, producing 10 to 100 tons/hour of steam, integrate with diverse fuels—natural gas offers clean combustion with minimal emissions, biomass leverages local waste like sawdust or agricultural residues, and waste heat taps free energy from processes like steelmaking or cement production. Unlike condensing turbines, back-pressure systems eliminate the need for a condenser and associated cooling infrastructure—exhaust steam is piped directly to heat exchangers, process equipment, or heating systems, simplifying installation and maintenance compared to the complex cooling towers and vacuum pumps of condensing setups. Control systems range from manual valves and mechanical governors in basic configurations to digital controls with sensors in modern units, adjusting steam flow to balance power and heat output, with costs ranging from $10,000 for a 50 kW unit to $1-2 million for a 10 MW system, depending on size and sophistication.
Applications of back-pressure steam turbines are concentrated in industries and settings where both electrical power and thermal energy are required, making them a natural fit for CHP operations. Pulp and paper mills are a prime example—a 5 MW turbine burns wood waste from sawmill operations, producing 4 MW of electricity to run machinery like grinders and pumps, and 10 tons/hour of 5-bar steam to dry paper pulp, capitalizing on abundant biomass to offset grid costs by $1-2 million annually. Chemical plants use them for process steam—a 10 MW turbine generates 8 MW of power and 20 tons/hour of 15-bar steam for reactors, distillation columns, or heating vats, often fueled by natural gas for its consistent heat output. Sugar mills rely heavily on them with bagasse—a 3 MW turbine powers milling equipment and delivers 8 tons/hour of 10-bar steam to evaporate cane juice, using cane waste to achieve near-self-sufficiency during harvest season, reducing reliance on external energy sources. Textile factories employ smaller units—a 500 kW turbine produces 400 kW and 2 tons/hour of 3-bar steam for dyeing fabrics or heating dye vats, often coal-fired in regions where coal is cheap and accessible. District heating systems in colder climates adopt them—a 2 MW turbine generates 1.5 MW of electricity and heats a small town with 5-bar steam piped to radiators, burning local biomass like wood pellets for sustainability and cost savings. Small cogeneration plants use them for grid support—a 20 MW turbine supplies 15 MW to the grid and steam to nearby factories, fueled by oil or gas in urban settings where fuel supply is reliable. Off-grid applications benefit as well—a 50 kW turbine in a remote farm burns wood, powering tools and heating livestock barns with 2-bar steam, providing energy independence where extending the grid would cost tens of thousands of dollars per kilometer.
Efficiency in back-pressure steam turbines depends on their operational priorities, with a focus on total energy utilization rather than electrical output alone. Electrical efficiency is typically lower than that of condensing turbines—ranging from 20-30%—because the steam expansion stops at a higher exhaust pressure, leaving much of its energy as usable heat rather than converting it fully to mechanical work. A 5 MW turbine burning 20 tons/hour of steam (50 bar, 540°C) might produce 1.5 MW of electricity (25% efficiency), with 70% of the energy exiting as 10-bar steam (3.5 MW thermal equivalent), and 5-10% lost to friction, blade turbulence, and leakage. In CHP mode, total efficiency—combining power and heat—reaches 70-85%, far surpassing the 35-45% electrical efficiency of condensing systems, making them highly efficient when both outputs are utilized. For instance, a 3 MW turbine might convert 25% to electricity (750 kW), 55% to 5-bar steam (1.65 MW thermal), and lose 20% to waste heat—a stark contrast to condensing turbines, which reject 50-60% of energy as unusable heat. Losses in back-pressure turbines come from incomplete expansion (exhaust at 5-20 bar vs. 0.05 bar in condensing units), blade inefficiencies due to fewer stages compared to large turbines, and leakage (5-10% with basic seals). Multi-stage designs with 5-20 stages or superheating steam to 540°C can boost electrical efficiency to 30-35%, but lowering the exhaust pressure (e.g., to 2 bar) increases power output at the expense of usable heat, a trade-off that CHP systems carefully manage to meet thermal demands. Fuel quality significantly impacts performance—dry biomass at 4.5 kWh/kg outperforms wet biomass at 3 kWh/kg, with moisture levels above 20% reducing steam output by 30% if not pre-dried, a common challenge in biomass-heavy applications like sugar mills or sawmills.
