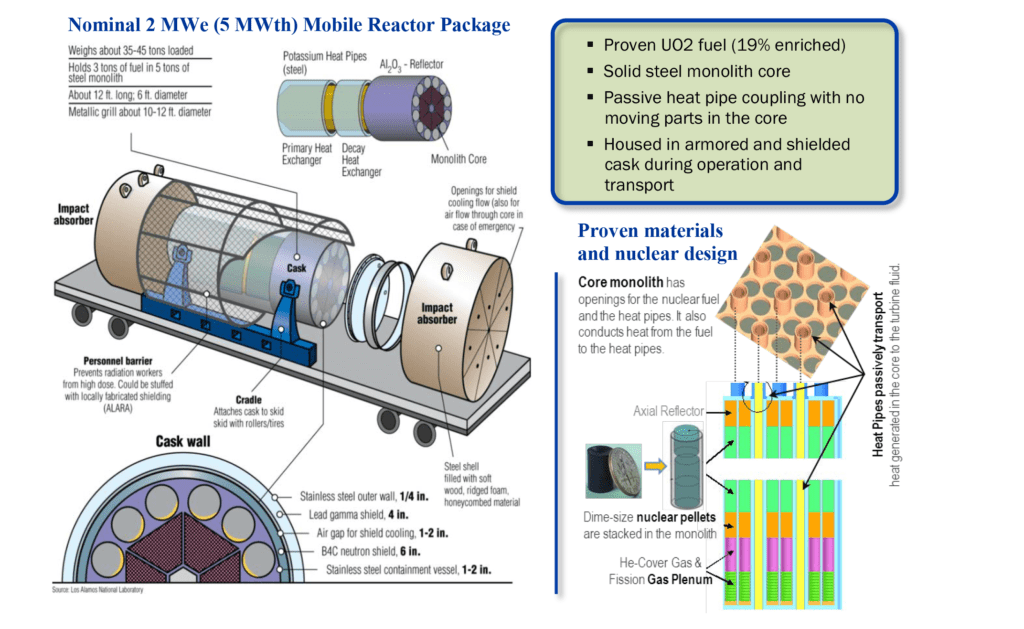
Micro Nuclear Plants Power Remote Areas: Electricity generation using nuclear energy is a process that involves harnessing the heat produced by nuclear fission reactions to generate steam, which then drives a turbine connected to an electrical generator. Here’s an overview of the key components and working principles of a nuclear power plant:
Basic Working Principle
- Nuclear Fission:
- Atoms of a fissile material (such as Uranium-235 or Plutonium-239) undergo fission, splitting into smaller nuclei and releasing a large amount of heat.
- This reaction is controlled within a nuclear reactor.
- Heat Transfer:
- The heat from fission is used to produce high-temperature steam by heating a coolant, typically water or gas.
- In pressurized water reactors (PWRs), the water is kept under high pressure to prevent boiling, while in boiling water reactors (BWRs), water turns directly into steam.
- Steam Generation:
- The steam is directed toward steam turbines, causing them to rotate.
- Electricity Generation:
- The rotating turbine drives a generator, which converts mechanical energy into electricity through electromagnetic induction.
- Cooling & Condensation:
- After passing through the turbine, the steam is cooled down using a condenser, turning it back into liquid water.
- This cooling is often done using a nearby river, lake, or cooling tower.
- Recycling & Reuse:
- The condensed water is pumped back to the reactor to continue the cycle.
Types of Nuclear Reactors
There are several types of reactors used in nuclear power plants:
- Pressurized Water Reactor (PWR) – Uses high-pressure water as a coolant; most common type.
- Boiling Water Reactor (BWR) – Water is boiled directly in the reactor to produce steam.
- Pressurized Heavy Water Reactor (PHWR) – Uses heavy water as both coolant and moderator.
- Gas-cooled Reactor (GCR) – Uses carbon dioxide or helium as a coolant.
- Fast Breeder Reactor (FBR) – Uses fast neutrons and can produce more fissile material than it consumes.
Advantages of Nuclear Power
✔ High Energy Density – A small amount of nuclear fuel generates a large amount of electricity.
✔ Low Carbon Emissions – Unlike fossil fuels, nuclear power does not release CO₂ during operation.
✔ Reliable & Continuous Power Supply – Unlike solar and wind, nuclear plants provide consistent baseload power.
✔ Long Fuel Life – Fuel rods last for several years before requiring replacement.
Challenges & Disadvantages
❌ Radioactive Waste – Spent nuclear fuel remains hazardous for thousands of years and requires safe disposal.
❌ High Initial Cost – Building a nuclear plant requires huge investments in safety and technology.
❌ Nuclear Accidents – Events like Chernobyl (1986) and Fukushima (2011) show the risks of radiation leaks.
❌ Limited Fuel Supply – Uranium reserves are finite, though breeder reactors can extend fuel availability.
Future of Nuclear Energy
- Small Modular Reactors (SMRs) – More compact, safer, and easier to deploy.
- Fusion Power – Future reactors (e.g., ITER) aim to use nuclear fusion, the same process as the Sun, for safer and nearly limitless energy.
- Advanced Reactors – Generation IV reactors focus on higher efficiency, safety, and waste reduction.
Electricity generation with nuclear energy begins with nuclear fission, where atoms of a fissile material such as Uranium-235 split into smaller nuclei, releasing a large amount of heat. This reaction occurs in a nuclear reactor, where the energy is controlled and sustained. The heat generated is used to convert water into steam, which is then directed to a steam turbine. The high-pressure steam causes the turbine blades to rotate, which in turn drives a generator that produces electricity through electromagnetic induction. After passing through the turbine, the steam is cooled and condensed back into liquid form using a condenser, often with the help of cooling water from a nearby river, lake, or cooling tower. The condensed water is then pumped back to the reactor to continue the cycle, ensuring a continuous supply of steam for power generation. This entire process enables nuclear power plants to generate electricity efficiently while producing minimal greenhouse gas emissions.
Electricity generation using nuclear energy relies on the process of nuclear fission, where the nucleus of a heavy atom, such as Uranium-235 or Plutonium-239, splits into smaller nuclei when struck by a neutron. This reaction releases a tremendous amount of heat energy along with additional neutrons, which can sustain a chain reaction when properly controlled within a nuclear reactor. The fission process is carefully managed using control rods, typically made of materials like boron or cadmium, which absorb excess neutrons to regulate the rate of the reaction and prevent overheating or runaway reactions.
The heat produced in the reactor is transferred to a coolant, which is usually water, gas, or liquid metal, depending on the type of reactor. In the case of pressurized water reactors (PWRs), which are the most common type, water is kept under high pressure to prevent it from boiling inside the reactor. The heated coolant then passes through a heat exchanger or steam generator, where it transfers its thermal energy to a separate water loop, turning the water into high-pressure steam. In boiling water reactors (BWRs), the water is allowed to boil directly within the reactor, producing steam without the need for a heat exchanger.
The high-pressure steam is then directed toward a steam turbine, where it expands and pushes the turbine blades, causing them to spin at high speeds. The turbine is mechanically connected to a generator, which consists of a rotating magnet surrounded by a stationary coil of wire. As the turbine spins the generator, it induces an electric current, converting the mechanical energy of the turbine into electrical energy that can be distributed through the power grid.
After passing through the turbine, the steam must be cooled and converted back into liquid form for reuse. This is achieved in a condenser, where the steam is exposed to cool water from an external source, such as a river, lake, or cooling tower. The cooling process allows the steam to condense back into liquid water, which is then pumped back into the system to be reheated in the reactor, ensuring a continuous cycle of energy production. The cooling water itself, after absorbing heat from the steam, is either recirculated or safely released back into the environment at a controlled temperature to minimize ecological impact.
Nuclear power plants are designed to operate with high efficiency and reliability, providing baseload power—a steady and continuous supply of electricity that is not affected by fluctuations in weather or fuel availability. Unlike fossil fuel power plants, nuclear reactors do not produce carbon dioxide or other greenhouse gases during operation, making them a low-emission energy source. However, the process does generate radioactive waste, including spent nuclear fuel, which must be carefully stored and managed to prevent environmental contamination and radiation exposure.
Despite challenges such as high initial construction costs, concerns over nuclear safety, and the need for long-term waste disposal solutions, nuclear energy remains a key part of global electricity generation. Ongoing research into advanced reactor designs, small modular reactors (SMRs), and nuclear fusion technology aims to improve safety, efficiency, and sustainability, making nuclear power a potential long-term solution for clean and reliable energy production.
The role of nuclear energy in electricity generation continues to evolve as advancements in technology aim to improve efficiency, safety, and sustainability. Traditional nuclear power plants primarily use light water reactors (LWRs), including pressurized water reactors (PWRs) and boiling water reactors (BWRs), which have been the dominant technology for decades. However, newer reactor designs, such as Generation IV reactors and small modular reactors (SMRs), are being developed to address challenges such as waste disposal, fuel efficiency, and safety concerns.
One of the key concerns in nuclear energy production is the management of radioactive waste, particularly spent nuclear fuel. After a fuel rod has been used in a reactor, it remains highly radioactive and must be carefully handled. Initially, spent fuel is stored in cooling pools within the power plant, where water acts as both a coolant and a radiation shield. After several years, once heat and radiation levels have decreased, the fuel can be transferred to dry cask storage, where it is sealed in reinforced concrete and steel containers for long-term safekeeping. Some countries, such as France and Russia, use nuclear fuel reprocessing to extract usable fissile material from spent fuel, reducing waste and increasing fuel efficiency. However, reprocessing is expensive and raises proliferation concerns, as it involves the separation of plutonium, which could be used in nuclear weapons.
Another critical aspect of nuclear power generation is safety. While nuclear reactors are designed with multiple layers of safety systems, past accidents—such as Chernobyl (1986), Fukushima (2011), and Three Mile Island (1979)—have highlighted potential risks associated with reactor operation. Modern reactors incorporate advanced passive safety features, which rely on natural physical processes rather than active mechanical systems to maintain cooling and containment in the event of an emergency. For example, some advanced reactors are designed with molten salt coolant or helium gas cooling, which are less likely to lead to catastrophic failures compared to traditional water-based cooling systems.
In addition to traditional nuclear fission, significant research efforts are being directed toward nuclear fusion, which has the potential to provide virtually limitless energy with minimal radioactive waste. Unlike fission, which splits heavy atomic nuclei, fusion involves combining light nuclei—such as hydrogen isotopes (deuterium and tritium)—to form helium, releasing vast amounts of energy in the process. The International Thermonuclear Experimental Reactor (ITER) in France is one of the largest fusion research projects in the world, aiming to demonstrate the feasibility of fusion as a large-scale energy source. If successful, fusion reactors could revolutionize energy production by providing a safer, cleaner, and more abundant alternative to conventional nuclear power.
Despite challenges, nuclear energy remains a crucial part of the global energy mix, providing a stable and low-carbon alternative to fossil fuels. Countries such as France, the United States, China, and Russia continue to invest in nuclear power as a key strategy for reducing greenhouse gas emissions and achieving energy security. The future of nuclear energy will likely be shaped by a combination of advanced fission reactors, small modular reactors (SMRs), and potential breakthroughs in fusion technology, offering cleaner, safer, and more efficient ways to generate electricity.
The continued advancement of nuclear energy technology is shaping its role in the future of global electricity generation. With growing concerns over climate change, energy security, and the need for reliable baseload power, many countries are reassessing and expanding their nuclear energy programs. The development of advanced reactors, improvements in nuclear fuel cycles, and the exploration of alternative reactor designs are all part of a broader effort to make nuclear energy safer, more efficient, and more sustainable.
One of the most promising innovations in nuclear energy is the development of Small Modular Reactors (SMRs). Unlike traditional large-scale nuclear power plants, SMRs are designed to be smaller, modular, and more flexible, making them easier to deploy in remote locations, industrial facilities, or areas with smaller energy demands. These reactors typically produce less than 300 megawatts (MW) of electricity, compared to conventional reactors that generate over 1,000 MW. SMRs are also designed with passive safety features, meaning they rely on natural physical processes like gravity, convection, and heat dissipation rather than active mechanical systems, reducing the risk of accidents. Countries like the United States, Canada, Russia, and China are leading efforts to commercialize SMRs, with several designs currently in development and testing.
In parallel with SMRs, Generation IV reactors are being designed to address challenges related to fuel efficiency, safety, and waste management. These next-generation reactors include fast neutron reactors (FNRs), molten salt reactors (MSRs), gas-cooled reactors, and lead-cooled reactors. A key advantage of these reactors is their ability to operate at higher temperatures and utilize breeder reactor technology, which can generate more fissile material than they consume. This process significantly improves fuel utilization and reduces the amount of long-lived nuclear waste. Some Generation IV reactors are also capable of using thorium as a fuel source, an element that is more abundant than uranium and produces less long-lived radioactive waste. Countries such as India and China are actively exploring thorium-based nuclear reactors as a future energy solution.
Beyond traditional fission, the pursuit of nuclear fusion remains one of the most exciting frontiers in energy research. Fusion offers the promise of nearly limitless clean energy without the risks associated with radioactive waste or nuclear accidents. Unlike fission, which splits heavy atoms, fusion joins light atomic nuclei—such as deuterium and tritium—to release energy. The challenge, however, is achieving the extremely high temperatures and pressures required to sustain a controlled fusion reaction. The ITER (International Thermonuclear Experimental Reactor) project, based in France, is currently the world’s largest fusion research effort. It aims to demonstrate the feasibility of fusion power by the 2030s. In addition to ITER, private companies and national research programs are working on alternative fusion approaches, such as magnetic confinement (tokamaks), inertial confinement (laser-based fusion), and magnetized target fusion.
Meanwhile, nuclear policy and public perception continue to influence the expansion of nuclear power worldwide. While some countries, like Germany, have chosen to phase out nuclear energy due to public concerns following the Fukushima disaster, others, including France, China, Russia, and India, are expanding their nuclear power infrastructure. The global shift toward decarbonization and energy security is likely to drive further investment in nuclear technology. Emerging economies are also looking toward nuclear power as a means of supporting industrialization while reducing reliance on fossil fuels.
Looking ahead, nuclear energy is expected to play a critical role in the transition to a carbon-neutral future. Its ability to provide continuous, high-output, low-carbon electricity makes it a strong complement to renewable energy sources such as wind and solar, which are intermittent by nature. The integration of advanced nuclear reactors, SMRs, and fusion technology will likely define the next era of nuclear power, ensuring that it remains a key player in global energy strategies.
Types of Nuclear Reactors
Nuclear reactors are classified based on their design, coolant type, moderator material, and fuel cycle. Each reactor type has unique characteristics that influence its efficiency, safety, and suitability for different applications. The most common classification includes thermal reactors and fast reactors, as well as emerging advanced reactor designs that aim to improve fuel utilization and reduce radioactive waste.
The most widely used nuclear reactors are light water reactors (LWRs), which use ordinary water as both a coolant and a neutron moderator. The two main types of LWRs are pressurized water reactors (PWRs) and boiling water reactors (BWRs). In a PWR, water is maintained under high pressure to prevent it from boiling inside the reactor core. Instead, the heated water is transferred to a secondary loop where it produces steam to drive a turbine. This design provides additional safety by ensuring that radioactive water does not directly interact with the turbine system. BWRs, on the other hand, allow water to boil directly within the reactor vessel, generating steam that is sent directly to the turbine. While simpler in design, BWRs require more radiation shielding in the turbine area due to the direct interaction between steam and reactor water. Both PWRs and BWRs are widely used around the world, with PWRs being the most common type of commercial reactor.
Another class of reactors is the pressurized heavy water reactor (PHWR), which uses heavy water (D₂O) as both a coolant and a moderator. Heavy water is more effective at slowing down neutrons than ordinary water, allowing PHWRs to use natural uranium as fuel without the need for enrichment. The most well-known PHWR design is the CANDU reactor, developed in Canada. These reactors are known for their online refueling capability, meaning they can be refueled without shutting down the reactor. This feature improves fuel efficiency and operational flexibility. PHWRs are used in several countries, including Canada, India, and Argentina.
A variation of heavy water reactors is the boiling heavy water reactor (BHWR), which functions similarly to a BWR but uses heavy water instead of light water. While not as widely used, this design offers the advantage of enhanced neutron economy, allowing for more efficient use of nuclear fuel.
Gas-cooled reactors (GCRs) are another category that uses gases like carbon dioxide or helium as a coolant instead of water. One example is the advanced gas-cooled reactor (AGR), developed in the United Kingdom. AGRs use carbon dioxide as a coolant and graphite as a moderator, operating at higher temperatures than LWRs. This higher temperature improves thermal efficiency, making AGRs more efficient than water-cooled reactors. The high-temperature gas-cooled reactor (HTGR) is a more advanced version that uses helium as a coolant and can achieve even higher operating temperatures, making it suitable for industrial heat applications in addition to electricity generation.
Another important type of reactor is the fast neutron reactor (FNR), which operates without a neutron moderator, relying on fast neutrons to sustain the fission process. Unlike thermal reactors, which use slow (thermal) neutrons, FNRs can efficiently breed new fissile material from fertile isotopes such as Uranium-238 or Thorium-232. This allows them to generate more fuel than they consume, making them a promising long-term energy solution. One notable FNR design is the liquid metal-cooled fast reactor (LMFR), which uses liquid sodium or lead as a coolant. Examples of operational fast reactors include Russia’s BN-600 and BN-800 reactors, which use sodium as a coolant. These reactors offer high fuel efficiency and can reduce nuclear waste by utilizing spent fuel from conventional reactors.
Another advanced type of nuclear reactor is the molten salt reactor (MSR), which uses liquid fuel dissolved in molten salt. This design allows for high-temperature operation and passive safety features, as molten salt remains stable at high temperatures and operates at atmospheric pressure, reducing the risk of pressure-related accidents. MSRs can also be designed to use thorium as a fuel source, which has the potential to be more abundant and produce less long-lived nuclear waste compared to uranium-based fuels. The most well-known molten salt reactor experiment was conducted in the United States at Oak Ridge National Laboratory in the 1960s. Today, several countries and private companies are working on modern MSR designs to commercialize this technology.
A variation of molten salt reactors is the liquid fluoride thorium reactor (LFTR), which specifically uses thorium-232 as its primary fuel. Thorium has the advantage of being more abundant than uranium and can be converted into Uranium-233, a fissile material that sustains the fission reaction. LFTRs are being explored as a cleaner and more sustainable alternative to conventional uranium-based reactors.
Another emerging category is the small modular reactor (SMR), which is not a specific type of reactor but rather a design concept that applies to various reactor types, including PWRs, FNRs, and MSRs. SMRs are designed to be smaller, factory-built, and modular, meaning multiple units can be deployed in different locations based on energy demand. These reactors typically generate less than 300 MW of electricity, compared to the 1,000+ MW output of conventional reactors. Their modular nature makes them easier to transport, install, and scale up, making them an attractive option for remote areas, industrial facilities, and developing countries. Countries such as the United States, Canada, China, and Russia are actively developing SMR technology, with several designs expected to be deployed in the coming years.
In addition to fission-based reactors, research is ongoing in nuclear fusion reactors, which aim to replicate the energy-producing process of the Sun. Unlike fission, which splits heavy atomic nuclei, fusion combines light atomic nuclei, such as deuterium and tritium, to form helium and release vast amounts of energy. The most prominent fusion research project is the International Thermonuclear Experimental Reactor (ITER) in France, which is expected to demonstrate large-scale fusion energy production by the 2030s. Fusion reactors, such as tokamaks and stellarators, require extremely high temperatures and advanced magnetic confinement techniques to sustain a controlled fusion reaction. If successful, fusion technology could provide virtually limitless, clean energy with minimal radioactive waste and no risk of meltdowns.
Overall, the diversity of nuclear reactor designs reflects the continuous evolution of nuclear technology to address challenges related to fuel efficiency, waste management, safety, and sustainability. While traditional reactors like PWRs and BWRs dominate the global nuclear fleet, advanced reactor designs such as fast reactors, molten salt reactors, and thorium reactors hold significant promise for the future. Additionally, the development of SMRs and fusion reactors could revolutionize the nuclear industry by making nuclear energy more accessible, flexible, and environmentally friendly. With ongoing research and investment, nuclear power is expected to play a crucial role in the transition to a low-carbon energy future, complementing renewable energy sources such as solar and wind while providing a reliable and sustainable source of electricity.
The development of nuclear reactors continues to evolve as governments, research institutions, and private companies seek to improve efficiency, safety, and sustainability. One of the key challenges facing nuclear power is the need for better fuel utilization and waste reduction, leading to the advancement of closed fuel cycles, breeder reactors, and thorium-based nuclear systems.
Closed Fuel Cycles and Breeder Reactors
Traditional nuclear reactors operate on an open fuel cycle, meaning that once the fuel is used, it is discarded as spent nuclear fuel and stored as radioactive waste. However, a closed fuel cycle aims to reprocess and recycle spent fuel, reducing the amount of high-level waste and making more efficient use of uranium resources. Some advanced reactor designs, such as fast breeder reactors (FBRs), are specifically designed to convert fertile isotopes (Uranium-238 or Thorium-232) into fissile materials (Plutonium-239 or Uranium-233), effectively breeding new fuel while generating power.
Fast breeder reactors operate using fast neutrons rather than thermal neutrons, eliminating the need for a neutron moderator. These reactors typically use liquid metal coolants, such as sodium or lead-bismuth, which allow for higher operating temperatures and improved thermal efficiency. The ability of breeder reactors to generate more fissile material than they consume makes them an attractive long-term solution for nuclear fuel sustainability. Countries like Russia, France, Japan, and India have been actively researching and developing FBR technology. Russia’s BN-600 and BN-800 reactors, as well as India’s Prototype Fast Breeder Reactor (PFBR), represent significant steps toward commercializing this technology.
Thorium-Based Nuclear Reactors
Thorium has been gaining attention as an alternative nuclear fuel due to its abundance, safety advantages, and waste reduction potential. Unlike uranium, thorium-232 is not directly fissile, meaning it must be converted into Uranium-233 through neutron absorption before it can sustain a nuclear chain reaction. This process occurs naturally in specially designed reactors, such as liquid fluoride thorium reactors (LFTRs) and heavy water reactors (HWRs).
One of the key advantages of thorium-based reactors is their ability to produce less long-lived radioactive waste compared to uranium-based systems. Additionally, thorium does not generate plutonium, which is a key concern for nuclear weapons proliferation. Countries such as India, China, and Norway are leading the development of thorium-based nuclear power due to their large domestic thorium reserves. India, in particular, has an extensive three-stage nuclear power program aimed at transitioning from uranium-fueled reactors to breeder reactors and eventually thorium-fueled reactors.
Molten Salt Reactors (MSRs) and Next-Generation Nuclear Technology
Molten salt reactors (MSRs) represent a major shift in nuclear reactor technology by using liquid fuel dissolved in molten fluoride or chloride salts instead of traditional solid fuel rods. This design offers several key benefits, including higher operating temperatures, improved thermal efficiency, and passive safety features. Because molten salt reactors operate at low pressure, they eliminate the risk of steam explosions or pressure-related accidents, which are concerns in traditional water-cooled reactors.