The historical development of back-pressure steam turbines traces back to the early days of steam power, evolving from the need to utilize steam efficiently in industrial settings. Charles Parsons’ 1884 multi-stage turbine and Gustaf de Laval’s impulse design provided the foundational technology, but by the early 20th century, industries adopted back-pressure turbines for their ability to supply both power and heat, outpacing less efficient steam engines (5-10% efficiency) with turbines offering 15-25% electrical efficiency plus usable steam. Pre-World War II factories used coal-fired units to drive machinery and heat workspaces, scaling to megawatts by the 1930s as metallurgy improved—chromium steels allowed higher temperatures and pressures, increasing durability and performance. During WWII, back-pressure turbines powered small plants or military facilities—e.g., a 1 MW unit ran a factory and warmed it using rationed fuel, proving their resilience in resource-scarce conditions. Post-war, condensing turbines took over large-scale power generation due to their higher electrical efficiency, but the 1970s energy crisis revived back-pressure designs for CHP applications, particularly with the rise of biomass and waste heat as alternative fuels amid soaring oil prices. Modern units enhance this legacy—digital automation optimizes steam use, and advanced materials like nickel alloys withstand temperatures up to 540°C, though they remain a specialized tool compared to the widespread use of condensing systems in utility-scale power plants.
The modern significance of back-pressure steam turbines lies in their role in energy efficiency and sustainability, particularly in industrial and decentralized energy systems. Industrial CHP applications thrive with these turbines—a 10 MW turbine saves a factory $2-3 million annually by reusing exhaust steam for processes like drying or heating, far more cost-effective than separate power generation (30-40% efficiency) and heating systems (50-60% fuel loss), which can double operational costs. Biomass utilization is on the rise—a 5 MW turbine burns 2 tons/hour of wood waste, reducing fossil fuel dependence in rural industries like sawmills or paper plants, aligning with carbon reduction goals such as those set by international agreements like the Paris Accord. Waste heat recovery expands their reach—a 2 MW turbine uses 400°C exhaust from a steel furnace, generating power and steam without additional fuel, cutting emissions and costs by $500,000 yearly in industries with high heat output. District heating systems in Europe and North America rely on them—a 3 MW turbine heats 500 homes and powers local loads with 5-bar steam, often fueled by gas or biomass for reliability and sustainability, providing a cost-effective alternative to separate electric and heating grids. Decentralized power applications grow too—a 50-500 kW turbine serves off-grid communities or farms where grid access costs exceed $10,000 per kilometer, offering a self-sufficient energy solution. Innovations bolster their role—digital controls with real-time sensors balance power and heat output, advanced coatings handle higher temperatures, and modular designs reduce installation costs, though condensing turbines dominate in scenarios focused solely on large-scale electricity production.
The advantages of back-pressure steam turbines are numerous and context-specific, making them a compelling choice for certain applications. Their simplicity—no need for a condenser or cooling towers—reduces initial costs to $500-$1,000 per kW, compared to $1,500-$2,000/kW for condensing systems, and eliminates the maintenance burden of complex cooling infrastructure like vacuum pumps or cooling water treatment systems. High CHP efficiency (70-85%) maximizes energy utilization—a 5 MW turbine delivers 4 MW of combined electrical and thermal output, outperforming standalone generators or boilers in total energy efficiency, especially when exhaust steam is fully utilized. Fuel flexibility accommodates a wide range of local resources—wood, agricultural waste, gas, or waste heat work effectively, making them viable in diverse settings like forested regions, agricultural zones, or industrial hubs with excess heat. Their compact size fits constrained spaces—a 1 MW unit requires a 5×10-meter footprint versus 10×20 meters for a condensing setup, easing installation in factories or small plants where space is at a premium. Reliability is robust—units can run for 20-30 years with regular maintenance, operating 5,000-8,000 hours/year in harsh conditions like dusty mills or humid climates, with fewer components prone to failure compared to condensing systems. Sustainability aligns with green initiatives—biomass-driven units achieve near carbon neutrality when paired with regrowth cycles, and waste heat recovery minimizes fuel use, supporting eco-friendly operations in industries aiming to reduce their carbon footprint.
Challenges, however, limit their broader adoption and must be carefully considered. Their lower electrical efficiency (20-30%) restricts power output compared to condensing turbines—a 5 MW turbine yields 1.5 MW of electricity versus 2 MW from a condensing turbine with the same steam input, requiring more fuel (e.g., 2 tons/hour of wood versus 1.5 tons) to achieve equivalent electrical generation, which can increase operational costs if heat isn’t fully utilized. The trade-off between exhaust pressure and power constrains design flexibility—higher exhaust pressures (10-20 bar) preserve more heat for thermal use but reduce electrical yield, while lower pressures (2-5 bar) boost power at the cost of thermal utility, requiring precise calibration to meet specific site needs. Maintenance needs—blade wear from wet steam, seal leakage, and boiler scaling—cost $10,000-$50,000 annually for a 5 MW unit, with downtime interrupting power or heat supply, a significant concern in continuous operations like chemical plants or paper mills. Emissions from biomass fuels—particulates at 50-200 mg/m³ and CO—require filters or scrubbers costing $10,000-$50,000 to meet stringent regulations like the U.S. EPA’s 25 mg/m³ limit or the EU’s 20 mg/m³ standard, adding expense and complexity to biomass-driven systems. Initial costs, while lower than condensing systems, remain significant—$500,000 for a 1 MW unit can’t compete with solar’s $1-2/W for small-scale power generation, making them less attractive for pure electricity needs. Fuel logistics pose additional risks—wet biomass (e.g., 50% moisture) or inconsistent waste heat reduces steam quality, cutting output by 20-30% if not managed with drying systems or heat buffers, a practical challenge in regions with variable fuel availability.
Examples showcase their practical impact across various scales and industries. A 1 MW back-pressure turbine in a textile plant burns coal, producing 800 kW of electricity and 3 tons/hour of 5-bar steam for dyeing processes, saving $200,000 annually on energy bills with a $400,000 setup cost, paying back in two years. A 10 MW turbine in a paper mill uses wood waste, generating 8 MW of power and 20 tons/hour of 10-bar steam for drying pulp, reducing operational costs by $2 million yearly against a $2 million investment, leveraging free biomass from mill operations. A 50 kW off-grid turbine in a rural farm burns logs, powering tools with 40 kW and heating a barn with 2-bar steam, costing $10,000 to install but saving $5,000 annually over diesel generators, ideal where fuelwood is abundant and grid access is impractical.
Back-pressure steam turbines excel in environments where power and heat are both valuable, offering a streamlined, efficient solution for industries, small plants, and off-grid sites. Their evolution—from early 20th-century industrial applications to modern CHP systems—reflects a focus on resource efficiency and adaptability, ensuring they remain a relevant and practical choice in a world increasingly balancing energy needs with sustainability goals. Their ability to turn steam into a dual-purpose resource keeps them a vital tool in the energy landscape, particularly where local fuels or waste heat can be harnessed effectively.
Here’s a continued exploration of back-pressure steam turbines, presented as a detailed plain text that builds on the previous discussion with deeper insights and expanded perspectives. Back-pressure steam turbines, also referred to as non-condensing steam turbines, are engineered to generate mechanical power—typically for electricity production or driving machinery—while exhausting steam at a pressure above atmospheric levels, usually between 2 and 20 bar, making the exhaust steam usable for industrial processes, heating, or other thermal applications. Unlike condensing turbines, which exhaust into a vacuum to maximize electrical output, back-pressure turbines prioritize a balance between power generation and thermal energy delivery, making them ideal for combined heat and power (CHP) systems and industrial settings like sugar mills, pulp and paper plants, and chemical facilities. With capacities ranging from a few kilowatts to tens of megawatts, they offer simplicity, high total energy efficiency when paired with heat use, and versatility with various fuel sources. Let’s delve further into their mechanics, design intricacies, applications, operational specifics, historical evolution, modern significance, advantages, and challenges.