One of the most promising MSR designs is the liquid fluoride thorium reactor (LFTR), which combines the benefits of thorium fuel with molten salt technology. LFTRs can operate with a continuous fuel reprocessing system, meaning they can extract valuable fissile material from spent fuel and significantly reduce nuclear waste. Several companies and research institutions, including TerraPower, Copenhagen Atomics, and the Chinese Academy of Sciences, are working on commercializing MSR technology.
Small Modular Reactors (SMRs) and Microreactors
In addition to new reactor designs, the nuclear industry is focusing on small modular reactors (SMRs) and microreactors as a way to provide flexible, scalable, and cost-effective nuclear power. Unlike large conventional reactors, which require multi-billion-dollar investments and years of construction, SMRs are designed to be factory-built, transportable, and rapidly deployed.
SMRs typically generate between 10 MW and 300 MW of electricity, making them ideal for remote communities, industrial facilities, military bases, and developing countries. Many SMR designs incorporate passive safety features, such as self-cooling capabilities, which eliminate the need for external power sources in case of an emergency. Some SMRs are based on existing light water reactor technology, while others use advanced reactor designs, such as high-temperature gas-cooled reactors (HTGRs), molten salt reactors (MSRs), and sodium-cooled fast reactors (SFRs).
Several countries are making significant progress in SMR deployment. In the United States, companies like NuScale Power and X-energy are developing SMRs for commercial use, while Canada is supporting SMR development for use in remote regions and mining operations. Russia has already deployed a floating nuclear power plant, the Akademik Lomonosov, which uses two small pressurized water reactors to provide electricity to Arctic regions.
An even smaller category of nuclear reactors, known as microreactors, is being developed to provide ultra-compact nuclear power solutions. These reactors, typically generating 1 MW to 10 MW, are designed to be transportable, self-regulating, and capable of operating autonomously for several years without refueling. Microreactors have potential applications in disaster relief, space exploration, and decentralized energy systems.
Fusion Reactors: The Future of Nuclear Power
While current nuclear power plants rely on fission, in which heavy atomic nuclei split to release energy, scientists are actively researching nuclear fusion, the process that powers the Sun and stars. Fusion offers the promise of limitless, clean, and safe energy, as it generates minimal radioactive waste and poses no risk of meltdowns.
In a fusion reactor, hydrogen isotopes (deuterium and tritium) are combined under extreme temperature and pressure to form helium, releasing massive amounts of energy. The challenge lies in maintaining the necessary conditions for a sustained fusion reaction. The most common approach is the tokamak design, which uses powerful magnetic fields to confine superheated plasma. The International Thermonuclear Experimental Reactor (ITER) in France is the world’s largest fusion research project, aiming to demonstrate the feasibility of large-scale fusion power by the 2030s. Other fusion projects, such as the Stellarator (Wendelstein 7-X in Germany) and inertial confinement fusion (ICF) using laser technology, are also being explored.
Private companies, including Commonwealth Fusion Systems, Helion Energy, and Tokamak Energy, are working on compact fusion reactors, with some claiming that they could achieve commercial fusion power within the next two decades. If successful, fusion energy could revolutionize the world’s electricity supply, providing an almost limitless and clean energy source that could replace fossil fuels entirely.
The Future of Nuclear Energy in a Low-Carbon World
As the world shifts toward clean energy solutions to combat climate change, nuclear power is expected to play a vital role in achieving carbon neutrality. Unlike fossil fuel power plants, nuclear reactors do not produce carbon dioxide (CO₂) emissions, making them a key option for decarbonizing electricity generation.
With advancements in breeder reactors, molten salt reactors, SMRs, and fusion technology, the future of nuclear power looks promising. While challenges remain, including public perception, regulatory hurdles, and long-term waste management, ongoing research and innovation are paving the way for a new generation of nuclear reactors that are safer, more efficient, and more sustainable.
The integration of advanced nuclear energy with renewable sources like solar and wind will be crucial in ensuring a stable and low-carbon energy grid. As governments and industries invest in next-generation nuclear technologies, nuclear power is likely to remain a cornerstone of global energy policy, supporting the transition toward a sustainable and emission-free future.
Innovations in Nuclear Fuel and Waste Management
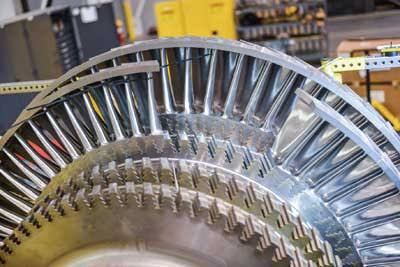
One of the most critical aspects of nuclear energy development is fuel efficiency and waste management. Traditional nuclear reactors use uranium-based fuel, but advancements in nuclear fuel technology are improving the sustainability and safety of nuclear power.
Advanced Nuclear Fuels
Conventional nuclear reactors primarily use Uranium-235 (U-235) enriched to about 3-5%. However, research is focused on developing advanced nuclear fuels that offer higher efficiency, increased safety, and reduced waste production.
- High-Assay Low-Enriched Uranium (HALEU):
- HALEU fuel is enriched to between 5% and 20% U-235, compared to traditional low-enriched uranium (LEU), which is limited to 5% U-235.
- HALEU is expected to be a key fuel for next-generation reactors, including SMRs and advanced fast reactors.
- Its higher enrichment level allows for greater efficiency, longer fuel cycles, and reduced nuclear waste.
- TRISO (Tristructural-Isotropic) Fuel:
- TRISO fuel consists of tiny, spherical uranium fuel particles encased in multiple layers of ceramic and carbon coatings.
- This fuel is highly resistant to radiation damage and extreme temperatures, making it ideal for high-temperature gas-cooled reactors (HTGRs) and SMRs.
- The structural integrity of TRISO particles prevents meltdowns, enhancing reactor safety.
- The U.S. Department of Energy (DOE) and companies like X-energy are actively developing TRISO-based reactors.
- Metallic Fuels:
- Metallic uranium or uranium-zirconium alloy fuels offer higher thermal conductivity, allowing for better heat dissipation and increased reactor efficiency.
- These fuels are particularly well-suited for fast neutron reactors (FNRs) and liquid metal-cooled reactors.
- Thorium-Based Fuels:
- Thorium (Th-232) is an alternative nuclear fuel that can be converted into Uranium-233 (U-233), a fissile material that sustains nuclear reactions.
- Thorium is three to four times more abundant than uranium, and its use can significantly reduce long-lived nuclear waste.
- India, China, and Norway are leading research in thorium reactor technology.
Nuclear Waste Reduction and Recycling
Spent nuclear fuel from reactors remains radioactive and requires long-term storage. Current methods for managing nuclear waste include geological disposal, reprocessing, and transmutation technologies.
- Nuclear Fuel Reprocessing (Closed Fuel Cycle):
- Traditional reactors use only about 5% of the potential energy in uranium fuel before it is classified as waste.
- Reprocessing spent fuel allows for the extraction of unused uranium and plutonium, which can be recycled into new fuel.
- Countries like France, Russia, and Japan have established fuel reprocessing facilities to reduce waste and improve fuel efficiency.
- The PUREX (Plutonium and Uranium Recovery by Extraction) process is the most widely used method for reprocessing spent fuel.
- Fast Reactors and Actinide Recycling:
- Fast neutron reactors (FNRs) can burn long-lived transuranic elements (e.g., plutonium and minor actinides), reducing the radioactive lifespan of nuclear waste.
- This process is known as transmutation, where highly radioactive isotopes are converted into shorter-lived or non-radioactive elements.
- Russia’s BN-800 reactor and France’s proposed ASTRID reactor are examples of fast reactors designed for waste reduction.
- Deep Geological Repositories (Long-Term Storage):
- For high-level radioactive waste that cannot be reprocessed, deep underground repositories are considered the safest disposal method.
- Countries like Finland, Sweden, and Canada are leading the way in developing permanent geological storage sites.
- Finland’s Onkalo repository is the world’s first permanent nuclear waste disposal facility, expected to begin operations by the 2030s.
- Molten Salt Reactors (MSRs) and Waste Burning:
- MSRs can use spent nuclear fuel as a fuel source, reducing existing nuclear waste.
- Some MSR designs can operate on U-233, plutonium, or minor actinides, helping to eliminate long-lived radioactive materials from spent fuel stockpiles.
Advanced Nuclear Safety and Passive Safety Systems
Nuclear reactor safety has significantly improved with modern designs that incorporate passive safety features to prevent accidents without human intervention.
- Passive Cooling Systems:
- Unlike traditional reactors that require external cooling pumps, passive safety reactors use natural convection, gravity, and heat dissipation to cool the reactor core.
- Examples include NuScale’s SMR design, which can safely shut down and cool itself without external power.
- Inherently Safe Fuel Designs:
- TRISO fuel and molten salt fuels are designed to withstand extreme temperatures without melting or releasing radiation.
- MSRs, for example, have a freeze plug system that automatically drains molten fuel into a safe containment tank if overheating occurs.
- Modular and Underground Reactor Construction:
- SMRs and some advanced reactor designs are being built underground or underwater, reducing the risk of external threats like earthquakes or terrorist attacks.
- Automated Reactor Control and AI Monitoring:
- AI and machine learning are being integrated into nuclear power plants to monitor reactor performance, predict failures, and optimize fuel usage.
Integration of Nuclear Energy with Renewables
As the global energy transition accelerates, nuclear power is being integrated with renewable energy sources like solar and wind to create a stable, low-carbon energy grid.
- Hybrid Nuclear-Renewable Energy Systems:
- Nuclear reactors can provide baseload power to support intermittent renewables.
- Advanced reactors can be used for hydrogen production, desalination, and industrial heat applications, reducing reliance on fossil fuels.
- Nuclear-Powered Hydrogen Production:
- High-temperature reactors (HTGRs and MSRs) can produce hydrogen through thermochemical cycles, enabling clean hydrogen production for transportation and industry.
- Countries like Japan, South Korea, and the United States are investing in nuclear-powered hydrogen plants.
Space Exploration and Nuclear Energy
Nuclear energy is also being explored for space applications, including space propulsion, lunar bases, and deep-space exploration.
- Nuclear Thermal Propulsion (NTP):
- NTP engines, using nuclear reactors to heat propellant, could enable faster travel to Mars and beyond.
- NASA and private companies are developing reactor-based propulsion systems for deep-space missions.
- Kilopower Reactors for Space Colonization:
- Small nuclear reactors, like NASA’s Kilopower project, could provide continuous power for lunar and Martian colonies.
- These compact reactors are designed to operate in extreme environments with minimal maintenance.
Conclusion: The Future of Nuclear Power
As energy demand rises and climate concerns intensify, nuclear power is poised to play a key role in the global transition to clean energy. Advancements in reactor design, fuel technology, waste management, and safety are making nuclear energy more sustainable, efficient, and adaptable.
With the development of small modular reactors, molten salt reactors, fusion technology, and hybrid nuclear-renewable systems, nuclear energy is set to become a cornerstone of the world’s clean energy future. Investment in next-generation nuclear technologies will ensure that nuclear power continues to provide reliable, low-carbon electricity for decades to come.
Public Perception and Policy Challenges in Nuclear Energy
Despite the advancements in reactor safety, fuel technology, and waste management, the nuclear industry faces public perception challenges, regulatory hurdles, and economic factors that impact its expansion. Addressing these issues is critical for the future of nuclear power as a key solution for decarbonization and energy security.
Public Perception and Nuclear Safety Concerns
The perception of nuclear energy has been shaped by historical accidents, misinformation, and public fear regarding radiation and nuclear waste. Events such as Three Mile Island (1979), Chernobyl (1986), and Fukushima (2011) have left lasting concerns about nuclear safety, even as modern reactors incorporate significant safety improvements.
- Radiation Misconceptions:
- Public fears about radiation exposure are often exaggerated, as nuclear power plants emit minimal radiation compared to natural sources like radon, cosmic rays, and medical imaging.
- Education campaigns and transparent communication are essential to counter misinformation and build public trust in nuclear energy.
- Nuclear Accidents and Lessons Learned:
- Chernobyl (1986): Caused by design flaws and operator error, leading to a catastrophic explosion and widespread contamination.
- Fukushima (2011): Triggered by a massive earthquake and tsunami, highlighting the need for improved backup power and passive cooling systems.
- Modern reactors integrate passive safety features, advanced monitoring, and improved containment structures to prevent similar incidents.
- Public Support and Opposition:
- Some countries, like France, Russia, and China, have strong government backing and public acceptance of nuclear power.
- Others, such as Germany, have chosen to phase out nuclear energy due to public pressure, despite its role in reducing carbon emissions.
- Younger generations are increasingly supporting nuclear energy as a climate solution, especially as renewable energy faces challenges related to intermittency and storage.
Regulatory and Economic Challenges
The nuclear industry faces significant regulatory barriers, high capital costs, and lengthy approval processes, which slow down the deployment of new reactors.
- Strict Licensing and Regulatory Approvals:
- Nuclear reactors require extensive safety reviews, environmental impact assessments, and regulatory approvals, leading to delays and cost overruns.
- In some countries, the process of licensing a new reactor can take 10–15 years, making it difficult to compete with faster-deploying renewable energy projects.
- Streamlining regulatory frameworks for advanced reactors could help accelerate nuclear deployment.
- High Construction and Capital Costs:
- Large nuclear plants require billions of dollars in upfront investment, making financing a major barrier.
- Cost overruns and delays have plagued projects like Hinkley Point C (UK) and Vogtle Units 3 & 4 (USA).
- Small Modular Reactors (SMRs) and factory-built reactors aim to reduce costs through standardization and mass production.
- Competition with Renewable Energy and Natural Gas:
- Solar and wind power have seen rapid cost declines, making them attractive alternatives to nuclear energy.
- However, renewables require energy storage solutions, while nuclear provides consistent, baseload power.
- Carbon pricing and government incentives could help level the playing field by accounting for nuclear’s role in reducing greenhouse gas emissions.
- Nuclear Waste Policy and Public Acceptance:
- Long-term disposal of spent nuclear fuel remains a political and technical challenge.
- Some countries, like Finland and Sweden, are leading the way with deep geological repositories, while others rely on temporary storage.
- Public opposition to nuclear waste transport and disposal sites can delay progress.
Government Policies and Global Nuclear Expansion
Despite challenges, many governments are recognizing the importance of nuclear power in achieving climate goals and energy security.
- Pro-Nuclear Policies and Investments:
- Countries like China, Russia, France, and India are heavily investing in nuclear energy, with dozens of new reactors under construction.
- The United States and Canada are supporting advanced reactor development, particularly SMRs, to ensure domestic energy security.
- Phasing Out vs. Expanding Nuclear Power:
- Germany shut down its last nuclear plants in 2023, increasing reliance on coal and natural gas.
- France, Japan, and South Korea have reversed previous plans to phase out nuclear, recognizing its role in reducing carbon emissions.
- International Collaboration on Nuclear Innovation:
- Organizations like the International Atomic Energy Agency (IAEA) and Generation IV International Forum (GIF) promote research on next-generation reactors.
- Russia and China are leading in exporting nuclear technology, including SMRs and floating nuclear plants.
- The U.S.-led Partnership for Global Infrastructure aims to compete with China’s nuclear exports by financing SMRs in developing countries.
The Role of Nuclear Energy in the 21st Century
As the world faces the dual challenges of climate change and energy security, nuclear power remains one of the most reliable and scalable sources of low-carbon electricity.
- Nuclear as a Climate Solution:
- Nuclear power plants prevent over 2 billion tons of CO₂ emissions annually, making them critical for net-zero targets.
- Countries integrating nuclear energy with renewables and hydrogen production will have more resilient energy systems.
- Future Innovations in Nuclear Technology:
- Fusion power could revolutionize energy production if commercial viability is achieved.
- Next-generation reactors (Gen IV), including molten salt, fast neutron, and thorium reactors, promise greater efficiency and sustainability.
- Decentralized and Microreactor Deployment:
- The rise of microreactors could make nuclear energy more accessible for remote areas, military bases, and disaster recovery.
- Companies like Oklo, Westinghouse, and Rolls-Royce are pioneering ultra-compact, portable nuclear power solutions.
Conclusion: A Nuclear Renaissance?
Despite historical setbacks, nuclear energy is experiencing a global resurgence, driven by the urgent need for clean, reliable, and sustainable power. With advancements in safety, modularity, and fuel technology, nuclear energy is poised to complement renewables in the transition to a low-carbon future.
The next decade will determine whether advanced reactors, SMRs, and fusion technology can overcome economic and regulatory barriers, leading to a nuclear renaissance that supports global climate goals and energy security.
Advantages of Nuclear Power
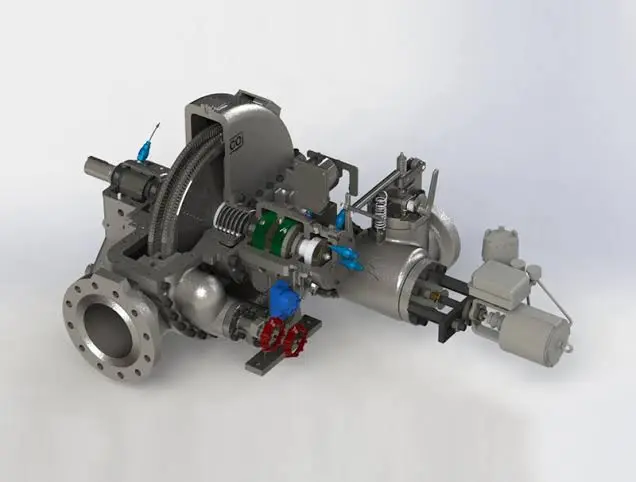
Nuclear power is a crucial component of the global energy mix, offering numerous economic, environmental, and technological benefits. As nations seek reliable, low-carbon energy sources to combat climate change, nuclear energy remains a key contender for sustainable power generation. Below are the primary advantages of nuclear power:
Low Carbon Emissions
One of the most significant benefits of nuclear power is its minimal carbon footprint. Unlike fossil fuels, nuclear power plants do not emit CO₂ or greenhouse gases during electricity generation.
- A single 1,000 MW nuclear plant prevents the emission of 5–8 million tons of CO₂ annually compared to coal-fired power plants.
- According to the International Energy Agency (IEA), nuclear energy has prevented over 60 gigatons of CO₂ emissions globally since 1971.
- Countries striving for net-zero emissions by 2050 recognize nuclear power as a critical component of their decarbonization strategies.
High Energy Density and Efficiency
Nuclear fuel has an extremely high energy density, meaning a small amount of uranium produces a vast amount of energy.
- 1 gram of uranium-235 can generate approximately 24,000 kWh of electricity, compared to only 8 kWh from 1 gram of coal.
- A single nuclear reactor can generate as much power as hundreds of wind turbines or thousands of solar panels.
- This efficiency makes nuclear power a cost-effective solution for supplying large-scale baseload electricity.
Reliable and Continuous Power Supply
Unlike solar and wind power, which are dependent on weather conditions, nuclear power provides stable and continuous electricity 24/7.
- Nuclear plants operate at a capacity factor of over 90%, meaning they generate electricity more than 90% of the time.
- In contrast, solar power has a capacity factor of around 20-30%, and wind power ranges from 30-50%.
- This reliability makes nuclear power essential for maintaining grid stability and energy security.
Reduces Dependence on Fossil Fuels
Nuclear energy reduces reliance on coal, oil, and natural gas, decreasing exposure to volatile fuel prices and geopolitical risks.
- Countries with strong nuclear programs, like France and Canada, have low electricity costs and energy independence.
- Nuclear power allows nations to diversify their energy portfolios, reducing reliance on imported fossil fuels.
Advanced Safety Measures and Low Accident Rate
Despite past accidents, modern nuclear reactors are designed with passive safety features that significantly reduce risks.
- Generation III+ and IV reactors have automatic shutdown systems, improved cooling mechanisms, and containment structures to prevent meltdowns.
- Small Modular Reactors (SMRs) feature self-cooling systems and underground construction, enhancing safety.
- The nuclear industry has one of the lowest accident and fatality rates compared to coal, oil, and hydroelectric power.
Small Land Footprint
Compared to solar farms and wind parks, nuclear plants require far less land per unit of electricity generated.
- A 1,000 MW nuclear plant occupies around 1-4 km², while a solar farm of the same capacity requires 20-50 km².
- This compact footprint makes nuclear power ideal for urbanized regions with limited land availability.
Potential for Advanced Reactors and New Technologies
Future nuclear technologies, such as Small Modular Reactors (SMRs), Molten Salt Reactors (MSRs), and Fast Neutron Reactors (FNRs), promise even greater efficiency, safety, and sustainability.
- SMRs offer flexible deployment, shorter construction times, and lower costs.
- Thorium reactors could provide safer, more abundant fuel alternatives with reduced long-lived waste.
- Fusion energy, if commercialized, could revolutionize power generation by producing virtually limitless clean energy with minimal waste.
Long Fuel Life and Reduced Resource Extraction
Unlike fossil fuels, nuclear fuel is highly efficient and requires less frequent replacement.
- A single nuclear fuel rod can last 3-5 years, whereas coal and gas plants require constant fuel input.
- Advanced reactor designs can reprocess spent fuel, further reducing the need for uranium mining.
Creates High-Paying Jobs and Boosts Economy
Nuclear energy supports high-skilled, long-term jobs across various sectors, including engineering, construction, and research.
- The World Nuclear Association (WNA) estimates that a nuclear power plant employs 400–700 full-time workers, compared to 50-100 for a natural gas plant.
- Countries investing in nuclear energy experience economic growth through innovation, infrastructure development, and energy exports.
Potential for Hydrogen Production and Industrial Use
Nuclear reactors can be used for more than just electricity generation—they can also produce clean hydrogen and supply heat for industrial processes.
- High-temperature gas-cooled reactors (HTGRs) can generate low-carbon hydrogen, reducing dependence on fossil fuels in industries like steelmaking and transportation.
- Nuclear energy could be a key driver in the hydrogen economy, supporting clean fuel production for a wide range of applications.
Conclusion
Nuclear power remains one of the most reliable, efficient, and environmentally friendly energy sources available today. With advancements in reactor safety, fuel technology, and waste management, nuclear energy is poised to play a critical role in the transition to a sustainable, low-carbon future.
While challenges such as high capital costs and regulatory barriers exist, continued investment in next-generation nuclear technologies and public education can help overcome these obstacles. As the world moves towards net-zero emissions, nuclear energy is expected to be a cornerstone of global energy security and decarbonization efforts.
Challenges and Future Prospects of Nuclear Power
Despite its numerous advantages, nuclear power faces economic, political, and technological challenges that impact its growth and acceptance. However, advancements in next-generation reactors, waste management, and public perception strategies could shape the future of nuclear energy in a low-carbon world.