The mechanics of a back-pressure steam turbine follow the Rankine cycle, tailored to provide both mechanical work and usable exhaust steam rather than focusing solely on electrical efficiency. A boiler produces steam by combusting fuels such as coal, natural gas, oil, or biomass (e.g., wood pellets, sawdust, or agricultural residues like corn stalks), or by capturing waste heat from sources like industrial exhausts at 400-600°C or engine tailpipes at 300-400°C. Steam enters the turbine at pressures typically between 10 and 100 bar and temperatures from 200 to 540°C, depending on the system’s size and fuel quality. This steam flows over blades mounted on a rotor—a forged steel shaft that ranges from a few kilograms in small units to several tons in larger ones. As the steam expands—say, from 50 bar to an exhaust pressure of 5 bar—it accelerates to speeds of 300-500 meters per second, driving the blades and spinning the rotor at speeds like 3,000 RPM for 50 Hz grids, 3,600 RPM for 60 Hz, or up to 10,000 RPM in high-speed configurations. The rotor powers a generator to produce electricity or drives machinery such as pumps, fans, compressors, or mills via a shaft, often with a gearbox to adjust speed—for example, reducing 10,000 RPM to 1,500 RPM for a pump. Unlike condensing turbines, which exhaust into a vacuum (0.05-0.1 bar) to extract maximum energy, back-pressure turbines release steam at a higher pressure—2 to 20 bar—set by downstream thermal requirements, such as heating a factory, drying lumber, or supplying steam for chemical distillation. This exhaust steam retains substantial thermal energy, often 50-70% of the input, making back-pressure turbines highly effective in CHP setups where the total energy utilization of both power and heat is the primary objective.
The design of back-pressure steam turbines is practical, emphasizing dual-purpose energy production and reduced complexity compared to condensing systems. They can be single-stage for small-scale use—such as a 50 kW unit with one blade set extracting energy in a single pass—or multi-stage with 5 to 20 stages for larger capacities like 10 MW, allowing gradual energy extraction across multiple blade rows. Impulse designs are prevalent in smaller units—steam accelerates through nozzles into high-velocity jets that strike curved, bucket-shaped blades, spinning the rotor at high speeds like 10,000-20,000 RPM, echoing Gustaf de Laval’s 1880s impulse turbine. Reaction designs, inspired by Charles Parsons’ multi-stage approach, are more common in larger back-pressure turbines—steam expands across alternating fixed stator and moving rotor blades, spinning at 3,000-6,000 RPM for improved efficiency and compatibility with standard generators. Combination designs may blend impulse and reaction stages—impulse for the high-pressure entry (e.g., 50 bar) and reaction for the lower-pressure exhaust (e.g., 10 bar)—optimizing performance in mid-sized systems. Blades are sized for the pressure range—5-20 cm in high-pressure sections, up to 50 cm in low-pressure stages—crafted from stainless steel for cost-effectiveness and corrosion resistance or nickel-based alloys like Inconel for heat tolerance up to 540°C, often coated with chromium or ceramic to combat erosion from wet steam. Rotors, forged steel, are precision-balanced to handle high speeds without vibration, while casings—cast steel or alloys—contain pressures up to 100 bar, with seals like labyrinths or packing glands minimizing leakage, though sealing is less critical here than in condensing turbines due to the higher exhaust pressure reducing the pressure differential that drives leaks.
Back-pressure steam turbine generators pair the turbine with a synchronous generator or alternator to produce electricity, tailored to the system’s scale. A 1 MW unit might generate 400 V AC for industrial machinery, while a 50 kW unit produces 230 V AC for smaller loads, synchronized at 50 or 60 Hz for grid or local use. Generators feature rotor poles spinning within stator windings, cooled by air in smaller units or water in larger ones to dissipate heat from 50-70% energy losses, with voltage regulators adjusting output to match demand. Boilers, producing 10 to 100 tons/hour of steam, integrate with a variety of fuels—natural gas provides clean, efficient combustion, biomass utilizes local waste like wood chips or straw, and waste heat taps free energy from processes such as glass manufacturing or cement kilns. Unlike condensing turbines, back-pressure systems skip the condenser and cooling towers—exhaust steam is piped directly to heat exchangers, process equipment, or heating systems, simplifying the setup and reducing the footprint and maintenance compared to the extensive cooling infrastructure of condensing turbines. Control systems vary—manual valves and mechanical governors suffice in basic setups, while modern units use digital controls with sensors to adjust steam flow, optimizing the balance between power and heat output, with costs ranging from $10,000 for a 50 kW unit to $1-2 million for a 10 MW system, depending on capacity and features.
Applications of back-pressure steam turbines are centered in industries and settings where both electrical power and thermal energy are in demand, making them a cornerstone of CHP operations. Pulp and paper mills frequently use them—a 5 MW turbine burns wood waste from sawmill operations, producing 4 MW of electricity to power grinders and conveyors, and 10 tons/hour of 5-bar steam to dry paper pulp, leveraging abundant biomass to save $1-2 million annually on grid electricity and heating costs. Chemical plants rely on them for process steam—a 10 MW turbine generates 8 MW of power and 20 tons/hour of 15-bar steam for reactors, distillation units, or heating, often fueled by natural gas for its reliability and low emissions. Sugar mills depend on them with bagasse—a 3 MW turbine powers milling equipment and supplies 8 tons/hour of 10-bar steam to evaporate cane juice, using cane waste to achieve near energy self-sufficiency during harvest season, minimizing reliance on external fuel sources. Textile factories use smaller units—a 500 kW turbine produces 400 kW and 2 tons/hour of 3-bar steam for dyeing or heating dye vats, often coal-fired in regions with affordable coal supplies. District heating systems in colder climates adopt them—a 2 MW turbine generates 1.5 MW of electricity and heats a small town with 5-bar steam piped to radiators, burning local biomass like wood pellets for cost-effective sustainability. Small cogeneration plants use them for grid support—a 20 MW turbine supplies 15 MW to the grid and steam to nearby factories, fueled by oil or gas in urban areas with reliable fuel access. Off-grid applications benefit too—a 50 kW turbine in a remote farm burns wood, powering tools and heating barns with 2-bar steam, offering energy independence where grid extension costs are prohibitive.
Efficiency in back-pressure steam turbines hinges on their dual-purpose operation, with a focus on total energy utilization rather than electrical output alone. Electrical efficiency is typically 20-30%, lower than condensing turbines (35-45%) because the steam expansion stops at a higher exhaust pressure, preserving energy for thermal use rather than converting it fully to mechanical work. A 5 MW turbine burning 20 tons/hour of steam (50 bar, 540°C) might produce 1.5 MW of electricity (25% efficiency), with 70% of the energy exiting as 10-bar steam (3.5 MW thermal equivalent), and 5-10% lost to friction, blade turbulence, and leakage. In CHP mode, total efficiency—combining power and heat—reaches 70-85%, significantly outperforming the 35-45% electrical efficiency of condensing systems when exhaust steam is fully utilized. For example, a 3 MW turbine might convert 25% to electricity (750 kW), 55% to 5-bar steam (1.65 MW thermal), and lose 20% to waste heat—a stark contrast to condensing turbines, which discard 50-60% of energy as unusable heat. Losses in back-pressure turbines arise from incomplete expansion (exhaust at 5-20 bar vs. 0.05 bar in condensing units), blade inefficiencies due to fewer stages than large condensing turbines, and leakage (5-10% with basic seals). Multi-stage designs with 5-20 stages or superheating steam to 540°C can increase electrical efficiency to 30-35%, but lowering the exhaust pressure (e.g., to 2 bar) boosts power at the expense of usable heat, a trade-off that CHP systems calibrate to match thermal demands. Fuel quality plays a critical role—dry biomass at 4.5 kWh/kg outperforms wet biomass at 3 kWh/kg, with moisture levels above 20% reducing steam output by 30% if not pre-dried, a frequent challenge in biomass-driven systems like sugar mills or rural installations.
The historical evolution of back-pressure steam turbines traces back to the early 20th century, driven by industrial needs for efficient steam use. Charles Parsons’ 1884 multi-stage turbine and Gustaf de Laval’s impulse design laid the groundwork, but by the 1920s, industries adopted back-pressure turbines for their ability to supply both power and heat, surpassing steam engines (5-10% efficiency) with turbines offering 15-25% electrical efficiency plus usable steam. Pre-World War II factories used coal-fired units to drive machinery and heat workspaces, scaling to megawatts by the 1930s as metallurgy advanced—chromium steels enabled higher temperatures and pressures, improving durability and output. During WWII, back-pressure turbines powered small plants or military facilities—e.g., a 1 MW unit ran a factory and heated it on limited fuel, showcasing their adaptability. Post-war, condensing turbines dominated large-scale power generation due to their higher electrical efficiency, but the 1970s energy crisis revived back-pressure designs for CHP, particularly with biomass and waste heat as oil prices soared, prompting a shift toward energy conservation. Modern units build on this legacy—digital automation enhances steam management, and materials like nickel alloys withstand 540°C, refining their role in industrial and sustainable energy systems, though they remain niche compared to condensing turbines in utility-scale applications.