High Initial Costs and Long Construction Times
One of the most significant barriers to nuclear energy expansion is the high capital cost of building new reactors.
- The construction of large-scale nuclear power plants often requires billions of dollars in investment and 10–15 years for completion.
- Projects like Hinkley Point C (UK) and Vogtle Units 3 & 4 (USA) have faced cost overruns and delays, making investors hesitant.
- Modular reactor designs (SMRs) could reduce costs through factory-based manufacturing and faster deployment.
Nuclear Waste Management and Public Concerns
Although nuclear energy produces far less waste than fossil fuels, the disposal of spent nuclear fuel remains a major challenge.
- High-level waste (HLW) remains radioactive for thousands of years and requires secure, long-term storage.
- Countries like Finland and Sweden have developed deep geological repositories, but public opposition often delays their implementation in other nations.
- Advanced fuel recycling and new reactor designs could significantly reduce waste and improve sustainability.
Public Perception and Nuclear Fear
Misinformation and fear surrounding radiation, nuclear accidents, and waste disposal have led to strong opposition in some regions.
- The impact of disasters like Chernobyl (1986) and Fukushima (2011) continues to shape public opinion, even though modern reactors have vastly improved safety measures.
- Educational campaigns and transparent government policies are crucial to building public trust in nuclear energy.
Competition with Renewable Energy and Energy Storage
The rapid growth of solar, wind, and battery storage technologies presents competition for nuclear power.
- Solar and wind energy costs have declined significantly, leading some governments to prioritize these over nuclear.
- However, renewables are intermittent, requiring backup solutions like energy storage, hydrogen, or nuclear baseload power to maintain grid stability.
- Many experts advocate for a balanced mix of renewables and nuclear energy to ensure a stable and clean electricity supply.
Political and Regulatory Barriers
Nuclear energy is heavily regulated due to safety and security concerns, making approvals for new reactors lengthy and expensive.
- In some countries, nuclear projects must undergo complex licensing procedures, delaying development.
- Geopolitical factors, such as uranium supply chains and international nuclear agreements, also influence nuclear expansion.
- Government incentives, carbon pricing, and streamlined regulations could help make nuclear energy more competitive.
Future Innovations and the Nuclear Renaissance
Despite challenges, nuclear technology is evolving, with promising advancements in reactor safety, efficiency, and sustainability.
- Small Modular Reactors (SMRs):
- Can be mass-produced in factories, reducing costs and construction time.
- Ideal for remote areas, industrial applications, and decentralized power grids.
- Countries like Canada, the U.S., Russia, and China are investing in SMR development.
- Advanced Generation IV Reactors:
- Includes Molten Salt, Fast Neutron, and Thorium reactors, offering higher safety and waste reduction.
- Can reuse spent nuclear fuel, making nuclear energy more sustainable.
- Fusion Energy:
- The ultimate goal of nuclear research, offering limitless clean power with no long-lived waste.
- Experimental projects like ITER and private fusion companies are making progress, but commercialization is still decades away.
Conclusion: The Path Forward
Nuclear power remains one of the most promising solutions for a clean, reliable, and sustainable energy future. While challenges like high costs, public perception, and regulatory barriers persist, advancements in reactor technology, waste management, and policy support could lead to a nuclear renaissance in the coming decades.
As nations seek to achieve net-zero emissions, nuclear power is expected to play a crucial role alongside renewables, hydrogen, and energy storage technologies. By investing in next-generation reactors and improving public engagement, the global energy sector can unlock the full potential of nuclear energy for future generations.
The Role of Nuclear Power in a Sustainable Energy Future
As the world transitions to low-carbon energy systems, nuclear power remains one of the most viable options for achieving energy security, grid stability, and carbon neutrality. Its ability to provide reliable, high-density energy makes it a crucial component in global decarbonization efforts.
Nuclear Power and Climate Change Mitigation
One of the primary drivers for nuclear energy expansion is its role in reducing greenhouse gas (GHG) emissions.
- Nuclear power currently provides about 10% of global electricity and nearly 30% of low-carbon electricity.
- Unlike fossil fuels, nuclear reactors do not produce CO₂, sulfur dioxide, or particulate matter, making them a clean alternative to coal and natural gas.
- Studies from the Intergovernmental Panel on Climate Change (IPCC) emphasize that limiting global warming to 1.5°C will require a significant increase in nuclear energy deployment alongside renewables.
Nations with ambitious net-zero targets (e.g., France, China, Canada, and the UK) are investing in nuclear power to maintain baseload electricity generation while integrating renewable sources like wind and solar.
The Integration of Nuclear Energy with Renewable Sources
While renewables are expanding rapidly, they come with challenges related to intermittency and grid stability. Nuclear power can complement renewables by providing consistent, round-the-clock energy that balances fluctuations in solar and wind generation.
- Hybrid Energy Systems: Combining nuclear with renewables and battery storage enhances grid reliability and resilience.
- Load-Following Reactors: Advanced nuclear designs can ramp up or down to adjust for fluctuations in renewable output.
- Hydrogen Production: Nuclear plants can generate low-carbon hydrogen, supporting sectors like transportation and heavy industry.
By integrating nuclear, solar, wind, and storage solutions, countries can create diverse, resilient energy portfolios that ensure long-term sustainability and energy independence.
The Global Expansion of Nuclear Power
Several countries are actively expanding their nuclear power programs, recognizing its strategic importance for energy security and economic growth.
Leading Nuclear Power Nations:
- France: One of the largest nuclear producers, generating over 70% of its electricity from nuclear power. Plans to build new reactors to meet climate goals.
- China: Rapidly expanding its nuclear fleet with over 20 reactors under construction and investments in advanced reactor designs.
- Russia: A major exporter of nuclear technology, with ongoing projects in India, Egypt, Turkey, and Bangladesh.
- United States: While facing economic challenges, the U.S. is developing Small Modular Reactors (SMRs) and extending the lifespans of existing plants.
- India: Expanding its nuclear capacity to support its growing economy while reducing dependence on coal-fired power plants.
Other nations, including Canada, South Korea, Japan, and the UK, are also reinvesting in nuclear energy as part of their long-term climate strategies.
The Future of Nuclear Reactor Technologies
Next-generation nuclear reactors promise to address cost, safety, and waste management issues, making nuclear energy more competitive and sustainable.
Small Modular Reactors (SMRs):
- Scalable and factory-produced, allowing for lower costs and faster deployment.
- Ideal for remote regions, industrial applications, and grid flexibility.
- SMRs are being developed by companies like NuScale (USA), Rolls-Royce (UK), and Rosatom (Russia).
Generation IV Reactors:
- Molten Salt Reactors (MSRs): Use liquid fuel with inherent safety features and minimal waste production.
- Fast Neutron Reactors (FNRs): Can reuse spent fuel, reducing nuclear waste and improving resource efficiency.
- High-Temperature Gas Reactors (HTGRs): Enable efficient hydrogen production for clean fuel applications.
Nuclear Fusion:
- The “Holy Grail” of energy, offering unlimited power with no risk of meltdown and minimal waste.
- Research projects like ITER (France), SPARC (MIT), and private companies like Helion Energy are working towards commercialization.
- While fusion is still decades away, breakthroughs in plasma physics, superconducting magnets, and AI-driven optimization are accelerating progress.
Overcoming Public and Political Barriers
For nuclear power to thrive, governments and energy companies must address public concerns, regulatory challenges, and misinformation.
Key Strategies for Nuclear Advocacy:
- Public Education & Transparency: Clear communication on nuclear safety, radiation risks, and waste disposal can build public trust.
- Regulatory Reform: Streamlining approval processes can reduce costs and accelerate nuclear deployment.
- International Cooperation: Joint research initiatives and technology-sharing agreements can drive global nuclear innovation.
- Investment in Advanced Reactors: Government funding for SMRs, fusion, and waste recycling will enhance nuclear energy’s long-term viability.
With effective policy support, public engagement, and technological innovation, nuclear power can overcome existing barriers and play a leading role in the global clean energy transition.
Conclusion: The Next Era of Nuclear Power
As the world faces increasing energy demands and climate challenges, nuclear power is positioned as a critical solution for low-carbon, high-efficiency energy production. While costs, regulatory issues, and public perception remain obstacles, advancements in reactor design, fuel technology, and waste management are paving the way for a nuclear resurgence.
In the coming decades, nuclear power—alongside renewables, energy storage, and hydrogen production—will be essential for creating a sustainable, resilient, and low-carbon global energy system. If supported by strong policies and continued innovation, nuclear energy could usher in a new era of clean, reliable power for future generations.
The Role of Nuclear Power in a Resilient Energy Future
As global energy systems evolve, nuclear power is expected to play a more dynamic role in ensuring energy resilience, economic stability, and deep decarbonization. While challenges remain, advancements in reactor technologies, fuel cycles, and hybrid energy systems position nuclear as a cornerstone of future energy strategies.
Enhancing Energy Security with Nuclear Power
Nuclear energy provides nations with a stable, domestically controlled power source, reducing dependence on imported fossil fuels and insulating economies from volatile energy markets.
- Reducing Reliance on Gas and Coal Imports:
- Countries like France, Canada, and South Korea have successfully reduced their dependence on coal and natural gas by prioritizing nuclear energy.
- The Russia-Ukraine conflict has highlighted the risks of overreliance on imported fossil fuels, prompting European nations to reconsider nuclear energy expansion.
- Diversified Energy Mix for Stability:
- Nuclear can operate as a baseload power source, supporting grid reliability as solar and wind capacity expands.
- Countries with a high share of nuclear power, such as Sweden and Finland, experience lower electricity price volatility than those heavily reliant on fossil fuel imports.
With energy markets experiencing frequent supply chain disruptions, nuclear power offers a long-term, strategic energy asset that enhances national security.
Innovations in Nuclear Fuel and Waste Management
The long-term sustainability of nuclear energy depends on its ability to maximize fuel efficiency and minimize waste production. Advanced technologies are addressing these challenges.
a. Recycling and Reprocessing Spent Fuel
- Traditional once-through fuel cycles leave behind high-level waste with long-lived isotopes.
- New reprocessing techniques, such as those used in France, Russia, and Japan, extract usable uranium and plutonium from spent fuel.
- Fast Neutron Reactors (FNRs) can utilize recycled fuel, reducing the volume and toxicity of nuclear waste.
b. Deep Geological Repositories for Safe Storage
- Countries like Finland (Onkalo Project) and Sweden are pioneering geological disposal sites for high-level waste.
- These sites provide permanent, passive containment, eliminating risks associated with above-ground storage pools.
c. Advanced Fuel Types for Next-Generation Reactors
- Thorium-Based Fuel: Offers enhanced proliferation resistance and abundant global reserves.
- TRISO Fuel (Tri-Structural Isotropic Particles):
- Used in High-Temperature Gas Reactors (HTGRs) and Molten Salt Reactors (MSRs).
- Highly resistant to meltdown scenarios, making reactors inherently safer.
- Metallic and Accident-Tolerant Fuels (ATFs):
- Improve heat dissipation and enhance reactor performance under extreme conditions.
Efforts in fuel innovation and waste reduction are crucial to ensuring the long-term viability of nuclear power.
Small Modular Reactors (SMRs) and Their Potential Impact
SMRs are emerging as a transformative technology that could make nuclear power more flexible, affordable, and accessible.
Key Advantages of SMRs:
- Lower Initial Costs and Shorter Construction Times:
- Factory-based manufacturing reduces construction risks and delays.
- Countries like Canada, the U.S., and the UK are investing in SMR deployment.
- Scalability and Regional Deployment:
- Ideal for off-grid locations, industrial sites, and disaster recovery zones.
- Countries with remote communities, such as Canada and Russia, are testing SMRs to replace diesel generators.
- Enhanced Safety Features:
- Passive cooling systems prevent overheating without external power sources.
- Many SMRs are designed for underground or underwater installation, reducing risks of sabotage or attacks.
Notable SMR Projects Worldwide:
- NuScale Power (USA): First SMR design approved by the U.S. Nuclear Regulatory Commission (NRC).
- Rolls-Royce SMRs (UK): Focused on rapid deployment and mass production.
- Russia’s Floating SMR (Akademik Lomonosov): Operates in the Arctic, supplying power to remote regions.
- China’s HTR-PM: A high-temperature gas-cooled SMR designed for hydrogen production.
SMRs could revolutionize nuclear power by making it more cost-effective, adaptable, and widely available.
Nuclear Fusion: The Future of Unlimited Clean Energy
While still in the research phase, nuclear fusion could eliminate many of the concerns associated with traditional nuclear fission.
Why Fusion Is Considered the Ultimate Energy Source:
- Virtually Unlimited Fuel Supply:
- Uses isotopes like deuterium and tritium, which are abundant in seawater.
- No Long-Lived Radioactive Waste:
- Unlike fission, fusion does not produce high-level radioactive waste requiring geological storage.
- Inherently Safe Reaction:
- Fusion cannot trigger a runaway reaction or meltdown—if disrupted, the reaction stops automatically.
Ongoing Fusion Research and Key Players:
- ITER (France): The world’s largest fusion experiment, expected to demonstrate net energy gain in the 2030s.
- SPARC (MIT) & Commonwealth Fusion Systems: Using high-temperature superconducting magnets to accelerate fusion breakthroughs.
- Private Companies (Helion Energy, Tokamak Energy): Pursuing compact, commercial-scale fusion reactors by the 2040s.
Although fusion energy remains decades away, continued investment and research could lead to commercial fusion power within the 21st century.
Policy and Investment Strategies for Nuclear Expansion
For nuclear energy to reach its full potential, governments, industries, and international organizations must implement policies that facilitate nuclear deployment.
Key Policy Measures for Supporting Nuclear Energy:
- Carbon Pricing and Incentives for Low-Carbon Energy:
- Establishing carbon taxes or cap-and-trade programs makes nuclear more economically competitive with fossil fuels.
- Streamlined Regulatory Approvals:
- Reducing excessive licensing delays and bureaucratic hurdles can accelerate reactor deployment.
- Public-Private Partnerships for Nuclear Innovation:
- Collaboration between government agencies and private firms can advance SMR and fusion technology commercialization.
- Global Nuclear Cooperation and Non-Proliferation Agreements:
- Ensuring international collaboration in reactor development, uranium enrichment, and nuclear security.
Governments that prioritize nuclear-friendly policies will gain a competitive advantage in the global clean energy market.
Conclusion: A Nuclear-Powered Future
As the world moves toward net-zero emissions and energy resilience, nuclear power will be a key pillar of the clean energy transition.
Key Takeaways:
- Nuclear energy provides stable, low-carbon electricity that complements renewables like solar and wind.
- Advancements in fuel recycling, SMRs, and fusion technology are making nuclear power safer and more cost-effective.
- Global investment in next-generation reactors and supportive policies will determine the future scale and impact of nuclear energy.
The next few decades will be crucial for shaping nuclear’s role in the global energy landscape. If technological innovation, public support, and political commitment align, nuclear power could drive a clean, reliable, and sustainable energy future for generations to come.
The Future of Nuclear Energy: Innovations, Challenges, and Global Prospects
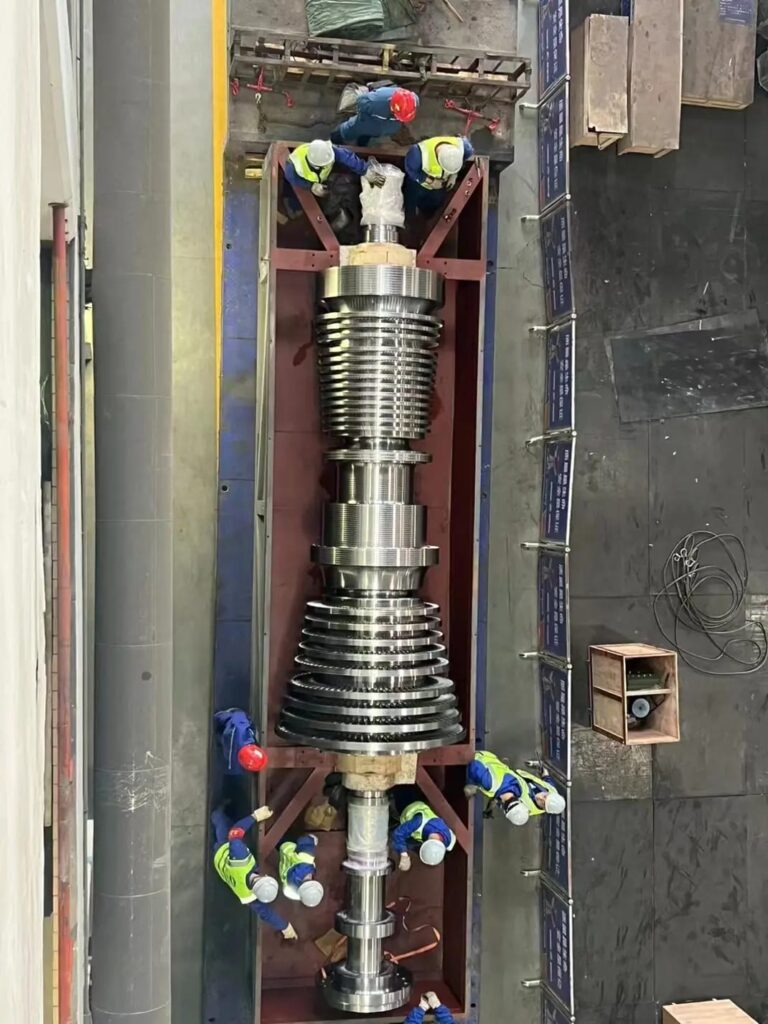
Nuclear energy is at a pivotal moment in history, as climate change, energy security, and technological advancements drive a renewed focus on its potential. While traditional nuclear power has been a reliable source of electricity for decades, the next generation of reactors, fusion energy breakthroughs, and advanced fuel cycles are set to redefine its role in global energy systems.
The Shift Towards Advanced Nuclear Technologies
The future of nuclear energy depends on safer, more efficient, and more cost-effective reactors. Several advanced technologies are currently being developed to address the challenges faced by conventional nuclear power.
a. Small Modular Reactors (SMRs): The Next Evolution in Nuclear Power
SMRs are gaining traction as a flexible and scalable alternative to large traditional nuclear plants.
- Smaller Size, Faster Deployment:
- SMRs are factory-built and modular, allowing for reduced construction times and costs.
- Can be installed in remote areas, industrial sites, or regions with smaller electricity demands.
- Enhanced Safety Features:
- Many SMR designs include passive cooling systems, reducing the risk of meltdowns.
- Underground or submerged installations improve security and resistance to natural disasters.
- Leading SMR Projects:
- NuScale (USA): First SMR design approved by the U.S. Nuclear Regulatory Commission.
- Rolls-Royce SMRs (UK): Focused on mass production and exportability.
- China’s HTR-PM: A high-temperature gas-cooled SMR designed for hydrogen production.
b. Generation IV Reactors: Safer, More Efficient, and Sustainable
Next-generation reactors aim to improve upon traditional nuclear power with better fuel utilization, reduced waste, and passive safety systems.
- Molten Salt Reactors (MSRs):
- Operate at lower pressure with liquid fuel, reducing meltdown risks.
- Can use thorium as a fuel source, offering higher fuel availability and minimal waste production.
- Fast Neutron Reactors (FNRs):
- Can burn existing nuclear waste, making nuclear energy more sustainable.
- Allow for closed fuel cycles, where spent fuel is reprocessed and reused.
- High-Temperature Gas Reactors (HTGRs):
- Operate at extremely high temperatures, enabling hydrogen production and industrial applications.
c. Nuclear Fusion: The Ultimate Clean Energy Source
While still in development, nuclear fusion promises unlimited, clean, and inherently safe energy.
- Key Advantages of Fusion:
- No risk of meltdown—if the reaction is disrupted, it simply stops.
- No long-lived radioactive waste.
- Virtually unlimited fuel supply from isotopes like deuterium and tritium.
- Major Fusion Projects:
- ITER (France): The world’s largest fusion experiment, expected to demonstrate net energy gain in the 2030s.
- SPARC (MIT) & Commonwealth Fusion Systems: Developing compact, commercially viable fusion reactors.
- Helion Energy & Tokamak Energy: Private companies racing to bring fusion power to market within decades.
While commercial fusion power remains at least two to three decades away, rapid advances in superconducting magnets, AI-driven plasma control, and materials science could accelerate its arrival.
Nuclear Energy and the Clean Energy Transition
As countries work towards net-zero carbon emissions, nuclear energy is expected to play a crucial role in decarbonizing electricity grids and supporting renewable energy integration.
a. Nuclear’s Role in a Renewable Energy-Dominated Future
- Stabilizing the Grid:
- Wind and solar energy are intermittent—nuclear provides constant, reliable baseload power.
- Hydrogen Production with Nuclear Reactors:
- Advanced reactors like HTGRs can efficiently produce green hydrogen, an essential fuel for heavy industry and transportation.
- Hybrid Energy Systems:
- Future power grids may integrate nuclear, solar, wind, and energy storage for maximum efficiency.
b. Reducing Dependence on Fossil Fuels
- Countries reliant on coal (e.g., India, China, Poland) are investing in nuclear to transition away from carbon-intensive power.
- Advanced nuclear fuels and waste recycling will make nuclear a long-term, low-carbon solution for energy security.
Challenges and Barriers to Nuclear Expansion
Despite its advantages, nuclear energy still faces economic, political, and societal challenges that must be addressed.
a. High Upfront Costs & Financing Challenges
- Traditional nuclear plants require billions of dollars and over a decade to construct, leading to financial risks.
- New financing models, such as government-backed investments, loan guarantees, and private-public partnerships, are needed to accelerate nuclear deployment.
b. Public Perception and Safety Concerns
- Accidents like Chernobyl (1986) and Fukushima (2011) have left lasting public fears about nuclear safety.
- Improved safety designs, stronger regulations, and transparent communication are essential to rebuilding trust in nuclear energy.
c. Nuclear Waste Management
- High-level nuclear waste remains radioactive for thousands of years, requiring long-term storage solutions.
- Innovations in fuel recycling, deep geological repositories, and advanced reactor designs are helping to reduce nuclear waste concerns.
d. Geopolitical and Regulatory Barriers
- Nuclear energy is often tied to political and non-proliferation concerns, requiring strict international oversight.
- Regulatory frameworks need modernization to support faster deployment of SMRs and next-generation reactors.
Global Trends in Nuclear Energy Expansion
a. Countries Leading Nuclear Growth
Several nations are investing heavily in nuclear energy as part of their long-term energy strategies.
- China:
- Has the fastest-growing nuclear program, with over 20 reactors under construction.
- Developing advanced reactors, including fast breeder and thorium-based designs.
- France:
- Reaffirmed commitment to nuclear power with plans to build new EPR reactors.
- Aims to maintain its high percentage (over 70%) of electricity from nuclear.
- Russia:
- A global leader in exporting nuclear technology, with projects in India, Turkey, Egypt, and Bangladesh.