The modern significance of back-pressure steam turbines lies in their efficiency and adaptability in industrial and decentralized energy contexts. Industrial CHP systems benefit greatly—a 10 MW turbine saves a factory $2-3 million annually by reusing exhaust steam for processes like drying or heating, far more economical than separate power generation (30-40% efficiency) and heating systems (50-60% fuel loss), which can inflate costs by 50-100%. Biomass utilization grows—a 5 MW turbine burns 2 tons/hour of wood waste, reducing fossil fuel reliance in rural industries like sawmills or paper plants, aligning with carbon neutrality goals and policies like the EU’s Green Deal. Waste heat recovery expands their scope—a 2 MW turbine uses 400°C exhaust from a glass furnace, generating power and steam without additional fuel, cutting emissions and costs by $500,000 yearly in heat-intensive sectors. District heating in colder regions leans on them—a 3 MW turbine heats 500 homes and powers local loads with 5-bar steam, often fueled by gas or biomass for reliability and eco-friendliness, offering a cost-effective alternative to separate grids. Decentralized power applications rise too—a 50-500 kW turbine serves off-grid communities or farms where grid extension costs exceed $10,000 per kilometer, providing self-sufficient energy. Innovations enhance their utility—digital controls with real-time sensors optimize power and heat balance, advanced coatings handle higher temperatures, and modular designs cut installation costs, though condensing turbines lead in pure power generation scenarios.
The advantages of back-pressure steam turbines are compelling for their specific applications. Their simplicity—no condenser or cooling towers—lowers initial costs to $500-$1,000 per kW versus $1,500-$2,000/kW for condensing systems, and reduces maintenance by avoiding complex cooling infrastructure like vacuum pumps or water treatment plants. High CHP efficiency (70-85%) maximizes energy use—a 5 MW turbine delivers 4 MW of combined electrical and thermal output, outperforming standalone generators or boilers when exhaust steam is fully utilized. Fuel flexibility accommodates diverse resources—wood, agricultural waste, gas, or waste heat work seamlessly, making them viable in forested areas, farming regions, or industrial zones with excess heat. Their compact size suits tight spaces—a 1 MW unit requires a 5×10-meter footprint versus 10×20 meters for a condensing setup, easing installation in factories or small plants. Reliability is strong—units run 20-30 years with regular maintenance, operating 5,000-8,000 hours/year in tough conditions like dusty mills or humid climates, with fewer failure-prone components than condensing systems. Sustainability aligns with green trends—biomass units offset CO2 with regrowth, and waste heat recovery cuts fuel use, appealing to eco-conscious operations.
Challenges limit their broader adoption. Lower electrical efficiency (20-30%) caps power output—a 5 MW turbine yields 1.5 MW versus 2 MW from a condensing turbine with the same input, needing more fuel (e.g., 2 tons/hour of wood vs. 1.5 tons) for equivalent electricity, raising costs if heat isn’t fully used. Exhaust pressure trade-offs constrain design—higher pressures (10-20 bar) preserve heat but reduce power, while lower pressures (2-5 bar) boost power at heat’s expense, requiring precise tuning. Maintenance—blade wear, seal leaks, boiler scaling—costs $10,000-$50,000 yearly for a 5 MW unit, with downtime disrupting output, a concern in continuous processes. Biomass emissions (50-200 mg/m³ particulates) need filters ($10,000-$50,000) to meet limits like the EU’s 20 mg/m³, adding cost. Initial costs—$500,000 for 1 MW—lag solar ($1-2/W) for small-scale power. Fuel inconsistency—wet biomass or variable heat—cuts output by 20-30% without mitigation.
Examples highlight their value. A 1 MW textile turbine burns coal, produces 800 kW and 3 tons/hour of 5-bar steam, saving $200,000 yearly. A 10 MW paper mill turbine uses wood waste, generates 8 MW and 20 tons/hour of 10-bar steam, saving $2 million. A 50 kW farm turbine burns logs, powers 40 kW and heats with 2-bar steam, saving $5,000 annually.