- United States:
- While facing economic challenges, the U.S. is investing in SMRs and extending reactor lifetimes.
- DOE funding for fusion research and next-generation reactors is increasing.
- India:
- Expanding its nuclear capacity to meet rising energy demands.
- Developing indigenous fast breeder reactors and thorium-based reactors.
b. Countries Reviving or Considering Nuclear Power
- Japan: Restarting nuclear reactors after safety upgrades post-Fukushima.
- Germany: Phasing out nuclear, but facing energy security challenges due to fossil fuel dependency.
- UK, Canada, Poland, and South Korea: Investing in new SMRs and next-gen nuclear plants.
The Next 50 Years of Nuclear Energy
Short-Term (2025-2035):
- Expansion of SMRs and Generation IV reactors.
- Increased focus on nuclear-renewable hybrid grids.
- Fusion experiments (ITER, SPARC) reach net energy gain milestones.
Medium-Term (2035-2050):
- Commercial deployment of fusion power becomes feasible.
- Large-scale nuclear-hydrogen production for industry and transportation.
- Nuclear-powered desalination plants address global water shortages.
Long-Term (2050 and Beyond):
- Fusion becomes the dominant clean energy source.
- Advanced AI-driven reactor management systems make nuclear operations safer and more efficient.
- Global decarbonization is largely achieved, with nuclear playing a key role.
Conclusion: A Nuclear Renaissance?
The future of nuclear energy depends on continued innovation, political commitment, and public acceptance. With new reactor designs, safer fuel cycles, and emerging fusion technologies, nuclear power is poised to play a critical role in the world’s sustainable energy future.
6. Innovations Driving the Future of Nuclear Energy
Technological advancements are reshaping the landscape of nuclear energy, making it safer, more efficient, and more adaptable to the world’s evolving energy needs. Future nuclear reactors will not only generate electricity but will also contribute to hydrogen production, industrial heat applications, and even space exploration.
a. Artificial Intelligence and Automation in Nuclear Energy
The integration of AI, machine learning, and automation is revolutionizing how nuclear plants are designed, operated, and maintained.
- Predictive Maintenance and Fault Detection:
- AI algorithms analyze reactor performance and predict equipment failures before they occur.
- This reduces downtime and lowers operational costs.
- Automated Reactor Control Systems:
- Advanced AI-driven control systems optimize reactor operations in real time.
- Helps improve fuel efficiency and power output stability.
- Enhanced Safety Monitoring:
- AI can detect anomalies, leaks, or deviations in reactor behavior faster than human operators.
- Ensures immediate response to potential safety concerns.
AI-powered automation will play a crucial role in the operation of SMRs, Generation IV reactors, and even future fusion reactors.
b. Advanced Nuclear Fuels: Higher Efficiency and Lower Waste
New fuel technologies are making nuclear energy more economical and environmentally friendly.
1. Accident-Tolerant Fuels (ATFs):
- Developed to withstand extreme temperatures and prevent meltdowns in case of power failures.
- Coated uranium fuel pellets and silicon-carbide cladding reduce the risk of hydrogen explosions (as seen in Fukushima).
2. Thorium-Based Nuclear Fuels:
- Thorium (Th-232) is three times more abundant than uranium.
- Produces less long-lived radioactive waste and is more resistant to nuclear proliferation.
- India, China, and Norway are leading the way in thorium reactor development.
3. TRISO (Tri-Structural Isotropic) Fuel:
- Used in High-Temperature Gas-Cooled Reactors (HTGRs).
- Encapsulated uranium particles prevent radiation leaks, making reactors inherently safer.
- U.S. and China are actively testing TRISO-based reactors.
With improved fuel efficiency and waste reduction, these new fuels will be critical in the long-term sustainability of nuclear energy.
c. Nuclear-Powered Hydrogen Production
Hydrogen is expected to be a key player in decarbonizing industries like steel, chemicals, and transportation. Nuclear energy offers a reliable and efficient way to produce clean hydrogen at scale.
- High-Temperature Gas Reactors (HTGRs) and Molten Salt Reactors (MSRs) can generate industrial-scale hydrogen more efficiently than electrolysis powered by renewables.
- Hydrogen from nuclear energy can replace fossil fuels in industries that are hard to electrify, such as:
- Steel production (replacing coal in blast furnaces)
- Chemical manufacturing (ammonia, fertilizers, plastics)
- Aviation and long-haul transport (hydrogen fuel cells, synthetic fuels)
Nuclear-powered hydrogen could play a pivotal role in achieving global net-zero goals while reducing dependence on fossil fuels.
d. Space Exploration and Nuclear Propulsion
Nuclear technology is expanding beyond Earth and into deep space exploration.
- Nuclear Thermal Propulsion (NTP):
- Uses nuclear reactors to heat propellant (e.g., liquid hydrogen), providing greater efficiency than chemical rockets.
- NASA and private companies are developing nuclear-powered spacecraft for missions to Mars and beyond.
- Radioisotope Thermoelectric Generators (RTGs):
- Power deep-space probes and rovers (e.g., Voyager, Curiosity, Perseverance).
- Uses decaying plutonium-238 to generate electricity in space.
- Nuclear Fission Reactors for Lunar and Martian Bases:
- The NASA Kilopower Project is designing compact nuclear reactors to power future Moon and Mars colonies.
- Provides a constant energy supply for life support, scientific research, and industrial processes.
As humanity expands its presence beyond Earth, nuclear energy will be critical for long-duration space missions and extraterrestrial settlements.
7. Global Policy and Investment Strategies for Nuclear Growth
While technology is advancing rapidly, the future of nuclear energy depends on strong policy frameworks, public acceptance, and strategic investments.
a. Government Policies and Climate Goals
- Countries are re-evaluating their nuclear energy strategies in response to climate change and energy security concerns.
- Carbon pricing and clean energy incentives are making nuclear more competitive with fossil fuels.
- Streamlining regulatory approvals can speed up the deployment of new reactors (especially SMRs).
b. Public-Private Partnerships in Nuclear Energy
- Governments are collaborating with private companies to fund next-generation nuclear projects.
- Private-sector players like Bill Gates’ TerraPower, Rolls-Royce, and Westinghouse are pioneering advanced reactors and SMRs.
c. International Nuclear Collaboration
- Global partnerships (IAEA, EU, U.S.-Japan alliances) are driving nuclear research and safety improvements.
- Countries like China and Russia are aggressively exporting nuclear reactor technology, expanding their influence in the global nuclear market.
A well-coordinated global approach will be necessary to ensure nuclear energy remains a key pillar of sustainable development.
8. The Next Decades: Nuclear Energy in 2040, 2050, and Beyond
By 2040:
- Widespread adoption of SMRs and Generation IV reactors.
- Increased use of nuclear-powered hydrogen production.
- Fusion reactors approach commercial viability.
By 2050:
- Fusion energy breakthroughs enable large-scale, commercial fusion reactors.
- AI-driven autonomous nuclear plants enhance safety and efficiency.
- Nuclear energy provides 30-40% of global electricity, complementing renewables.
Beyond 2050:
- Nuclear-powered space colonies on the Moon and Mars.
- Widespread fusion power eliminates dependence on fossil fuels.
- Nuclear technology contributes to carbon-negative energy systems (e.g., nuclear-powered direct air capture of CO₂).
Conclusion: A New Era of Nuclear Innovation
The future of nuclear energy is brighter than ever, with rapid advancements in reactor technology, fuel cycles, AI integration, and space applications. As the world transitions to low-carbon energy systems, nuclear power will be a key player in ensuring energy security, economic stability, and environmental sustainability.
With continued research, investment, and global collaboration, nuclear energy could soon power the world more safely, efficiently, and sustainably than ever before. 🚀
9. Overcoming Key Challenges in Nuclear Energy Expansion
While nuclear energy offers immense potential, it must overcome several economic, regulatory, and public perception challenges before achieving widespread adoption. Addressing these issues will be crucial for securing its place in the global clean energy transition.
a. Reducing the High Costs of Nuclear Power
One of the biggest obstacles to nuclear energy expansion is high capital costs. Large-scale nuclear plants require billions of dollars and often face delays in construction, making them less competitive compared to renewables like solar and wind.
Strategies to Lower Costs:
- Modular Construction of SMRs
- Factory-produced Small Modular Reactors (SMRs) reduce construction times and capital investment.
- Countries like Canada, the U.K., and the U.S. are investing in fast-tracking SMR deployment.
- Advanced Reactor Designs with Longer Lifespans
- Generation IV reactors aim to extend reactor lifetimes to 60–80 years, maximizing return on investment.
- Fast reactors improve fuel efficiency, reducing fuel costs and waste disposal expenses.
- Public-Private Partnerships & Government Support
- Loan guarantees and subsidies can reduce investment risks for nuclear developers.
- International financing mechanisms (World Bank, IAEA support) could assist developing nations in nuclear adoption.
b. Strengthening Nuclear Waste Management Solutions
Spent nuclear fuel remains one of the most debated concerns around nuclear energy. While nuclear waste volumes are relatively small, long-term disposal solutions remain a challenge.
1. Advanced Waste Recycling & Reprocessing
- Fast Breeder Reactors (FBRs) can reprocess spent fuel, turning waste into new fuel for further use.
- Countries like France, Russia, and Japan have closed fuel cycles, meaning they reuse nuclear waste instead of permanently storing it.
2. Deep Geological Repositories (DGRs)
- Finland’s Onkalo facility is the world’s first operational deep geological nuclear waste repository.
- The U.S., Canada, and Sweden are working on similar projects to safely store high-level nuclear waste for thousands of years.
3. Advanced Reactor Designs with Minimal Waste
- Molten Salt Reactors (MSRs) and thorium reactors generate significantly less radioactive waste than traditional uranium reactors.
- Future reactors may be designed to burn existing nuclear waste, effectively eliminating long-lived radioactive materials.
By investing in better waste recycling and storage solutions, nuclear energy can eliminate one of its largest obstacles to public acceptance.
c. Addressing Public Perception and Nuclear Safety
Public perception remains a significant barrier, often shaped by historical nuclear accidents such as Chernobyl (1986) and Fukushima (2011).
1. Strengthening Reactor Safety Features
Modern nuclear reactors are designed with passive safety systems, meaning they automatically shut down in emergencies without human intervention.
- SMRs and Gen IV reactors use:
- Natural convection cooling, preventing meltdowns even during power failures.
- Underground reactor designs, reducing risks of terrorist attacks or natural disasters.
- AI-driven safety monitoring, ensuring real-time risk detection and response.
2. Transparent Public Communication & Education
- Governments and energy companies must engage with the public, explaining the safety benefits of modern nuclear power.
- Educational campaigns on radiation safety, waste management, and environmental benefits can shift public perception.
3. Improved Regulatory Frameworks
- Many nuclear regulatory processes are outdated and slow, making it difficult to deploy new, safer reactor technologies quickly.
- Fast-tracking approvals for SMRs and advanced reactors will help nuclear energy compete with renewables.
By modernizing regulations and improving public awareness, nuclear energy can regain trust and accelerate its global expansion.
10. The Role of Nuclear Energy in a Decentralized Energy Grid
The global energy system is shifting from large centralized power plants to decentralized, flexible energy grids. Nuclear energy is adapting to this transformation in several ways.
a. Microreactors and Distributed Energy Generation
Microreactors (tiny nuclear power plants) offer a new approach to energy distribution.
- Key Advantages of Microreactors:
- Can be deployed in remote locations, military bases, and disaster-stricken areas.
- Require minimal maintenance and can run for decades without refueling.
- Companies like Oklo, Westinghouse, and Rolls-Royce are developing microreactor designs for off-grid applications.
b. Hybrid Nuclear-Renewable Energy Systems
Nuclear power can work alongside solar, wind, and battery storage to create a stable and resilient energy grid.
- Flexible Nuclear Operations:
- Advanced reactors can adjust power output to balance fluctuations from wind and solar energy.
- Helps reduce reliance on fossil fuel backup power (like natural gas).
- Nuclear-Powered Energy Storage Solutions:
- Excess electricity from nuclear plants can be used to charge batteries or produce hydrogen, acting as energy storage for renewable integration.
- High-temperature reactors can store thermal energy in molten salts, which can be released on demand to generate electricity.
A combined nuclear-renewable grid could ensure both sustainability and energy security without over-reliance on fossil fuels.
11. Nuclear Energy and Global Geopolitics
Nuclear energy plays a strategic role in international relations and energy security. Countries with strong nuclear programs gain economic, political, and technological advantages.
a. China and Russia’s Nuclear Expansion Strategies
- China:
- Aims to become the world’s nuclear leader by building 150 new reactors by 2050.
- Developing exportable SMR technology to supply nuclear power to developing nations.
- Russia:
- The largest global exporter of nuclear technology, building nuclear plants in over 30 countries.
- Leads in floating nuclear reactors, deploying them in remote Arctic regions.
b. The U.S. and Europe’s Nuclear Renaissance
- United States:
- Investing in advanced reactors, microreactors, and SMRs to maintain global leadership in nuclear technology.
- The Biden administration’s clean energy policies include nuclear energy incentives and research funding.
- European Union:
- The EU is reconsidering its nuclear policies, with countries like France, Poland, and Finland expanding nuclear energy, while Germany and Austria remain opposed.
c. Nuclear Energy’s Role in Energy Security
- Countries are diversifying energy sources to reduce dependence on fossil fuels from unstable regions.
- SMRs and microreactors could provide energy independence for small nations and energy-strained regions.
Geopolitically, nuclear power is not just an energy source—it’s a strategic asset shaping global power dynamics.
12. The Final Vision: A Nuclear-Powered Sustainable Future
If nuclear energy overcomes economic, safety, and regulatory challenges, it could lead the world into a new era of clean, abundant power.
Short-Term (2025-2035):
✅ SMRs and advanced reactors gain regulatory approval and widespread adoption.
✅ Nuclear-powered hydrogen production scales up for industrial applications.
✅ AI and automation improve nuclear safety and efficiency.
Mid-Term (2035-2050):
✅ Nuclear fusion reaches commercial viability, revolutionizing the energy industry.
✅ Hybrid nuclear-renewable energy grids become the norm.
✅ AI-powered microreactors enable off-grid, decentralized energy solutions.
Long-Term (2050 and Beyond):
✅ Nuclear-powered space missions to Mars and beyond.
✅ Fusion reactors power entire cities, eliminating the need for fossil fuels.
✅ Nuclear energy contributes to climate restoration efforts (e.g., carbon-negative nuclear processes).
Conclusion: The Dawn of a Nuclear Energy Revolution
Nuclear energy is entering a new golden age, fueled by breakthrough technologies, global climate goals, and shifting energy policies. If governments, industries, and scientists continue investing in innovation, nuclear power could become the dominant energy source of the 21st century, ensuring a sustainable, clean, and technologically advanced future for humanity. 🌍⚛️
13. Nuclear Energy’s Role in Climate Change Mitigation
As the world races to achieve net-zero emissions by mid-century, nuclear power is increasingly recognized as a vital component of climate change mitigation. Unlike fossil fuels, nuclear energy provides a constant, low-carbon energy source that can complement intermittent renewables.
a. Comparing Nuclear Power to Other Low-Carbon Energy Sources
While solar and wind are crucial for decarbonization, they have limitations:
- Intermittency – Solar and wind depend on weather conditions and require large-scale energy storage.
- Land Use – Wind and solar farms need hundreds of times more land than nuclear plants for the same energy output.
- Grid Stability – Without baseload power (like nuclear or hydro), grid reliability can suffer.
How Nuclear Complements Renewables
- Provides 24/7 clean power, reducing dependence on fossil fuel backup generation.
- Small land footprint allows nuclear plants to be built near cities without disrupting ecosystems.
- Stable electricity production prevents grid instability during periods of low wind or solar output.
Nuclear power, alongside renewables, can create a balanced, resilient, and carbon-free energy mix.
b. Nuclear Energy’s Role in Achieving Net-Zero by 2050
The Intergovernmental Panel on Climate Change (IPCC) and the International Energy Agency (IEA) have emphasized that reaching net-zero emissions will require a significant expansion of nuclear energy.
Key targets include:
✅ Doubling global nuclear capacity by 2050 to replace coal and gas.
✅ Developing SMRs to provide clean energy to remote areas and industrial hubs.
✅ Using nuclear reactors for hydrogen production, reducing emissions in hard-to-decarbonize industries.
Several countries have already committed to expanding nuclear power as part of their climate action plans:
- United States: $6 billion in funding to keep nuclear plants operational.
- European Union: Recognized nuclear as a green investment under its taxonomy rules.
- China: Plans to build 150 new reactors by 2050.
- Japan: Restarting reactors and exploring next-generation nuclear technologies.
With strategic policy decisions and sustained investment, nuclear energy could become a cornerstone of the global clean energy transition.
14. The Road to Fusion Energy: The Ultimate Clean Power Source
While today’s nuclear fission plants provide reliable, low-carbon energy, nuclear fusion could revolutionize the industry by offering limitless clean power with minimal waste and zero meltdown risk.
a. How Fusion Works
Unlike fission (which splits atoms), fusion combines hydrogen isotopes (deuterium & tritium) to release massive amounts of energy—the same process that powers the Sun and stars.
Fusion energy has several game-changing advantages:
✅ No greenhouse gas emissions – Completely carbon-free.
✅ Virtually unlimited fuel – Hydrogen is abundant in seawater.
✅ No long-lived nuclear waste – Unlike fission, fusion waste decays quickly.
✅ Inherent safety – No risk of meltdown, as fusion automatically stops if containment fails.
b. Major Fusion Projects and Breakthroughs
- International Thermonuclear Experimental Reactor (ITER)
- The world’s largest fusion experiment, based in France.
- Expected to produce 10x more energy than it consumes by 2035.
- U.S. National Ignition Facility (NIF)
- Achieved net energy gain in 2022, proving that fusion can generate more power than it consumes.
- A milestone toward commercial fusion reactors.
- Private Fusion Companies
- Commonwealth Fusion Systems (MIT spin-off): Developing magnetically confined fusion using high-temperature superconductors.
- Helion Energy & TAE Technologies: Working on compact, cost-effective fusion reactors for industrial power.
With trillions of dollars in potential economic impact, fusion energy could become the ultimate clean power source by the second half of the 21st century.
15. Nuclear Energy and the Circular Economy
As the world transitions to sustainable energy, nuclear technology is evolving to fit within a circular economy model, where waste is minimized, resources are reused, and sustainability is maximized.
a. Closing the Nuclear Fuel Cycle
Instead of discarding spent fuel, new reactor designs aim to recycle and reuse nuclear materials, reducing waste and increasing efficiency.
1. Fast Breeder Reactors (FBRs)
- Convert spent nuclear fuel into new fuel, reducing waste by up to 90%.
- Russia, France, and China are leading fast reactor development.
2. Thorium Fuel Cycle
- Uses thorium-232, which is more abundant and produces less long-lived waste than uranium.
- India and China are investing in thorium reactor technology.
b. Using Nuclear Heat for Industrial Decarbonization
Nuclear plants can generate high-temperature heat for industries that are difficult to electrify, such as:
🔥 Steel production – Replacing coal-fired blast furnaces.
🔥 Chemical manufacturing – Decarbonizing ammonia and fertilizer production.
🔥 Seawater desalination – Providing clean drinking water in arid regions.
By integrating nuclear power into multiple industries, the economy can become more sustainable and less dependent on fossil fuels.
16. Nuclear Power and Space Colonization
As space agencies and private companies plan long-term human missions to the Moon, Mars, and beyond, nuclear energy is emerging as the best solution for space power generation.
a. Lunar and Martian Colonies
- Solar power is unreliable on Mars due to dust storms and weak sunlight.
- NASA and private firms are designing small nuclear reactors to power future space bases.
- The Kilopower project is developing compact fission reactors for deep-space missions.
b. Nuclear-Powered Spacecraft
- Nuclear Thermal Propulsion (NTP):
- Can cut travel time to Mars in half by generating more thrust than chemical rockets.
- NASA and the U.S. military are developing NTP for future interplanetary missions.
- Radioisotope Thermoelectric Generators (RTGs):
- Already used in Voyager, Curiosity, and Perseverance rovers to provide long-lasting space power.
Nuclear propulsion and power systems will play a critical role in humanity’s expansion beyond Earth.
Final Thoughts: The Next Era of Nuclear Innovation
From carbon-free electricity to fusion breakthroughs, space exploration, and industrial decarbonization, nuclear energy is evolving into one of the most versatile and transformative technologies of the 21st century.
Key Takeaways:
✅ SMRs and microreactors will enable decentralized nuclear power.
✅ Advanced fuel cycles and reprocessing will minimize waste.
✅ Fusion energy will become viable by the late 21st century.
✅ Nuclear-powered hydrogen will decarbonize heavy industry.
✅ AI and automation will enhance nuclear safety and efficiency.
With global investment, innovation, and policy support, nuclear energy could soon power the world, drive space exploration, and secure a sustainable future for generations to come. 🚀⚛️
Nuclear microgrids provide stable electricity
Nuclear microgrids offer a reliable and continuous source of electricity, ensuring stability even in remote or off-grid locations. Unlike renewable sources that depend on weather conditions, nuclear microgrids generate power consistently, making them ideal for critical infrastructure, industrial sites, and military bases. Their ability to operate independently from centralized grids enhances energy security and resilience, reducing vulnerability to power disruptions. Advanced reactor designs used in these systems provide enhanced safety features, long operational lifespans, and minimal environmental impact. By integrating with renewable energy sources, nuclear microgrids contribute to a balanced and sustainable energy mix, supporting long-term energy independence.
Nuclear microgrids provide a continuous and highly stable electricity supply, making them essential for critical applications where energy security and reliability are paramount. Unlike traditional power grids, which rely on centralized infrastructure and are vulnerable to disruptions from natural disasters, cyberattacks, or supply chain issues, nuclear microgrids function independently, ensuring uninterrupted power generation. This independence is particularly beneficial for remote communities, military installations, space missions, and industrial operations that require a dependable energy source regardless of external conditions. Because nuclear microgrids operate using compact reactors designed for long-term use, they offer a distinct advantage over conventional diesel generators or intermittent renewable sources. While solar and wind power contribute to clean energy solutions, their dependency on weather conditions necessitates large-scale energy storage or backup systems, which can be costly and inefficient. In contrast, nuclear microgrids generate steady power without requiring constant refueling, as many advanced reactor designs can run for years or even decades before needing fuel replenishment. This extended operational capability makes them an attractive option for locations with limited access to fuel supplies or infrastructure for frequent maintenance.
Modern nuclear microgrids integrate advanced safety features, such as passive cooling systems, automated shutdown mechanisms, and containment designs that significantly reduce the risk of accidents. Unlike older nuclear power technology, these microreactors are designed to be inherently safe, meaning that they require little human intervention and can automatically stabilize in the event of operational anomalies. This makes them particularly useful for deployment in harsh or isolated environments where skilled personnel may not always be available for immediate oversight. Additionally, their modular nature allows for scalable energy production, meaning that multiple units can be deployed to match increasing energy demands. This flexibility makes them highly adaptable to a range of applications, from powering research stations in extreme environments to serving as backup power sources for hospitals and data centers in urban settings.