Back-pressure steam turbines shine where power and heat converge, their efficiency and adaptability making them vital for industries and CHP, blending steam’s legacy with modern energy needs.
EMS Power Machines
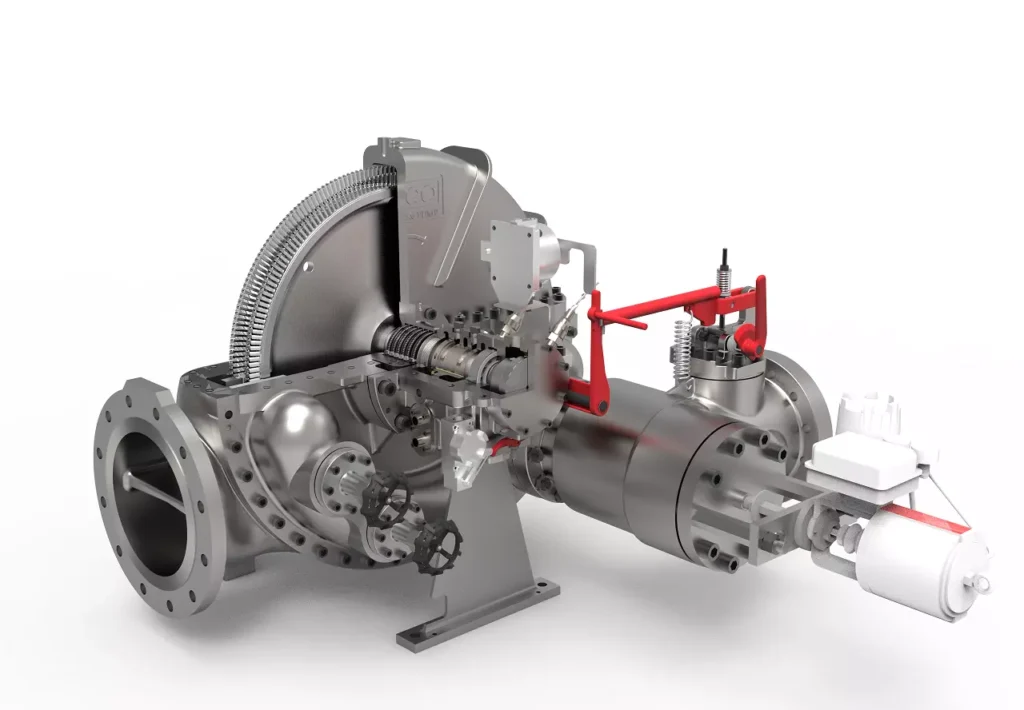
We design, manufacture and assembly Power Machines such as – diesel generators, electric motors, vibration motors, pumps, steam engines and steam turbines
EMS Power Machines is a global power engineering company, one of the five world leaders in the industry in terms of installed equipment. The companies included in the company have been operating in the energy market for more than 60 years.
EMS Power Machines manufactures steam turbines, gas turbines, hydroelectric turbines, generators, and other power equipment for thermal, nuclear, and hydroelectric power plants, as well as for various industries, transport, and marine energy.
EMS Power Machines is a major player in the global power industry, and its equipment is used in power plants all over the world. The company has a strong track record of innovation, and it is constantly developing new and improved technologies.
Here are some examples of Power Machines’ products and services:
- Steam turbines for thermal and nuclear power plants
- Gas turbines for combined cycle power plants and industrial applications
- Hydroelectric turbines for hydroelectric power plants
- Generators for all types of power plants
- Boilers for thermal power plants
- Condensers for thermal power plants
- Reheaters for thermal power plants
- Air preheaters for thermal power plants
- Feedwater pumps for thermal power plants
- Control systems for power plants
- Maintenance and repair services for power plants
EMS Power Machines is committed to providing its customers with high-quality products and services. The company has a strong reputation for reliability and innovation. Power Machines is a leading provider of power equipment and services, and it plays a vital role in the global power industry.
EMS Power Machines, which began in 1961 as a small factory of electric motors, has become a leading global supplier of electronic products for different segments. The search for excellence has resulted in the diversification of the business, adding to the electric motors products which provide from power generation to more efficient means of use.