Another advantage of nuclear microgrids is their ability to integrate with renewable energy sources to form hybrid energy systems. While nuclear reactors provide a consistent baseline power supply, excess energy from wind or solar generation can be stored or utilized during peak production periods, optimizing overall system efficiency. This combination reduces dependency on fossil fuels while maintaining grid stability, making nuclear microgrids an essential component of sustainable energy strategies. Their ability to generate high-temperature heat also enables them to support industrial processes such as hydrogen production, desalination, and chemical manufacturing, further broadening their potential applications. As energy demand continues to grow and concerns over grid stability and climate change intensify, nuclear microgrids represent a forward-looking solution that combines resilience, efficiency, and sustainability. With continued advancements in reactor technology, regulatory support, and public investment, nuclear microgrids have the potential to revolutionize energy distribution, making clean and reliable power accessible to a wide range of industries and communities worldwide.
The development and deployment of nuclear microgrids are expected to accelerate as energy demands rise and the need for reliable, clean power sources becomes more critical. Unlike large-scale nuclear plants, which require extensive infrastructure and regulatory oversight, microreactors designed for microgrids can be manufactured in modular units and transported to their destinations with relative ease. This modularity allows for quicker deployment and lower upfront costs compared to traditional nuclear power stations. Once installed, these reactors can operate autonomously for extended periods without frequent refueling, reducing the logistical and economic burden of energy production in remote or off-grid areas.
One of the key advantages of nuclear microgrids is their ability to ensure energy resilience in regions prone to natural disasters or geopolitical instability. In many cases, centralized power grids are vulnerable to extreme weather events, cyberattacks, or supply chain disruptions that can lead to widespread blackouts. By decentralizing electricity generation, nuclear microgrids reduce dependency on fragile transmission networks and provide a self-sustaining energy solution that can keep essential services operational. Hospitals, military bases, research facilities, and disaster relief operations stand to benefit significantly from the uninterrupted power supply that nuclear microgrids offer, ensuring that critical infrastructure remains functional even when larger power systems fail.
The role of nuclear microgrids in industrial and commercial applications is also expanding as companies seek sustainable and cost-effective alternatives to fossil fuel-based energy sources. Industries such as mining, manufacturing, and oil and gas exploration often operate in locations where access to reliable electricity is limited or prohibitively expensive. Deploying nuclear microgrids in these settings can dramatically reduce reliance on diesel generators, which are costly to maintain and contribute to carbon emissions. Furthermore, by providing high-temperature heat, nuclear microgrids can support industrial processes that require continuous thermal energy, such as metal refining, cement production, and synthetic fuel manufacturing. This capability enhances the efficiency of industrial operations while simultaneously reducing their environmental impact.
In addition to terrestrial applications, nuclear microgrids are poised to play a crucial role in space exploration and off-world colonization. As agencies like NASA and private companies such as SpaceX and Blue Origin work toward establishing permanent human settlements on the Moon and Mars, the need for a reliable, long-term power source becomes increasingly evident. Solar energy, while a viable option in space, faces limitations due to long planetary nights, dust storms, and variable sunlight exposure. Nuclear microgrids provide a robust solution by offering continuous power generation, enabling habitats, life support systems, and scientific experiments to operate without interruption. The development of compact, transportable nuclear reactors for space missions could lay the foundation for self-sustaining extraterrestrial colonies and deep-space exploration missions.
As public and private sectors continue to invest in nuclear microgrid technology, advancements in reactor design, fuel efficiency, and safety mechanisms are making these systems more viable and attractive for widespread adoption. Research into advanced nuclear fuels, such as TRISO particles, and alternative reactor types, including molten salt and fast reactors, is paving the way for more efficient and resilient microreactors. These innovations are not only enhancing the safety and sustainability of nuclear energy but also addressing longstanding concerns about radioactive waste and proliferation risks. With increasing global efforts to transition toward low-carbon energy sources, nuclear microgrids have the potential to become a cornerstone of future energy systems, providing stable, scalable, and clean electricity in a rapidly evolving world.
The expansion of nuclear microgrids is expected to reshape energy infrastructure by introducing localized, independent power generation systems that can operate autonomously for years. This shift is particularly important for regions where energy security is a pressing concern, including areas with unstable grids, remote communities, and military installations requiring continuous operations. Unlike traditional centralized power grids that rely on long-distance transmission lines, nuclear microgrids generate electricity directly at the point of use, eliminating many of the vulnerabilities associated with power distribution. This self-sufficiency is a key advantage in ensuring resilience against extreme weather, cyber threats, and geopolitical disruptions that could otherwise compromise energy availability.
One of the most promising applications of nuclear microgrids is in disaster response and emergency preparedness. Natural disasters such as hurricanes, earthquakes, and wildfires frequently disrupt conventional power grids, leaving millions of people without electricity for extended periods. In such scenarios, mobile or rapidly deployable nuclear microgrids could serve as a reliable backup power source, restoring critical services such as hospitals, water purification systems, and communication networks. The ability of microreactors to operate independently, without the need for continuous fuel deliveries like diesel generators, makes them particularly well-suited for emergency situations where supply chains may be disrupted. Government agencies and humanitarian organizations are increasingly exploring nuclear microgrid technology as part of disaster resilience planning, recognizing its potential to provide long-term, sustainable power solutions in crisis zones.
In addition to emergency response, nuclear microgrids offer significant advantages for commercial and industrial operations in remote locations. Mining sites, offshore drilling platforms, and Arctic research stations are often dependent on costly and logistically challenging fuel shipments to sustain their power needs. By replacing diesel-powered generators with nuclear microgrids, these industries can achieve greater energy independence, lower operating costs, and significantly reduce their carbon footprint. In the Arctic, where extreme cold and long periods of darkness make renewable energy sources less effective, microreactors can provide a stable and continuous power supply, supporting scientific research, defense operations, and indigenous communities. This technology could also play a key role in powering future infrastructure projects in developing nations, where access to a stable electricity grid remains a major challenge for economic growth and modernization.
The development of advanced reactor designs further strengthens the case for nuclear microgrids. Innovations in fuel technology, such as high-assay low-enriched uranium (HALEU) and accident-tolerant fuels, enhance reactor safety and efficiency while minimizing waste production. Passive cooling systems and automated control mechanisms make modern microreactors inherently safe, reducing the likelihood of human error or mechanical failure. Many next-generation microreactors are also designed to be factory-built and easily transportable, allowing for rapid deployment in areas where electricity demand is growing. These improvements in nuclear technology make microgrids not only a practical solution for immediate energy needs but also a long-term investment in global energy stability and decarbonization.
As the world transitions toward a cleaner energy future, nuclear microgrids are increasingly recognized as a key component of hybrid energy systems. By integrating with renewable energy sources such as solar, wind, and hydroelectric power, nuclear microgrids can provide consistent baseline power, balancing the fluctuations inherent in intermittent generation methods. In many cases, excess electricity from nuclear microgrids can be used for energy-intensive applications such as hydrogen production, carbon capture, or battery storage, further enhancing grid flexibility and reducing reliance on fossil fuels. This synergy between nuclear and renewables is expected to play a critical role in achieving global climate goals while maintaining a stable and resilient energy supply.
Beyond their use in terrestrial applications, nuclear microgrids are poised to revolutionize power generation in space exploration. The harsh environments of the Moon and Mars require energy solutions that are both reliable and self-sustaining, as traditional solar power faces challenges such as dust storms and long lunar nights. NASA and private space companies are actively developing compact nuclear reactors to support lunar bases, space stations, and deep-space missions. These microreactors would provide power for life support systems, scientific experiments, and resource extraction, enabling long-term human presence beyond Earth. As space exploration advances, nuclear microgrids will likely become a foundational technology for sustaining human settlements on other planets, offering an energy solution that is not dependent on external fuel supplies or variable environmental conditions.
With ongoing advancements in reactor design, regulatory frameworks, and public acceptance, nuclear microgrids are well-positioned to become a mainstream energy solution in the coming decades. Governments and private investors are increasingly recognizing their potential to provide clean, resilient, and decentralized power to a wide range of applications. As these systems continue to evolve, their role in ensuring energy security, supporting economic development, and mitigating climate change will become even more significant. The future of nuclear microgrids is not just about providing electricity; it is about enabling a more sustainable, flexible, and technologically advanced energy landscape for generations to come.
As nuclear microgrids continue to gain traction, their impact on global energy infrastructure is expected to grow significantly, reshaping the way electricity is produced and distributed. The transition from large, centralized power plants to decentralized microgrid systems allows for greater adaptability in meeting local energy demands while minimizing transmission losses. This transformation is particularly important in regions with underdeveloped or aging energy infrastructure, where traditional power generation methods struggle to keep pace with rising demand. By deploying nuclear microgrids, these areas can leapfrog conventional grid expansion, establishing resilient and sustainable energy systems without the need for extensive transmission networks.
A key factor driving the adoption of nuclear microgrids is their ability to provide stable and continuous power, independent of external conditions. Unlike renewable sources such as wind and solar, which are subject to fluctuations in weather patterns, nuclear microgrids generate electricity consistently, ensuring a reliable energy supply for critical infrastructure. This reliability makes them particularly attractive for high-priority facilities such as data centers, medical research institutions, financial institutions, and telecommunications networks, where even brief power disruptions can lead to significant economic losses and security risks. The uninterrupted power supply provided by microreactors also supports the widespread adoption of electric vehicles, enabling the development of robust charging networks in remote or off-grid locations.
One of the most exciting prospects for nuclear microgrids is their role in industrial decarbonization. Many heavy industries, including steel, cement, and chemical manufacturing, rely on fossil fuels for high-temperature heat, which is essential for their production processes. Conventional renewable energy sources often struggle to provide the consistent heat required for these applications, whereas nuclear microgrids can deliver both electricity and industrial-grade thermal energy. By replacing coal and natural gas with advanced microreactors, industries can significantly reduce their carbon emissions while maintaining operational efficiency. Additionally, nuclear microgrids can facilitate the production of green hydrogen—a key component in future energy systems—by providing the high temperatures and electricity necessary for efficient electrolysis. This integration of nuclear technology into industrial processes presents a major opportunity to accelerate the global transition toward a low-carbon economy.
Another major advantage of nuclear microgrids is their scalability and modular design. Unlike traditional nuclear plants, which require years of construction and significant capital investment, microreactors are designed to be manufactured in a factory setting and transported to their destination, reducing both costs and deployment timelines. This modular approach allows communities, businesses, and governments to implement nuclear energy solutions incrementally, scaling up as demand increases. Furthermore, the ability to deploy multiple microgrids in parallel provides an additional layer of energy security, ensuring that if one unit requires maintenance, others can continue to operate without disruption. This scalability makes nuclear microgrids particularly well-suited for rapidly growing urban centers, military bases, and island nations seeking to reduce their reliance on imported fossil fuels.
The safety and environmental considerations associated with nuclear energy have long been points of debate, but modern microreactors are designed with cutting-edge safety features that significantly reduce risks. Many advanced designs incorporate passive cooling systems, fail-safe shutdown mechanisms, and meltdown-proof reactor cores, ensuring that they can operate safely with minimal human intervention. Furthermore, advancements in fuel technology are leading to the development of reactors that produce lower amounts of nuclear waste, with some designs even capable of recycling spent fuel for additional energy generation. These innovations are helping to shift public perception, demonstrating that nuclear microgrids can be a safe, clean, and efficient solution for meeting future energy demands.
As the geopolitical landscape continues to evolve, energy independence is becoming an increasingly important consideration for nations around the world. Many countries rely on imported fossil fuels, making them vulnerable to supply chain disruptions and price volatility. Nuclear microgrids offer a path toward greater energy sovereignty by providing a stable and domestically controlled power source. This is particularly relevant for regions with limited natural energy resources, where nuclear microgrids can serve as a long-term, strategic energy investment. Governments are beginning to recognize the potential of microreactors as a means of reducing reliance on foreign energy supplies while simultaneously advancing their climate commitments.
Looking ahead, the continued development of nuclear microgrids will depend on a combination of technological innovation, regulatory adaptation, and investment in research and development. As more nations prioritize clean energy solutions, the market for microreactors is expected to expand, driving further improvements in efficiency, affordability, and deployment speed. Collaboration between the public and private sectors will be essential in scaling up production and establishing the necessary infrastructure for widespread adoption. Additionally, international cooperation on safety standards and best practices will play a crucial role in ensuring that nuclear microgrids are deployed responsibly and effectively across diverse regions.
Ultimately, nuclear microgrids represent a transformative step toward a more resilient, decentralized, and sustainable energy future. Their ability to provide uninterrupted power, integrate with renewables, support industrial applications, and enhance energy security positions them as a key solution for the challenges of the 21st century. As advancements in reactor technology continue to accelerate, the potential applications for nuclear microgrids will only expand, opening new opportunities for innovation in fields ranging from space exploration to carbon-neutral cities. In the coming decades, nuclear microgrids will likely become an indispensable component of global energy systems, offering a reliable and long-lasting source of clean power for generations to come.
Decentralized power with micro nuclear tech
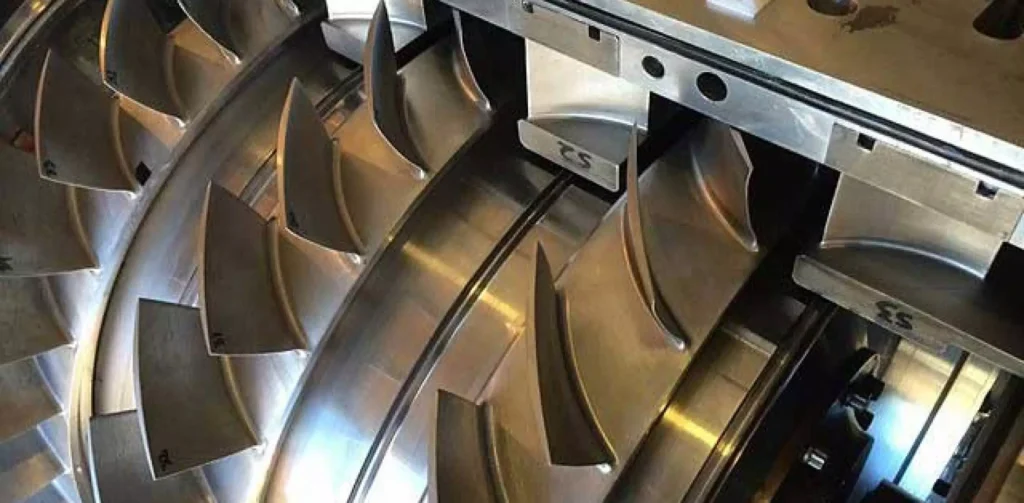
Decentralized power generation using micro nuclear technology is emerging as a transformative solution to modern energy challenges, offering reliable, resilient, and scalable electricity in a variety of settings. Unlike traditional large-scale nuclear power plants, which require extensive infrastructure and centralized control, micro nuclear reactors operate independently or in small networks, reducing dependence on vast transmission systems. This shift toward decentralized energy production enhances grid stability, mitigates the risk of widespread blackouts, and provides a consistent power source in areas where traditional energy infrastructure is limited or unreliable.
One of the key advantages of decentralized power generation with micro nuclear technology is its ability to provide energy security in remote or off-grid locations. Conventional power grids are vulnerable to natural disasters, cyberattacks, and geopolitical disruptions, often resulting in prolonged outages that impact critical services. By deploying small-scale nuclear reactors closer to the point of consumption, communities, industries, and military installations can maintain continuous operations without relying on distant power plants or fuel supply chains. This localized approach to energy generation is particularly valuable for isolated regions, research stations, island nations, and disaster-prone areas that require a resilient and autonomous power solution.
Micro nuclear reactors are designed to be modular and transportable, making them ideal for rapid deployment and scalability. Unlike large nuclear facilities that take years to construct, microreactors can be prefabricated in factories, shipped to their destination, and installed with minimal site preparation. This flexibility allows energy providers to quickly adapt to changing demand patterns, deploying additional reactors as needed without the financial and logistical burdens associated with expanding traditional power plants. Moreover, the compact nature of these reactors enables their integration into existing energy systems, allowing businesses and municipalities to supplement their power supply without overhauling their infrastructure.
Another significant benefit of decentralized nuclear power is its synergy with renewable energy sources. While solar and wind power are essential for reducing carbon emissions, their intermittency poses challenges for grid reliability. Micro nuclear reactors can provide a stable baseline power supply, ensuring that electricity remains available even when weather conditions limit renewable energy output. In hybrid energy systems, excess power from renewables can be stored in batteries or used for processes like hydrogen production, while nuclear reactors continue to supply a steady stream of electricity. This combination of technologies enhances overall energy resilience and accelerates the transition to a low-carbon economy.
The safety of micro nuclear technology has been a major focus of recent advancements, with modern designs incorporating fail-safe mechanisms, passive cooling systems, and meltdown-resistant fuel configurations. Unlike older nuclear power plants, which require active cooling and extensive human oversight, many microreactors are engineered for autonomous operation, significantly reducing the risk of accidents. Some advanced designs, such as molten salt and high-temperature gas-cooled reactors, further enhance safety by using fuel that remains stable under extreme conditions. These improvements have helped alleviate concerns about nuclear energy, making decentralized micro nuclear power a more viable option for widespread adoption.
In addition to electricity generation, micro nuclear technology has the potential to support a range of industrial applications that require high-temperature heat. Industries such as steel production, cement manufacturing, and chemical processing currently rely on fossil fuels to generate the heat needed for their operations. By integrating microreactors into these processes, companies can significantly reduce their carbon footprint while maintaining efficiency and productivity. Similarly, microreactors can be used for water desalination, district heating, and hydrogen production, providing versatile solutions to some of the world’s most pressing energy and environmental challenges.
The geopolitical implications of decentralized nuclear power are also significant, as nations seek to reduce their dependence on fossil fuel imports and strengthen their energy security. By investing in micro nuclear technology, countries can establish independent and resilient energy systems that are less susceptible to global supply chain disruptions. This decentralization of power generation not only enhances national security but also promotes economic growth by enabling industries and communities to thrive without concerns about energy shortages or price volatility.
As technological advancements continue to improve the efficiency, safety, and affordability of micro nuclear reactors, their adoption is expected to increase across a wide range of applications. Governments, private companies, and research institutions are investing heavily in developing next-generation microreactors that are more compact, cost-effective, and environmentally friendly. With ongoing regulatory support and public awareness initiatives, decentralized nuclear power is poised to become a cornerstone of modern energy systems, offering a sustainable and resilient solution to the challenges of the 21st century.
The continued advancement of decentralized power generation using micro nuclear technology is poised to revolutionize the global energy landscape, addressing critical issues such as energy security, climate change, and infrastructure resilience. As populations grow and energy demands increase, traditional centralized power grids are struggling to keep pace, particularly in developing regions and areas prone to extreme weather events. By integrating micro nuclear reactors into decentralized networks, energy providers can ensure stable and uninterrupted electricity supply, reducing dependency on aging and vulnerable transmission infrastructure.
One of the defining characteristics of micro nuclear technology is its ability to function as an autonomous power source with minimal external intervention. Many of these reactors are designed for long-term operation without the need for frequent refueling, in some cases running for decades before requiring maintenance. This feature makes them particularly suitable for locations where energy logistics are challenging, such as remote military bases, mining operations, and space missions. Unlike fossil fuel-powered generators, which require continuous fuel deliveries, microreactors offer a self-sustaining solution that significantly lowers operational costs and environmental impact.
In urban environments, decentralized nuclear power can be integrated into microgrids, providing a reliable source of electricity that operates independently from the larger grid. This is especially valuable in regions that experience frequent power outages due to grid congestion, extreme weather, or cyber threats. Cities looking to modernize their infrastructure can use micro nuclear reactors to create local energy hubs that ensure critical services—such as hospitals, emergency response centers, and water treatment facilities—remain functional even during grid failures. These self-sufficient energy systems can be particularly beneficial for disaster preparedness, reducing the economic and human costs associated with prolonged blackouts.
Decentralized nuclear energy also plays a crucial role in reducing carbon emissions and transitioning toward a sustainable energy future. While renewable sources like wind and solar are essential components of decarbonization, their variability presents challenges in maintaining a stable energy supply. Micro nuclear reactors complement renewables by providing a steady source of baseload power, balancing the fluctuations inherent in intermittent generation methods. In hybrid energy systems, nuclear reactors can supply consistent electricity when renewables are unavailable, ensuring continuous power availability while reducing reliance on fossil fuels. This integration allows for a more flexible and efficient energy mix, supporting global climate targets without compromising reliability.
Beyond electricity generation, micro nuclear technology has the potential to support a range of advanced energy applications that can drive economic growth and innovation. High-temperature microreactors can be used to produce hydrogen, a clean fuel that is expected to play a key role in future energy systems. Hydrogen produced through nuclear-powered electrolysis can serve as an alternative to fossil fuels in industries such as transportation, aviation, and manufacturing, further reducing greenhouse gas emissions. Additionally, nuclear microgrids can support energy-intensive processes such as desalination, which is essential for providing clean drinking water in arid regions and coastal cities.
The geopolitical and economic benefits of decentralized nuclear power are also becoming increasingly apparent. Many nations rely on imported fossil fuels to meet their energy needs, leaving them vulnerable to market fluctuations, supply chain disruptions, and geopolitical conflicts. By developing domestic nuclear microgrid infrastructure, countries can reduce their dependence on external energy sources and strengthen their national security. Furthermore, the deployment of microreactors in remote and underdeveloped regions can drive local economic development, providing reliable electricity to support businesses, education, and healthcare services.
The future of decentralized nuclear energy will be shaped by continued advancements in reactor design, regulatory frameworks, and public perception. Innovations in passive safety systems, advanced fuels, and modular construction techniques are making micro nuclear technology more viable and cost-effective for widespread deployment. At the same time, efforts to streamline regulatory approval processes and build public trust in nuclear energy will play a crucial role in determining the pace of adoption. Governments, private sector investors, and research institutions must work together to address these challenges and unlock the full potential of decentralized nuclear power.
As the world moves toward a more distributed and resilient energy model, micro nuclear technology is set to become a key pillar of the future energy system. Its ability to provide stable, low-carbon, and scalable electricity solutions makes it an ideal complement to existing renewable energy sources, ensuring that power remains available in all circumstances. Whether deployed in urban centers, remote locations, or off-world colonies, micro nuclear reactors have the potential to redefine how energy is produced and consumed, offering a sustainable and secure foundation for the next generation of power infrastructure.
The continued evolution of decentralized nuclear power is not only reshaping energy distribution but also fostering innovation in reactor technology, grid integration, and global energy policy. As governments and industries recognize the strategic advantages of micro nuclear reactors, investments in research and development are accelerating, paving the way for new reactor designs that are safer, more efficient, and more adaptable to various energy needs. The potential applications of these advanced reactors extend beyond traditional electricity generation, opening doors to breakthroughs in clean industrial processes, sustainable urban planning, and even space exploration.
One of the most promising developments in micro nuclear technology is the emergence of advanced reactor designs that enhance safety, reduce waste, and improve fuel efficiency. Many next-generation microreactors utilize high-assay low-enriched uranium (HALEU) or other innovative fuel types that allow for longer operational lifespans while minimizing the risk of proliferation. Some designs, such as molten salt and pebble bed reactors, offer inherent safety mechanisms that eliminate the possibility of catastrophic failures, making them well-suited for decentralized deployment. These passive safety features reduce the need for complex and expensive containment structures, making nuclear power more accessible to a wider range of industries and communities.
As micro nuclear technology becomes more viable, its integration with smart grid systems is also gaining momentum. Unlike traditional centralized power grids, which rely on large plants to supply electricity over vast transmission networks, smart grids use decentralized generation sources and digital technologies to balance supply and demand in real time. Microreactors fit seamlessly into this model by providing stable, always-available power that can be dynamically distributed based on local energy needs. In combination with battery storage and renewable energy sources, decentralized nuclear power can help create self-sustaining energy ecosystems that optimize efficiency and reliability while minimizing environmental impact.
A key advantage of decentralized nuclear power is its ability to strengthen infrastructure resilience in an era of increasing climate-related challenges. Natural disasters, cyberattacks, and geopolitical conflicts pose significant threats to traditional energy systems, often resulting in widespread blackouts that disrupt critical services. By deploying micro nuclear reactors as part of a decentralized network, communities and industries can maintain essential operations even in the face of grid failures. For example, hospitals, emergency response centers, and water treatment facilities can rely on on-site nuclear power to ensure uninterrupted service, reducing the human and economic toll of energy disruptions.
Beyond electricity production, micro nuclear technology is set to play a transformative role in decarbonizing industries that have historically been difficult to electrify. Heavy industrial sectors such as steel manufacturing, cement production, and chemical refining require high-temperature heat, which is traditionally generated using fossil fuels. Microreactors, particularly those designed for high-temperature applications, can provide a clean and consistent heat source for these processes, enabling industries to reduce their carbon footprint while maintaining efficiency. This capability is critical for achieving global climate goals, as industrial emissions account for a significant portion of total greenhouse gas output.
The role of decentralized nuclear power in hydrogen production is another area of growing interest. Green hydrogen, produced using renewable energy sources or nuclear power, is emerging as a key component of future energy systems due to its potential to replace fossil fuels in transportation, power generation, and industrial applications. Microreactors can be used to generate the high temperatures needed for efficient electrolysis, allowing for the production of clean hydrogen at scale. By integrating nuclear-powered hydrogen production into decentralized energy networks, nations can create a sustainable fuel source that reduces reliance on carbon-intensive alternatives.
The expansion of decentralized nuclear power also has significant geopolitical and economic implications. Many countries currently depend on imported oil, natural gas, or coal to meet their energy needs, making them vulnerable to supply chain disruptions and price volatility. By investing in micro nuclear reactors, nations can enhance their energy security and reduce their exposure to geopolitical risks. Furthermore, the localization of nuclear power generation fosters economic development by creating jobs in reactor manufacturing, maintenance, and fuel processing. This shift toward domestic energy independence can strengthen national economies while reducing reliance on fossil fuel exports.
Looking toward the future, the regulatory landscape will play a critical role in determining how quickly decentralized nuclear power can be adopted on a global scale. Historically, nuclear energy has been subject to strict regulations due to safety concerns, which has slowed innovation and increased development costs. However, as microreactors are designed with enhanced safety features and passive shutdown mechanisms, regulatory agencies are beginning to explore more streamlined approval processes for small-scale nuclear deployments. The development of international standards for microreactor certification and operation will be essential for scaling up deployment while ensuring consistent safety protocols across different regions.
Public perception of nuclear energy will also influence the widespread adoption of micro nuclear technology. Despite its many advantages, nuclear power has faced skepticism due to past accidents and concerns about radioactive waste disposal. To address these concerns, education and outreach efforts will be crucial in highlighting the safety advancements and environmental benefits of modern microreactors. Transparent communication about waste management strategies, safety protocols, and the long-term sustainability of nuclear power can help build public trust and encourage broader acceptance of decentralized nuclear energy.
As technological advancements continue, decentralized nuclear power is likely to play an increasingly important role in shaping the future of energy. From powering remote communities and industrial facilities to supporting renewable energy integration and reducing global carbon emissions, micro nuclear reactors offer a versatile and resilient solution for modern energy challenges. With continued investment, policy support, and public engagement, the transition toward a decentralized nuclear-powered future is well within reach, paving the way for a cleaner, more reliable, and more secure global energy system.
The trajectory of decentralized nuclear power is set to redefine the global energy framework by enhancing sustainability, security, and adaptability. As micro nuclear reactors continue to develop, their potential to revolutionize both urban and remote energy systems grows stronger. These small-scale reactors offer a unique combination of stability, efficiency, and low environmental impact, making them an ideal complement to renewables and a key driver of global decarbonization efforts.
One of the most significant advantages of micro nuclear technology is its ability to function independently from large-scale power grids, reducing the vulnerabilities associated with centralized energy distribution. Traditional grids, while effective for large-scale power delivery, are susceptible to outages caused by natural disasters, cyberattacks, and aging infrastructure. By establishing localized energy generation with microreactors, communities, businesses, and critical facilities can achieve energy independence, ensuring uninterrupted power supply even in adverse conditions. This resilience is particularly valuable for regions with unstable energy infrastructure, where frequent blackouts hinder economic development and quality of life.
The modular nature of microreactors makes them well-suited for deployment in diverse environments, from dense urban centers to remote industrial sites. In cities, these compact reactors can be integrated into district energy systems, providing both electricity and heat for residential and commercial buildings. Their low-emission operation supports urban sustainability goals while reducing reliance on fossil fuel-based heating systems. In industrial settings, microreactors can be co-located with manufacturing plants, data centers, and desalination facilities, supplying consistent power without the need for extensive fuel transportation and storage. This self-sufficiency is particularly beneficial in isolated areas where energy logistics present a major challenge.
Furthermore, the potential for micro nuclear technology extends beyond Earth’s surface. Space agencies and private companies exploring extraterrestrial colonization are increasingly looking at small nuclear reactors as a reliable power source for lunar and Martian habitats. Solar power, while useful in space, is subject to limitations such as dust storms on Mars and long periods of darkness at the Moon’s poles. Microreactors, on the other hand, can operate continuously, providing the necessary energy for life support systems, scientific research, and in-situ resource utilization. The development of space-ready nuclear reactors could also lead to advancements that benefit terrestrial applications, improving reactor safety, efficiency, and miniaturization.
Another critical aspect of decentralized nuclear energy is its role in facilitating energy equity. Many developing regions lack access to reliable electricity, limiting economic growth, healthcare, and education. Deploying micro nuclear reactors in these areas can provide a stable and sustainable power source, reducing dependence on costly and polluting diesel generators. Unlike large-scale nuclear plants that require extensive infrastructure and regulatory approval, microreactors can be deployed faster and with fewer logistical hurdles. Their long operational lifespans and minimal fuel requirements further enhance their viability as a long-term solution for energy-poor regions.
The intersection of nuclear microgrids with digitalization and artificial intelligence (AI) is also shaping the future of decentralized power. AI-driven monitoring systems can optimize reactor performance, predict maintenance needs, and enhance safety by detecting anomalies before they escalate into issues. Advanced grid management software can integrate microreactors with other energy sources, dynamically balancing supply and demand while reducing waste. These intelligent systems make decentralized nuclear power even more efficient, ensuring maximum reliability with minimal human intervention.
As the global push for decarbonization intensifies, micro nuclear reactors offer a path forward for hard-to-abate sectors that cannot easily transition to intermittent renewable energy. The transportation industry, for example, could benefit from nuclear-powered charging stations for electric vehicles, reducing strain on existing grids while supporting widespread EV adoption. Similarly, ports and shipping industries could utilize micro nuclear reactors to generate clean power for docked vessels, eliminating the need for diesel-powered auxiliary engines and significantly reducing maritime emissions.
Despite their immense potential, the widespread adoption of micro nuclear reactors will depend on overcoming economic and regulatory challenges. While technological advancements have made these reactors more cost-effective, initial investment costs remain high. Governments and private investors must collaborate to develop funding models that support early-stage deployments and incentivize long-term adoption. Streamlining regulatory approval processes while maintaining rigorous safety standards will also be crucial in accelerating deployment. International cooperation in setting clear guidelines for the construction, operation, and decommissioning of microreactors will help standardize best practices and ensure widespread acceptance.
Public perception remains another key factor in the future of decentralized nuclear power. Decades of misinformation and concerns about nuclear accidents have contributed to skepticism toward the technology. Transparent communication, education initiatives, and real-world demonstrations of microreactor safety and efficiency will be essential in addressing these concerns. Showcasing successful case studies where microreactors have provided reliable, clean energy with minimal risk will help shift public opinion and pave the way for broader adoption.
Looking ahead, the continued integration of micro nuclear technology into decentralized energy systems will play a critical role in building a more resilient and sustainable global energy network. Whether powering cities, industries, remote locations, or even future space colonies, microreactors provide a stable, low-carbon, and scalable energy solution that aligns with the world’s growing need for cleaner and more reliable power sources. By addressing key technical, economic, and regulatory challenges, decentralized nuclear power has the potential to redefine the way energy is generated and distributed, ensuring a more secure and sustainable future for generations to come.
Micro nuclear energy for industrial use
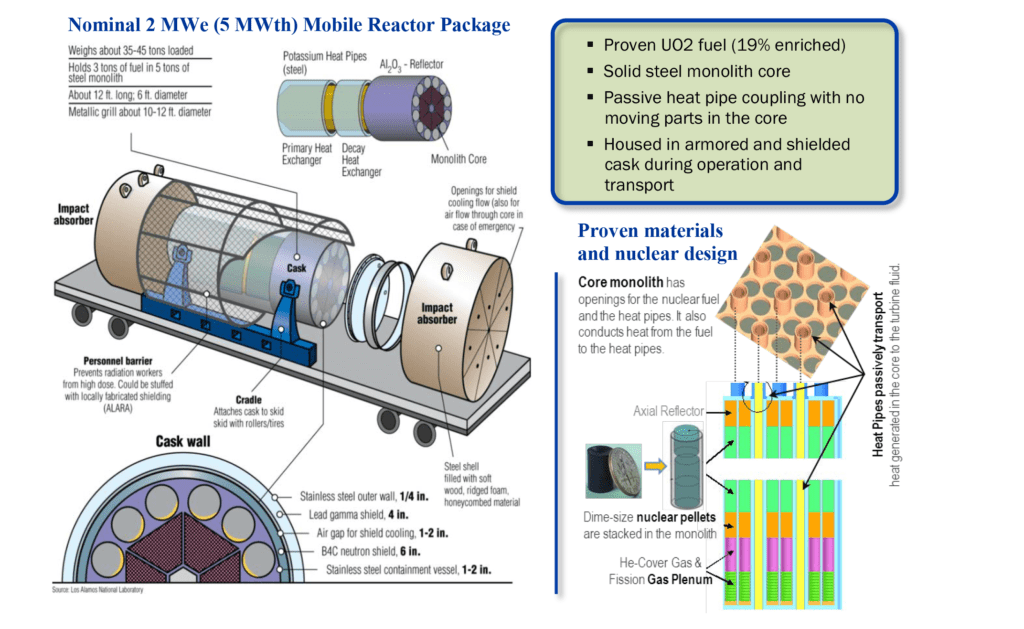
Micro nuclear energy is poised to revolutionize industrial power consumption by providing a stable, low-carbon, and high-efficiency energy source tailored to meet the intensive demands of various sectors. Industries that rely on continuous, high-energy output—such as manufacturing, mining, chemical processing, and steel production—require a dependable power supply that traditional renewables struggle to provide due to intermittency issues. Micro nuclear reactors (MNRs) offer a scalable solution, capable of delivering consistent heat and electricity while significantly reducing reliance on fossil fuels.
One of the primary advantages of micro nuclear energy for industrial use is its ability to supply both electricity and high-temperature process heat. Many industrial processes require temperatures that exceed what renewable sources like wind and solar can efficiently generate. MNRs, particularly high-temperature gas-cooled reactors (HTGRs), can operate at temperatures exceeding 700°C, making them suitable for applications such as hydrogen production, ammonia synthesis, and petrochemical refining. By integrating MNRs into these sectors, industries can achieve greater energy efficiency while drastically cutting carbon emissions.
The mining sector, which often operates in remote locations with limited access to reliable energy infrastructure, stands to benefit significantly from micro nuclear deployment. Diesel generators, which are commonly used to power mining operations, are costly to operate and contribute heavily to greenhouse gas emissions. MNRs can provide a long-term, uninterrupted energy source, reducing fuel transportation costs and improving overall operational sustainability. This shift not only enhances energy security for mining companies but also aligns with global sustainability goals aimed at reducing industrial carbon footprints.
Similarly, the steel and cement industries, which are among the largest contributors to global CO₂ emissions, require consistent and high-temperature heat for production processes. Electrification using intermittent renewables is challenging in these sectors, making micro nuclear an attractive alternative. Advanced microreactor designs can be integrated into steel mills to provide direct heat and electricity, reducing the need for coal-based blast furnaces. This transition is crucial in achieving net-zero targets, as conventional steelmaking accounts for approximately 8% of global CO₂ emissions.
Micro nuclear energy can also play a transformative role in industrial hydrogen production. Green hydrogen, which is produced using electrolysis powered by renewables, is often limited by fluctuating energy availability. Nuclear-powered hydrogen production, however, offers continuous operation, improving efficiency and reducing costs. High-temperature microreactors can facilitate thermochemical water-splitting processes, which require less electricity than conventional electrolysis, further optimizing hydrogen production. Given the growing demand for hydrogen in fuel cell technology and industrial applications, nuclear-powered hydrogen production presents a viable path toward large-scale adoption.
Beyond energy-intensive industries, micro nuclear technology has the potential to enhance the resilience and sustainability of industrial parks and manufacturing hubs. Many industrial zones rely on centralized grid connections that are susceptible to outages and price fluctuations. By deploying microreactors as part of a localized energy strategy, companies can establish energy-independent operations, protecting themselves from supply chain disruptions and volatile energy markets. This decentralized approach strengthens business continuity while supporting economic growth through reliable power availability.
Another key advantage of micro nuclear reactors for industrial use is their modular and scalable nature. Unlike traditional large nuclear plants, MNRs can be constructed off-site and transported to industrial locations, significantly reducing installation times and costs. Modular designs also allow companies to scale their energy capacity according to demand, making nuclear power more accessible and cost-effective for industrial applications. As regulatory frameworks evolve to accommodate small-scale reactors, industries will benefit from streamlined approval processes, further accelerating adoption.
Despite these advantages, widespread deployment of micro nuclear energy in industry faces certain challenges. High initial capital costs, regulatory hurdles, and public perception concerns remain key barriers to adoption. However, as advanced reactor designs become more cost-efficient and policymakers develop supportive regulatory frameworks, the path toward industrial-scale micro nuclear energy is becoming increasingly viable. Collaborations between governments, energy providers, and industrial leaders will be essential in driving forward pilot projects and demonstrating the long-term benefits of this technology.
In the coming decades, micro nuclear energy is set to play an integral role in industrial decarbonization efforts. By providing stable, emission-free energy for heavy industries, mining operations, and hydrogen production, MNRs offer a practical solution to one of the biggest challenges in global energy transition. With continued advancements in reactor technology, safety enhancements, and policy support, micro nuclear power will serve as a cornerstone of sustainable industrial development, enabling economic growth while achieving ambitious carbon reduction targets.
The expansion of micro nuclear energy in industrial applications will not only enhance operational efficiency but also reshape the global energy landscape by providing a reliable and sustainable alternative to fossil fuels. As industries continue to seek viable pathways to decarbonization, microreactors are emerging as a key solution capable of meeting energy-intensive demands while maintaining environmental responsibility.
One of the most compelling benefits of micro nuclear energy is its ability to deliver continuous baseload power. Unlike solar and wind energy, which are dependent on weather conditions and require extensive energy storage solutions, microreactors operate independently of external environmental factors. This uninterrupted energy supply is critical for industrial processes that require 24/7 operation, such as aluminum smelting, glass manufacturing, and chemical processing. Even minor disruptions in power can lead to significant financial losses in these sectors, making the reliability of microreactors a highly attractive feature.
Moreover, the integration of micro nuclear reactors into industrial operations allows for greater energy self-sufficiency. Instead of relying on distant power plants and vulnerable transmission lines, industries can deploy on-site nuclear power solutions that eliminate the risks associated with grid instability and energy price volatility. This shift to decentralized nuclear energy also mitigates the risk of supply chain disruptions, ensuring that industries maintain stable production even during periods of geopolitical tension or resource shortages.
Industries that rely heavily on steam and high-temperature heat for their processes will particularly benefit from micro nuclear energy. In petroleum refining, for example, the distillation and cracking of crude oil require vast amounts of heat, traditionally provided by burning natural gas or coal. Microreactors can supply the necessary thermal energy without the associated carbon emissions, significantly reducing the environmental footprint of oil and gas processing. Similarly, in paper and pulp manufacturing, where steam is used for drying and chemical treatment, nuclear heat can replace fossil fuel combustion, making the process cleaner and more sustainable.
Microreactors also offer a practical solution for remote industrial sites that struggle with energy access. Mining operations in Arctic regions, deserts, or deep underground often face logistical challenges when transporting diesel fuel for power generation. Deploying microreactors in these areas eliminates the need for continuous fuel shipments, reducing both costs and environmental risks associated with fuel spills and emissions. A single microreactor can operate for years without refueling, providing long-term energy security for these isolated facilities.
The ability of micro nuclear energy to support industrial electrification and hybrid energy systems further enhances its role in decarbonization. As industries transition from fossil fuels to electric-powered machinery, the demand for clean electricity will continue to rise. Microreactors can be seamlessly integrated with other low-carbon energy sources, such as wind and solar, to create hybrid energy systems that maximize efficiency and sustainability. By balancing renewable energy fluctuations with a constant nuclear power supply, industrial facilities can optimize their energy consumption while reducing their reliance on conventional power grids.
As technological advancements improve the efficiency and safety of microreactors, their economic viability is also increasing. Next-generation reactor designs incorporate passive safety features, advanced fuel cycles, and simplified maintenance protocols, reducing both operational risks and long-term costs. The development of factory-built, modular reactors allows for mass production and standardization, further driving down capital expenditures and making micro nuclear energy more accessible to a wider range of industries.
However, for micro nuclear energy to reach its full potential in industrial applications, regulatory frameworks must evolve to support faster deployment. Traditional nuclear regulations, designed for large-scale reactors, can be overly complex and time-consuming for small modular reactors. Policymakers and industry leaders must work together to establish streamlined approval processes that ensure safety while enabling the rapid adoption of micro nuclear technology. Additionally, financial incentives, such as tax credits and public-private partnerships, will be instrumental in accelerating initial investments and demonstrating the feasibility of industrial microreactor deployment.
Public perception will also play a critical role in shaping the future of micro nuclear energy. While nuclear power has historically faced opposition due to concerns about radiation, waste disposal, and past accidents, the latest reactor designs incorporate fail-safe mechanisms that significantly enhance safety. Educating stakeholders and the general public about the benefits of micro nuclear energy—including its role in reducing carbon emissions and supporting energy security—will be essential in overcoming resistance and driving widespread acceptance.
Looking ahead, micro nuclear energy is set to become a cornerstone of industrial power generation, offering a clean, reliable, and scalable alternative to traditional fossil fuels. As industries embrace this innovative technology, the global energy transition will gain momentum, bringing us closer to a sustainable, low-carbon future. By leveraging the full potential of micro nuclear reactors, industrial sectors can achieve unprecedented levels of efficiency, resilience, and environmental responsibility, ensuring long-term economic and ecological stability.
The deployment of micro nuclear reactors (MNRs) in industrial settings is not just an alternative energy solution but a transformative shift in how industries approach power generation and consumption. The ability of MNRs to provide consistent, high-density energy in a compact and modular format opens new opportunities for industrial operations that were previously constrained by unreliable grid access, fuel transportation challenges, or high carbon footprints.
As industries face increasing pressure to decarbonize, the demand for reliable clean energy solutions continues to grow. Microreactors offer a unique advantage by addressing one of the key challenges in industrial decarbonization: the need for high-temperature heat. Many renewable energy sources struggle to deliver heat at the levels required for heavy industrial processes such as cement production, glass manufacturing, and chemical synthesis. Micro nuclear reactors, particularly high-temperature gas-cooled reactors (HTGRs) and molten salt reactors (MSRs), can generate heat at temperatures exceeding 700°C, making them ideal for industrial applications that require continuous thermal energy.
Beyond heat production, microreactors enable the direct electrification of industries that have traditionally relied on fossil fuels. For example, aluminum smelting, a highly energy-intensive process, requires large amounts of electricity to extract aluminum from bauxite ore. Historically, coal-fired power plants have supplied this electricity, contributing to significant carbon emissions. By switching to MNRs as an energy source, aluminum producers can reduce their reliance on fossil fuels while maintaining the stable power supply necessary for efficient operations. This shift aligns with global efforts to reduce industrial emissions and meet net-zero targets.
Micro nuclear technology also enhances industrial resilience by mitigating the risks associated with energy supply chain disruptions. Many industries rely on imported fossil fuels, making them vulnerable to price fluctuations, geopolitical instability, and logistical constraints. By producing energy on-site with microreactors, companies can achieve greater energy independence, reducing costs associated with fuel procurement and transportation. This self-sufficiency is particularly valuable in industries with remote operations, such as mining, where fuel transportation challenges can significantly impact profitability.
Another key area where micro nuclear energy is poised to make an impact is hydrogen production. Hydrogen is increasingly seen as a critical component of the clean energy transition, with applications in transportation, industrial heating, and energy storage. However, conventional hydrogen production methods, such as steam methane reforming (SMR), are highly carbon-intensive. Green hydrogen, produced through electrolysis powered by renewable energy, offers a cleaner alternative but faces challenges due to the intermittency of wind and solar power. Microreactors provide a solution by enabling continuous hydrogen production through either high-temperature electrolysis or thermochemical water splitting. This consistent, carbon-free hydrogen supply could accelerate the adoption of hydrogen as an industrial fuel, further reducing reliance on fossil fuels.
The modular nature of MNRs offers another advantage: scalability. Unlike traditional large-scale nuclear plants, which require significant upfront investment and long construction timelines, microreactors can be deployed incrementally, allowing industries to scale their energy capacity based on demand. This flexibility makes nuclear power more accessible to a broader range of industries, from small manufacturing facilities to large-scale industrial hubs. Additionally, the ability to co-locate microreactors with industrial facilities reduces transmission losses and infrastructure costs, improving overall efficiency.
As microreactor technology advances, integration with digital and AI-driven energy management systems will further enhance industrial efficiency. Smart grid technologies and real-time monitoring systems can optimize reactor performance, predict maintenance needs, and adjust power output based on fluctuating industrial demand. These innovations will make micro nuclear energy even more cost-effective and reliable, ensuring that industries can maximize productivity while minimizing energy waste.
However, despite these advantages, the widespread adoption of micro nuclear reactors in industry will require overcoming several hurdles. One of the most significant challenges is regulatory approval. Current nuclear regulations are primarily designed for large-scale reactors, which means that adapting them for microreactors will require regulatory agencies to develop new frameworks that balance safety with efficiency. Governments and industry stakeholders must collaborate to create streamlined licensing processes that facilitate faster deployment without compromising safety standards.
Financing is another critical factor that will determine the pace of MNR adoption. While microreactors have the potential to be cost-effective in the long term, the initial capital investment can be substantial. To encourage industrial adoption, financial incentives such as tax credits, low-interest loans, and public-private partnerships will be essential. Governments that prioritize energy security and industrial decarbonization may offer subsidies to companies investing in micro nuclear technology, accelerating deployment and reducing reliance on carbon-intensive energy sources.
Public perception of nuclear energy also plays a crucial role in determining its industrial future. While advanced reactor designs incorporate enhanced safety features, negative associations with past nuclear incidents continue to influence public opinion. Transparent communication about the benefits, safety mechanisms, and environmental advantages of micro nuclear energy will be necessary to build public and investor confidence. Demonstration projects showcasing the successful implementation of MNRs in industrial settings can help shift perceptions and drive wider acceptance.
Looking ahead, micro nuclear energy is set to become a cornerstone of industrial power solutions. By providing a stable, low-carbon, and highly efficient energy source, MNRs will enable industries to achieve energy security, reduce emissions, and enhance operational efficiency. As technological advancements drive down costs and regulatory frameworks evolve to support deployment, microreactors will play a crucial role in reshaping industrial energy consumption. The next decade will be critical in determining how quickly this transformation occurs, but the potential for micro nuclear energy to drive industrial decarbonization is undeniable.
The long-term potential of micro nuclear energy in industrial applications extends beyond just providing clean and reliable power; it represents a fundamental shift in how industries approach sustainability, energy security, and operational resilience. As more companies and governments recognize the strategic value of micro nuclear reactors (MNRs), their adoption is expected to accelerate, reshaping global industrial energy systems in ways that were previously unimaginable.
One of the most promising aspects of micro nuclear technology is its ability to function as the backbone of industrial microgrids. Traditionally, large-scale industries have depended on centralized power plants, which require extensive transmission infrastructure and are vulnerable to outages, price volatility, and supply disruptions. By integrating microreactors into industrial complexes, businesses can create self-sufficient microgrids that provide a stable and localized power supply. These microgrids can be further enhanced by incorporating battery storage and intermittent renewable sources, creating hybrid energy systems that optimize efficiency while minimizing environmental impact.
For industries operating in regions with unstable energy infrastructures, microreactors offer a crucial advantage. Many emerging economies struggle with power shortages and grid instability, which can hinder industrial growth and economic development. Deploying MNRs in these regions can provide industries with an independent energy source that supports long-term expansion while reducing reliance on imported fossil fuels. This is particularly relevant for energy-intensive sectors such as steel production, chemical manufacturing, and data centers, where uninterrupted power is essential for continuous operations.
The role of micro nuclear energy in industrial electrification is another critical factor driving its adoption. As industries move away from traditional combustion-based processes toward electrified alternatives, the demand for reliable electricity will continue to rise. Microreactors can provide a consistent power supply for electrified industrial machinery, conveyor systems, and automated manufacturing processes. This transition not only improves energy efficiency but also significantly reduces greenhouse gas emissions, helping industries meet stringent environmental regulations and corporate sustainability goals.
Moreover, micro nuclear energy aligns well with the growing trend of corporate energy independence. Large industrial corporations are increasingly looking for ways to secure their own energy supply to avoid exposure to market fluctuations and regulatory uncertainties. By investing in on-site microreactors, companies can lock in long-term energy costs, making financial planning more predictable while avoiding the risks associated with fossil fuel price spikes. This is particularly relevant for industries with high operational costs, such as aluminum smelting, cement production, and semiconductor manufacturing.
Another transformative application of micro nuclear technology is its potential to revolutionize district heating for industrial clusters. Many industrial parks require both electricity and process heat, which is traditionally supplied through centralized fossil fuel-based plants. Microreactors, particularly high-temperature designs, can provide a carbon-free alternative by delivering direct heat for industrial applications while simultaneously generating electricity. This dual-function capability makes MNRs an attractive option for integrated industrial systems seeking to maximize energy efficiency.
Additionally, micro nuclear reactors can play a key role in decarbonizing heavy transport and logistics operations. Industries that rely on extensive transportation networks, such as shipping, rail, and aviation, can benefit from nuclear-powered hydrogen production. As global markets shift toward hydrogen-powered transportation, industries with access to dedicated hydrogen production from microreactors will gain a competitive edge in sustainable logistics. This shift could significantly reduce emissions from industrial freight operations, which currently account for a substantial portion of global carbon output.
The modular and transportable nature of MNRs also opens the door for rapid deployment in crisis-response scenarios. In the aftermath of natural disasters, industrial supply chains often face disruptions due to power outages and fuel shortages. Portable microreactors could be deployed to restore energy supply in affected areas, ensuring that critical industrial operations—such as food processing, medical supply manufacturing, and emergency logistics—remain functional. This capability makes micro nuclear energy not only a long-term industrial solution but also a valuable asset for disaster resilience and recovery efforts.
Despite the numerous advantages of micro nuclear energy, its widespread industrial adoption will depend on continued advancements in reactor design, fuel technology, and regulatory adaptation. Next-generation microreactors are expected to incorporate even more efficient fuel cycles, further extending operational lifespans and reducing waste. Advanced reactor designs, such as molten salt and fast reactors, could significantly enhance performance while reducing the complexity of fuel reprocessing and storage. These improvements will help address lingering concerns about nuclear waste and safety, making MNRs an even more attractive option for industrial deployment.
Furthermore, collaboration between governments, private industry, and research institutions will be essential in driving innovation and streamlining regulatory approval processes. Policymakers must develop frameworks that facilitate faster licensing and deployment of microreactors while maintaining rigorous safety standards. Public-private partnerships will play a crucial role in funding early projects and demonstrating the viability of micro nuclear energy in real-world industrial settings. As pilot programs successfully showcase the benefits of MNRs, confidence in their scalability and economic feasibility will grow, paving the way for widespread adoption.
In the coming decades, micro nuclear energy is poised to become a cornerstone of industrial energy strategies worldwide. By offering a scalable, low-carbon, and resilient power solution, MNRs will help industries transition toward cleaner operations while maintaining competitive advantage in an increasingly sustainability-driven market. As technology continues to evolve and regulatory landscapes adapt, micro nuclear power will not only redefine industrial energy consumption but also contribute to global efforts in combating climate change and securing a more stable energy future.
Steam turbines play a crucial role in modular nuclear units by converting the thermal energy generated from nuclear fission into mechanical and, ultimately, electrical energy. The efficiency, reliability, and compact design of steam turbines make them a key component in ensuring the successful operation of modular nuclear reactors (MNRs) and small modular reactors (SMRs).
The Role of Steam Turbines in Modular Nuclear Units
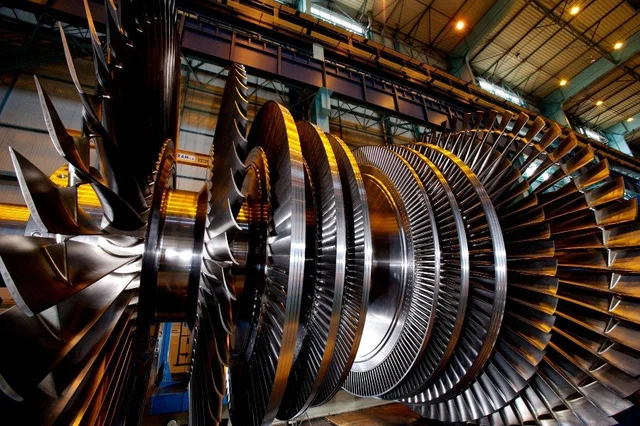
In modular nuclear units, nuclear fission generates intense heat, which is transferred to a coolant—typically water or a gas such as helium or carbon dioxide. In water-cooled reactors, this heat turns water into high-pressure steam, which is directed through a steam turbine. The turbine’s rotating blades extract energy from the steam, converting thermal energy into mechanical energy. This rotational motion drives an electrical generator, producing power for industrial use, grids, or decentralized applications.
Unlike traditional large-scale nuclear plants, which often use massive turbine-generator sets, modular nuclear units require compact, high-efficiency steam turbines that match their lower power output. These turbines are designed for quick deployment, lower maintenance, and compatibility with varying reactor designs, from pressurized water reactors (PWRs) and boiling water reactors (BWRs) to advanced high-temperature reactors (HTRs) and molten salt reactors (MSRs).
Key Features of Steam Turbines in Modular Nuclear Reactors
- Compact and Scalable Design
- Steam turbines in modular reactors are typically smaller and designed for scalability. They can be optimized for power outputs ranging from 10 MW to 300 MW, depending on the reactor’s thermal power and application.
- These turbines are often pre-fabricated and assembled as part of the modular construction approach, reducing installation time and costs.
- High Thermal Efficiency
- Modern steam turbines used in SMRs incorporate advanced blade design and materials to maximize efficiency while operating under lower pressures and temperatures compared to large nuclear power plants.
- Superheated steam cycles and reheat systems can be employed to enhance thermal efficiency, ensuring maximum energy extraction from the steam.
- Integration with Advanced Coolants
- While traditional steam turbines rely on water-cooled reactor designs (PWR, BWR), modular reactors using gas or molten salt coolants can incorporate indirect steam generation loops.
- High-temperature reactors (HTRs) using helium coolant can transfer heat to a secondary steam cycle, allowing steam turbines to operate at elevated efficiencies.
- In molten salt reactors, a heat exchanger transfers thermal energy to a secondary water loop, producing steam that drives the turbine without direct contact with the reactor core.
- Flexible Load-Following Capabilities
- Modular reactors are designed for flexible operation, making steam turbines in these units capable of adjusting power output based on demand.
- Load-following capabilities are essential for integrating SMRs into hybrid energy systems that combine nuclear with renewable sources such as wind and solar.
- Simplified Maintenance and Longer Lifespan
- Steam turbines in modular nuclear units benefit from advanced coatings, high-performance materials, and digital monitoring systems to reduce wear and tear.
- Many designs incorporate predictive maintenance technologies, reducing downtime and ensuring continuous operation.
Applications of Steam Turbines in Modular Nuclear Units
- Grid-Connected Power Generation: SMRs equipped with steam turbines provide stable, baseload electricity to national or regional grids. Their modular nature allows for phased deployment, enhancing grid flexibility.
- Industrial Power and Heat: Steam turbines in modular nuclear units are well-suited for industrial applications requiring both electricity and high-temperature process heat, such as hydrogen production, steel manufacturing, and chemical processing.
- Remote and Off-Grid Applications: In isolated regions, microreactors with steam turbines supply reliable power to military bases, mining operations, and remote communities without relying on fossil fuels.
- Hybrid Energy Systems: Modular nuclear units with steam turbines can operate alongside renewable energy sources, balancing intermittency by providing steady, dispatchable power.
Future Developments in Steam Turbines for Modular Nuclear Units
- Supercritical CO₂ Turbines: Some advanced modular reactors aim to replace traditional steam turbines with supercritical carbon dioxide (sCO₂) turbines, which offer higher efficiencies and reduced physical size.
- Digital Twins and AI Optimization: The integration of digital twin technology and AI-driven performance monitoring will optimize turbine operation, improving efficiency and extending component life.
- Improved Materials for Higher Temperatures: The development of advanced alloys and ceramic components will enable steam turbines to handle higher temperatures and pressures, increasing overall energy output.
As modular nuclear reactors continue to gain traction in the energy sector, steam turbines will remain a crucial technology for converting nuclear heat into usable power. Their ability to operate efficiently, reliably, and flexibly within SMR frameworks makes them an integral part of the future nuclear energy landscape.
The evolution of steam turbines for modular nuclear units is driven by the need for higher efficiency, compact designs, and better adaptability to various reactor types. As the energy sector transitions toward decentralized and flexible power generation, steam turbine technology in modular nuclear reactors is being refined to meet the demands of both grid-connected and off-grid applications.
Enhancing Steam Cycle Efficiency in Modular Nuclear Units
One of the key advancements in modular nuclear energy systems is the improvement of the Rankine steam cycle, which governs how thermal energy is converted into mechanical work through steam turbines. Traditional large-scale nuclear plants operate at relatively moderate steam conditions—typically with steam temperatures around 280-320°C and pressures of 7-8 MPa—due to material limitations. However, in modular reactors, efforts are being made to push these limits by incorporating advanced superheated steam cycles, reheating stages, and improved turbine blade designs to extract more energy from the steam.
For high-temperature modular reactors such as molten salt reactors (MSRs) and high-temperature gas-cooled reactors (HTGRs), the potential exists to generate steam at much higher temperatures—up to 600°C or more. These conditions significantly improve thermal efficiency, increasing the power output per unit of thermal energy produced. To harness these higher temperatures, steam turbines must be constructed using advanced materials such as nickel-based superalloys, which can withstand greater thermal stress while maintaining durability over long operational periods.
Compact and Modular Turbine Designs
Unlike conventional large nuclear turbines, which are designed for gigawatt-scale plants, steam turbines in modular reactors are optimized for smaller power outputs, typically in the range of 10 MW to 300 MW. These turbines need to be compact, easy to transport, and suitable for factory fabrication.
The shift towards modular turbine-generator sets allows for a streamlined manufacturing process where turbines are built in standardized units that can be quickly deployed and assembled on-site. This approach reduces construction time and costs while ensuring consistent quality and performance. Additionally, the modularity of steam turbines enables a phased deployment strategy, where multiple small turbines can be added incrementally to increase capacity as energy demand grows.
Steam Turbine Integration with Next-Generation Nuclear Reactors
Different types of modular reactors require different steam turbine configurations based on their coolant and thermal output characteristics.
- Pressurized Water Reactors (PWRs) in SMRs
- Many SMRs, including designs from NuScale and Rolls-Royce, are based on scaled-down versions of traditional PWR technology. These reactors generate steam indirectly through a heat exchanger.
- The steam produced is directed to conventional low-pressure steam turbines, which have been adapted for smaller-scale applications while maintaining high efficiency.
- Boiling Water Reactors (BWRs) in SMRs
- Some modular designs use BWR technology, where steam is generated directly in the reactor core and fed into the turbine.
- This simplifies the design by eliminating the need for steam generators and secondary loops, though it requires turbines to handle steam with trace amounts of radioactive particles.
- High-Temperature Gas-Cooled Reactors (HTGRs)
- HTGRs use helium or another inert gas as the primary coolant and can reach higher operating temperatures than water-cooled reactors.
- In systems where steam turbines are used, heat exchangers transfer thermal energy from the helium to a secondary steam cycle, which then powers a turbine.
- Molten Salt Reactors (MSRs)
- MSRs operate with liquid fluoride or chloride salts as coolants and can achieve very high thermal efficiencies.
- Like HTGRs, MSRs use a heat exchanger to produce steam for turbine operation, ensuring that no radioactive materials enter the steam cycle.
Load-Following and Flexibility for Industrial and Grid Applications
A key requirement for modern energy systems is flexibility. While traditional nuclear power plants are designed for continuous baseload power generation, modular nuclear units must adapt to variable energy demands. This is particularly important in hybrid energy systems that incorporate renewables, such as wind and solar, which can cause fluctuations in electricity supply.
To address this, steam turbines in modular reactors are designed with improved load-following capabilities, allowing them to adjust power output in response to grid demand. This is achieved through:
- Throttling and bypass systems: Adjusting steam flow to the turbine to control power generation.
- Multi-stage turbines: Allowing partial steam admission to optimize efficiency at varying loads.
- Fast startup and shutdown capabilities: Enabling modular reactors to respond quickly to changing power needs.
For industrial applications, particularly those requiring process heat, modular reactors with steam turbines offer the advantage of co-generation—simultaneously producing both electricity and heat. Industries such as chemical processing, hydrogen production, and district heating can benefit from this dual-function capability, reducing overall energy costs and emissions.
Digitalization and Advanced Monitoring in Steam Turbines
The next generation of steam turbines in modular nuclear units is also benefiting from advancements in digital monitoring and automation. AI-powered predictive maintenance systems use sensors and real-time data analysis to detect potential issues before they lead to failures, reducing downtime and maintenance costs.
By integrating digital twin technology—a virtual representation of the physical turbine—engineers can simulate performance, optimize operational parameters, and predict wear on critical components. This enhances reliability and efficiency, ensuring that modular nuclear units operate at peak performance throughout their lifecycle.
Future Developments in Steam Turbine Technology for Modular Nuclear Units
The future of steam turbines in modular nuclear reactors is shaped by several emerging innovations:
- Supercritical and Ultra-Supercritical Steam Cycles: Advancements in materials science may enable the adoption of supercritical (SC) and ultra-supercritical (USC) steam cycles in small modular reactors, significantly improving thermal efficiency.
- Supercritical CO₂ Turbines: Some advanced nuclear designs aim to replace traditional steam turbines with supercritical CO₂ (sCO₂) turbines, which operate at higher efficiencies and require a much smaller footprint.
- Hybrid Energy Systems: Future modular nuclear units could integrate steam turbines with renewable energy sources, using surplus steam for energy storage or district heating applications.
As modular nuclear technology continues to evolve, steam turbines will remain a critical component in maximizing energy conversion efficiency. Their adaptability, reliability, and ability to integrate with both existing and next-generation nuclear reactor designs ensure that they will play a central role in the future of nuclear energy.
Optimizing Steam Turbine Performance in Modular Nuclear Units
The performance of steam turbines in modular nuclear reactors (MNRs) is continually being optimized to improve efficiency, reliability, and integration with advanced reactor technologies. As MNRs become a key component in the transition to clean energy, steam turbines must adapt to new operational demands, emerging fuel cycles, and innovative thermal management systems.
One of the major design considerations for steam turbines in modular reactors is optimizing the thermodynamic cycle to maximize power output while minimizing thermal losses. Traditional Rankine cycles, which are commonly used in large nuclear power plants, can be improved in modular reactors by incorporating superheated and reheated steam cycles. These enhancements help extract more energy from the steam before it is condensed and recycled back into the reactor’s heat exchanger.
For next-generation small modular reactors (SMRs) operating at higher temperatures—such as molten salt reactors (MSRs) and high-temperature gas-cooled reactors (HTGRs)—there is an opportunity to develop more advanced steam cycles. Higher operating temperatures enable greater thermal efficiencies, which translate to improved overall power generation. This requires the use of turbine materials that can withstand higher thermal and mechanical stresses, such as nickel-based superalloys, ceramics, and composite materials designed for extreme environments.
Advanced Steam Cycle Configurations for Modular Reactors
- Reheat Steam Cycles:
- In a reheat cycle, steam exiting the high-pressure turbine is sent back to the heat exchanger for reheating before entering the intermediate-pressure turbine.
- This increases efficiency by reducing moisture content in the steam, preventing turbine blade erosion, and improving energy extraction.
- Some modular reactor designs, especially those intended for industrial applications, integrate reheat cycles to improve both electricity and process heat generation.
- Regenerative Steam Cycles:
- Regenerative feedwater heating improves cycle efficiency by using extracted steam from turbine stages to preheat the feedwater before it re-enters the reactor’s heat exchanger.
- This reduces the energy required to turn water into steam, thereby improving the overall thermal efficiency of the system.
- Supercritical and Ultra-Supercritical Steam Cycles:
- While traditional nuclear plants typically operate with subcritical steam conditions, some advanced modular reactors are exploring supercritical steam cycles, which operate at pressures above 22.1 MPa and temperatures above 600°C.
- Ultra-supercritical (USC) steam cycles push these limits even further, offering the potential for significantly higher efficiencies and lower emissions.
Material Innovations for High-Performance Steam Turbines
The ability of steam turbines to handle high temperatures and pressures in modular nuclear units depends on advanced materials that enhance durability, thermal resistance, and corrosion resistance. Key developments include:
- Nickel-Based Superalloys: These alloys are commonly used in aerospace and high-performance turbine applications and are now being adapted for next-generation nuclear steam turbines. Their ability to maintain strength at high temperatures makes them ideal for advanced reactors.
- Ceramic Matrix Composites (CMCs): CMCs offer high resistance to thermal stress and corrosion, making them suitable for turbine blades exposed to extreme conditions in modular reactors.
- Coatings and Surface Treatments: Advanced coatings, such as thermal barrier coatings (TBCs) and oxidation-resistant layers, improve turbine longevity by reducing wear and thermal degradation.
Modularization and Prefabrication of Steam Turbines
A significant advantage of steam turbines in modular nuclear units is their ability to be prefabricated and delivered as pre-assembled modules. This modular approach reduces installation time, minimizes on-site construction costs, and enhances standardization across different reactor designs.
- Factory Fabrication: Steam turbine modules can be manufactured in controlled environments, ensuring high precision and quality.
- Rapid Deployment: Prefabricated turbine-generator sets can be transported to remote locations or industrial sites, making them ideal for off-grid applications.
- Scalability: Modular turbines can be deployed in a phased approach, allowing operators to gradually increase power output as energy demand grows.
Integration of Digital Technologies for Steam Turbine Optimization
The use of digital monitoring and control systems is transforming steam turbine operations in modular nuclear units. Advanced analytics, artificial intelligence (AI), and predictive maintenance tools improve efficiency and reliability by:
- Real-Time Performance Monitoring: Sensors collect data on temperature, pressure, vibration, and steam flow, enabling operators to optimize turbine efficiency dynamically.
- AI-Based Predictive Maintenance: Machine learning algorithms analyze operational data to predict potential failures before they occur, reducing unplanned downtime and maintenance costs.
- Digital Twin Technology: Virtual models of steam turbines allow engineers to simulate performance, test operational adjustments, and identify areas for efficiency improvements.
Load-Following Capabilities for Flexible Power Generation
Unlike traditional large nuclear plants designed for steady baseload operation, modular nuclear units with steam turbines must be capable of flexible power output to meet changing electricity demands. This is especially important for hybrid energy systems that integrate nuclear power with renewable sources such as wind and solar.
Steam turbines in modular reactors incorporate several features to enhance load-following capabilities:
- Variable Steam Admission: Adjusting the amount of steam entering the turbine enables rapid power output changes without compromising efficiency.
- Partial Load Operation: Turbines can operate efficiently at reduced loads, allowing modular reactors to ramp power up or down based on demand.
- Fast Startup and Shutdown: Some SMRs and microreactors are designed for quick deployment and rapid response to fluctuating grid conditions.
Applications of Steam Turbines in Modular Nuclear Units
Steam turbines in modular reactors support a wide range of applications beyond traditional grid power generation:
- Industrial Energy Supply: Many manufacturing and chemical processing plants require both electricity and high-temperature process heat. Steam turbines in modular nuclear units provide combined heat and power (CHP), improving energy efficiency.
- Hydrogen Production: High-temperature modular reactors can generate steam for hydrogen production via electrolysis or thermochemical processes, supporting the transition to clean fuels.
- Desalination: Coastal and arid regions benefit from modular reactors with steam turbines supplying both power and steam for desalination plants.
- Remote and Off-Grid Power: Microreactors with steam turbines provide reliable energy for remote military bases, mining operations, and research stations.
Future Innovations in Steam Turbine Design for Nuclear Applications
As nuclear technology advances, steam turbines will continue to evolve to maximize efficiency and adaptability in modular reactor systems. Future developments may include:
- Supercritical CO₂ Power Cycles: Some next-generation modular reactors are exploring supercritical carbon dioxide (sCO₂) cycles instead of steam cycles, offering higher efficiency and reduced turbine size.
- Hybrid Nuclear-Renewable Systems: Steam turbines may be integrated with energy storage systems, allowing modular reactors to store excess energy for peak demand periods.
- Autonomous Operation and AI Control: Fully automated steam turbine control systems could optimize performance without human intervention, further improving efficiency and reliability.
Conclusion
Steam turbines remain a fundamental component of modular nuclear energy systems, offering a reliable and efficient means of converting nuclear heat into electricity. Advances in turbine materials, cycle efficiency, digital monitoring, and flexible operation are driving improvements in performance, making steam turbines well-suited for next-generation nuclear reactors. As modular nuclear technology gains traction in industrial, grid, and off-grid applications, the role of steam turbines will continue to expand, contributing to the broader transition toward clean and resilient energy systems.
Advanced Steam Turbine Configurations for Modular Nuclear Units
The ongoing development of steam turbines for modular nuclear reactors (MNRs) is shaping the next generation of nuclear power plants by enhancing efficiency, flexibility, and adaptability. With modular reactor designs ranging from small modular reactors (SMRs) to microreactors and next-generation high-temperature reactors, steam turbine systems must evolve to meet various operational requirements while maintaining reliability and cost-effectiveness.
One of the primary goals of modern modular steam turbines is to extract maximum energy from the available thermal output of nuclear reactors. Since MNRs operate at different temperature and pressure conditions depending on their design, steam turbine configurations must be optimized to ensure effective power conversion. This includes the incorporation of advanced steam cycles, innovative turbine blade geometries, and new thermodynamic processes that minimize energy losses.
Hybrid Steam Cycles for Enhanced Efficiency
Steam turbines in modular reactors can leverage hybrid cycle configurations to maximize efficiency and output:
- Reheat Cycles: By reheating steam between turbine stages, energy extraction is improved, reducing moisture content in the low-pressure section and preventing turbine blade degradation.
- Combined Cycles: Some modular reactors may integrate steam turbines with supercritical CO₂ (sCO₂) or gas turbines for increased thermal efficiency. This hybrid approach can lead to efficiencies exceeding 50%, a significant improvement over conventional nuclear power plants.
- Organic Rankine Cycle (ORC) Integration: For lower-temperature applications, such as microreactors, ORC turbines utilizing organic fluids can provide efficient electricity generation at reduced thermal input levels.
Material Science Innovations for High-Performance Steam Turbines
The ability of modular nuclear steam turbines to operate at higher efficiencies relies on advancements in turbine blade and rotor materials:
- Advanced Alloys: Nickel-based superalloys provide exceptional high-temperature strength, corrosion resistance, and durability under thermal cycling conditions.
- Titanium and High-Strength Stainless Steels: Used in low-pressure turbine sections to resist corrosion and fatigue from moisture-laden steam.
- Thermal Barrier Coatings (TBCs): Ceramic-based coatings protect turbine components from extreme heat, enabling prolonged operation at elevated temperatures.
- Additive Manufacturing (3D Printing): Allows for the creation of complex turbine blade geometries with optimized aerodynamics and reduced material waste.
Scalability and Modularity in Steam Turbine Design
One of the key advantages of MNRs is their scalable nature, which extends to the steam turbine systems. This modular approach offers several benefits:
- Factory-Built Turbines: Prefabricated turbine modules can be manufactured in a controlled environment, improving quality and reducing on-site assembly time.
- Stackable Power Modules: Steam turbines can be installed in parallel configurations, allowing for incremental power generation expansion as demand increases.
- Containerized Turbine Systems: Microreactor applications may utilize containerized steam turbines, enabling rapid deployment in remote or off-grid locations.
Dynamic Load Management for Grid Stability
Unlike traditional nuclear plants that operate at steady baseload output, MNRs with steam turbines must adapt to variable energy demand in modern grids:
- Variable Steam Admission Technology: Adjusts steam flow to maintain efficiency across different power output levels.
- Fast Ramping Capability: Enables turbines to quickly respond to fluctuations in grid demand, making them suitable for hybrid nuclear-renewable energy systems.
- Cogeneration and District Heating: Excess thermal energy from steam turbines can be used for industrial heating, desalination, or hydrogen production.
Steam Turbines in Microreactors for Industrial and Remote Power
Microreactors designed for decentralized energy applications require compact and efficient steam turbines to maximize their energy output:
- Industrial Heat and Power: Microreactors equipped with steam turbines can supply industrial facilities with both electricity and high-temperature steam for manufacturing processes.
- Remote Power Generation: Containerized steam turbine systems can be deployed in isolated areas, military bases, or Arctic research stations to provide reliable, long-term energy.
- Offshore and Marine Applications: Advanced microreactors could power maritime vessels, floating desalination plants, or offshore drilling platforms using steam turbines for efficient energy conversion.
Next-Generation Steam Turbine Innovations
As MNR technology continues to advance, steam turbine systems are expected to evolve with new capabilities:
- Supercritical Steam Cycles: Ultra-efficient turbines designed for next-generation reactors capable of producing superheated steam above 600°C.
- AI-Driven Performance Optimization: Machine learning algorithms continuously adjust turbine parameters for peak efficiency and predictive maintenance.
- Energy Storage Integration: Steam turbines in modular reactors may work in conjunction with thermal energy storage systems to provide dispatchable power on demand.
Conclusion
The role of steam turbines in modular nuclear reactors is expanding as nuclear power moves toward decentralized, flexible, and high-efficiency energy systems. With advancements in thermodynamic cycles, material science, modular construction, and digital monitoring, steam turbines will continue to be a critical component of next-generation nuclear energy. Whether in industrial co-generation, remote microgrids, or grid-scale hybrid power systems, modular nuclear steam turbines will remain at the forefront of sustainable and reliable energy production.
Efficient Power Generation from Micro Nuclear Plants
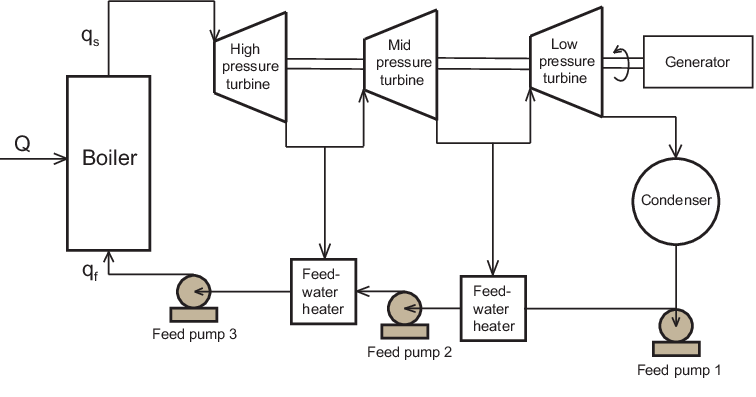
Micro nuclear plants are emerging as a highly efficient and reliable energy solution for decentralized power generation. These compact reactors, often designed for outputs ranging from a few megawatts to tens of megawatts, provide stable, low-carbon electricity with minimal fuel consumption and operational complexity. Their efficiency stems from advanced reactor designs, optimized thermodynamic cycles, and innovative energy conversion technologies.
Optimized Thermal Efficiency
Efficiency in micro nuclear plants is largely determined by the reactor’s thermal output and the effectiveness of the energy conversion system. Unlike conventional large-scale nuclear reactors, microreactors are designed for direct energy extraction with minimal losses, utilizing several key strategies:
- High-Temperature Operation: Some microreactors, particularly those based on high-temperature gas-cooled reactor (HTGR) technology, operate at temperatures exceeding 700°C. This allows for greater thermal efficiency when paired with Brayton or Rankine cycle turbines.
- Supercritical CO₂ (sCO₂) Power Cycles: Instead of traditional steam turbines, some microreactors use supercritical carbon dioxide as a working fluid. sCO₂ cycles achieve higher efficiency than steam cycles at smaller scales, improving power conversion while reducing system size.
- Advanced Heat Exchangers: Efficient thermal transfer is crucial in microreactors, and next-generation compact heat exchangers minimize energy losses while maintaining safety and reliability.
Load-Following and On-Demand Power
Micro nuclear plants are designed for flexible operation, making them well-suited for distributed power applications. Unlike traditional baseload nuclear power plants, microreactors offer:
- Rapid Startup and Shutdown: Many microreactors can achieve full power within hours or even minutes, allowing them to respond quickly to changing energy demands.
- Variable Power Output: Advanced control systems allow operators to adjust power levels dynamically, ensuring optimal efficiency under varying loads.
- Hybrid Integration with Renewables: Microreactors can complement solar and wind energy by providing stable backup power when renewable generation fluctuates.
Modular and Factory-Built Efficiency
One of the primary advantages of micro nuclear plants is their modular construction and prefabrication, which enhances efficiency in deployment and operation:
- Factory Fabrication: Components are manufactured in controlled environments, ensuring high precision and reducing on-site assembly time.
- Plug-and-Play Design: Many microreactors are delivered as fully assembled units that can be installed with minimal site preparation, cutting down installation costs and time.
- Minimal Cooling Requirements: Unlike large reactors that rely on extensive water cooling, some microreactors utilize air-cooled or passive cooling systems, further enhancing efficiency in remote and off-grid applications.
Applications of Efficient Micro Nuclear Power
Micro nuclear plants are being deployed across various sectors, demonstrating their efficiency in diverse applications:
- Industrial Power Supply: Many manufacturing and processing industries require both electricity and high-temperature heat. Microreactors provide combined heat and power (CHP), reducing energy waste and improving overall efficiency.
- Remote and Off-Grid Energy: Small communities, military bases, mining operations, and research facilities in isolated regions benefit from micro nuclear power by eliminating reliance on diesel generators.
- Hydrogen and Synthetic Fuel Production: High-temperature microreactors can drive efficient hydrogen production through electrolysis or thermochemical processes, supporting the clean fuel transition.
Future Prospects for Micro Nuclear Efficiency
Advancements in microreactor technology continue to push efficiency boundaries through:
- Next-Generation Fuel Designs: High-assay low-enriched uranium (HALEU) and TRISO fuel enable longer operational cycles with higher energy output.
- AI-Optimized Operation: Machine learning and digital twins improve efficiency by continuously monitoring performance and adjusting reactor parameters in real-time.
- Autonomous Operation and Maintenance: Many microreactors are designed for automated control, reducing the need for on-site personnel and improving operational reliability.
Conclusion
Micro nuclear plants are redefining efficient power generation by leveraging advanced reactor designs, high-performance energy conversion systems, and flexible deployment strategies. Their ability to provide clean, reliable, and scalable energy makes them an essential part of the future energy landscape, particularly in remote locations, industrial sectors, and hybrid renewable-nuclear power systems. As technology advances, micro nuclear plants will continue to improve in efficiency, ensuring their role in the transition to a more sustainable and resilient energy future.
Innovations Enhancing Efficiency in Micro Nuclear Plants
The evolution of micro nuclear plants is driven by advancements in reactor design, energy conversion systems, and operational flexibility. These small-scale nuclear reactors are designed to maximize energy output while minimizing resource consumption, making them an increasingly attractive solution for decentralized and efficient power generation. Their efficiency is further improved through innovative fuel technologies, advanced heat extraction methods, and integration with emerging energy systems.
High-Efficiency Reactor Core Designs
One of the primary factors influencing the efficiency of micro nuclear plants is the reactor core design. Several new approaches are being explored to optimize heat generation and transfer:
- TRISO Fuel Technology: Many next-generation microreactors use TRISO (Tristructural-Isotropic) fuel, which consists of uranium particles encased in multiple protective layers. This design enables higher fuel utilization, reduces waste production, and allows for operation at higher temperatures, improving thermal efficiency.
- Liquid Metal and Molten Salt Reactors: Some microreactors utilize liquid metals (such as sodium or lead) or molten salts as coolants instead of traditional water. These materials have superior heat transfer properties and can operate at higher temperatures, leading to improved thermodynamic efficiency.
- Compact Reactor Cores: Optimized fuel geometries and neutron reflectors enhance neutron economy, allowing for greater energy extraction from a smaller volume of fuel. This contributes to higher efficiency and longer operational life without refueling.
Advanced Power Conversion Technologies
Energy conversion plays a crucial role in determining the overall efficiency of micro nuclear plants. Traditional steam turbines, while effective, are being supplemented and, in some cases, replaced by more advanced power cycles:
- Supercritical CO₂ (sCO₂) Brayton Cycles: Compared to conventional Rankine cycles, sCO₂ cycles operate at higher efficiencies due to reduced thermodynamic losses. Their compact size and high power density make them particularly well-suited for micro nuclear plants.
- Direct Power Conversion: Some microreactors, particularly those using high-temperature designs, can integrate thermionic or thermoelectric generators for direct heat-to-electricity conversion, eliminating the need for moving mechanical parts and improving reliability.
- Microturbines and Stirling Engines: Alternative power conversion systems, such as microturbines or Stirling engines, are being explored for smaller-scale applications where efficiency and simplicity are prioritized.
Passive Cooling and Heat Management for Greater Efficiency
Efficient thermal management is critical for micro nuclear reactors to maintain optimal performance while ensuring safety. Innovations in passive cooling and heat rejection systems contribute significantly to overall efficiency:
- Air-Cooled and Passive Cooling Systems: Unlike conventional reactors that require large amounts of water for cooling, many microreactors utilize air-cooled heat exchangers or passive cooling mechanisms. This makes them ideal for remote locations where water availability is limited.
- Phase-Change Materials (PCMs): Some designs incorporate PCMs to store excess thermal energy and release it when needed, allowing for better load balancing and grid integration.
- Hybrid Heat Utilization: In some applications, waste heat from microreactors is captured and repurposed for industrial heating, desalination, or district heating, further improving overall system efficiency.
Scalability and Modular Deployment
One of the biggest advantages of micro nuclear plants is their scalability. Unlike traditional large reactors, which require extensive infrastructure and long construction times, microreactors are designed for modular deployment:
- Factory-Fabricated Units: Standardized microreactors are built in controlled environments, ensuring high quality and reducing on-site assembly time.
- Pre-Assembled Energy Modules: Many micro nuclear plants are delivered as complete, transportable modules that can be quickly installed, minimizing commissioning time and costs.
- Multi-Unit Configurations: In cases where additional power is needed, multiple microreactors can be deployed in parallel, providing an efficient way to scale energy production.
Integration with Hybrid Energy Systems
Micro nuclear plants are increasingly being integrated into hybrid energy systems, allowing them to work alongside renewable energy sources to enhance overall efficiency:
- Energy Storage Compatibility: Excess power generated by microreactors can be stored in batteries, thermal storage systems, or hydrogen production facilities for later use.
- Grid Stabilization and Load Balancing: Microreactors can provide consistent baseline power, compensating for the variability of wind and solar energy.
- Decentralized Power Networks: By serving as distributed energy nodes, micro nuclear plants contribute to the resilience and stability of modern smart grids.
Future Prospects for Even Greater Efficiency
As nuclear microreactor technology continues to evolve, several upcoming innovations promise to enhance efficiency further:
- Automated and AI-Driven Operation: Advanced control systems use artificial intelligence to optimize reactor performance in real time, reducing human intervention and improving efficiency.
- Advanced Fuel Recycling: New methods of fuel reprocessing and recycling may enable microreactors to extract even more energy from nuclear fuel, reducing waste and extending operational lifespans.
- Higher Temperature Reactors: Future designs aim to push operational temperatures even higher, enabling even more efficient power conversion methods, such as direct thermionic generation.
Conclusion
Micro nuclear plants are redefining efficient power generation by integrating high-performance reactor designs, cutting-edge energy conversion systems, and innovative thermal management strategies. Their ability to provide reliable, low-carbon energy in diverse applications—ranging from industrial power supply to remote and off-grid solutions—positions them as a key component of the future energy landscape. With continued advancements in materials, cooling technologies, and automation, micro nuclear plants will continue to push the boundaries of efficiency, supporting the global transition to sustainable and resilient energy systems.
Maximizing Efficiency in Micro Nuclear Plants
The drive toward efficiency in micro nuclear plants is shaping the future of decentralized energy production. These compact reactors, capable of operating in diverse environments, are being optimized to extract the maximum possible energy from nuclear fuel while minimizing operational costs and energy losses. By integrating cutting-edge fuel technologies, innovative heat transfer mechanisms, and advanced digital control systems, micro nuclear plants are setting new standards for reliable, efficient, and sustainable power generation.
Fuel Utilization and Extended Operational Life
One of the key aspects of efficient power generation in micro nuclear plants is fuel utilization. Modern microreactors employ advanced fuel types and configurations to extract more energy from a smaller fuel supply while extending reactor lifespans:
- High-Assay Low-Enriched Uranium (HALEU): HALEU fuel, enriched to around 19.75% U-235, offers higher energy density than conventional nuclear fuel, enabling microreactors to run longer without refueling.
- TRISO Particle Fuel: This highly robust fuel type can withstand extreme temperatures and radiation levels, improving reactor safety and efficiency while reducing the need for active cooling systems.
- Molten Salt Fuels: Some advanced microreactors use liquid fuel designs, such as molten salt reactors (MSRs), which provide continuous fuel replenishment and higher thermal efficiency due to better heat transfer properties.
- Long-Lived Core Designs: Many microreactors are designed for sealed operation, meaning they can function for years—sometimes decades—without refueling, drastically reducing maintenance costs and fuel-related downtime.
Innovative Heat Transfer and Energy Conversion Technologies
Efficient power generation in micro nuclear plants depends on optimizing heat transfer and energy conversion systems. Advanced thermodynamic cycles ensure that more of the reactor’s thermal energy is converted into usable electricity:
- Direct Heat-to-Electricity Conversion: Some microreactors utilize thermoelectric or thermionic energy conversion, bypassing traditional turbine-based systems and reducing mechanical inefficiencies.
- Supercritical CO₂ (sCO₂) Brayton Cycle: sCO₂ turbines operate at higher efficiencies than steam turbines, particularly in smaller-scale applications like microreactors. Their compact size, lower maintenance requirements, and higher efficiency make them ideal for micro nuclear power systems.
- Brayton and Rankine Hybrid Cycles: Some micro nuclear plants combine traditional steam turbines with Brayton cycle gas turbines to maximize efficiency across a range of operating temperatures.
- Passive and Advanced Heat Exchangers: High-performance compact heat exchangers improve thermal transfer efficiency, allowing reactors to generate more power while minimizing cooling requirements.
Autonomous Operation and AI Optimization
Artificial intelligence (AI) and digital automation are playing an increasingly important role in optimizing micro nuclear plant efficiency. Advanced digital systems can monitor and adjust reactor performance in real time, ensuring optimal operation under varying conditions:
- AI-Driven Load Adjustment: Machine learning algorithms can predict energy demand and adjust reactor output dynamically, ensuring optimal efficiency without unnecessary energy waste.
- Predictive Maintenance: AI-powered diagnostics monitor reactor components for early signs of wear, reducing unplanned downtime and improving long-term reliability.
- Automated Reactor Control: Many microreactors are designed for autonomous operation, eliminating the need for a constant on-site workforce and reducing operational costs.
- Digital Twins for Performance Optimization: Virtual models of microreactors can simulate different operational scenarios, allowing engineers to fine-tune efficiency parameters before making physical adjustments.
Hybrid Power Systems and Energy Storage Integration
Micro nuclear plants are increasingly being integrated with hybrid power systems to enhance overall efficiency. By working alongside renewable energy sources and energy storage technologies, microreactors can provide more stable and reliable power:
- Grid Balancing with Renewable Energy: Microreactors can serve as a stable backup to intermittent renewable sources like wind and solar, providing continuous power when renewable generation fluctuates.
- Thermal Energy Storage: Excess heat from the reactor can be stored in molten salts or phase-change materials, allowing energy to be released on demand for electricity generation or industrial heating.
- Hydrogen Production: High-temperature microreactors can efficiently generate hydrogen through thermochemical or high-temperature electrolysis processes, supporting the development of a hydrogen-based energy economy.
- Microgrid Compatibility: Micro nuclear plants can operate as part of decentralized microgrids, enhancing local energy resilience and reducing reliance on centralized power infrastructure.
Scalability and Deployment Flexibility
Micro nuclear plants offer unique advantages in terms of scalability and deployment speed. Unlike large nuclear power plants that require extensive construction time and regulatory approval, microreactors are designed for rapid deployment and modular scalability:
- Factory-Built and Transportable Units: Many micro nuclear plants are built in controlled factory environments, improving quality control and reducing on-site construction time. Some are designed as transportable units that can be shipped to remote locations.
- Plug-and-Play Installation: Standardized modular designs enable quick integration into existing energy infrastructures with minimal modification.
- Scalable Power Generation: Microreactors can be deployed as single units or in clusters, allowing for incremental expansion of power generation capacity based on demand.
- Rapid Deployment for Emergency and Remote Use: In disaster-stricken areas or remote locations, micro nuclear plants can provide reliable power where conventional energy sources are unavailable.
Long-Term Economic and Environmental Benefits
Efficiency improvements in micro nuclear plants translate into significant economic and environmental benefits:
- Lower Fuel Costs and Waste Production: High-efficiency fuel utilization reduces the frequency of refueling and minimizes nuclear waste generation.
- Reduced Carbon Emissions: By providing stable, emissions-free power, microreactors can replace fossil fuel-based generators in industrial and remote applications.
- Minimal Land and Resource Use: Micro nuclear plants require significantly less land compared to large-scale renewables, making them ideal for space-constrained environments.
- Enhanced Energy Security: Countries and industries that adopt micro nuclear plants can reduce dependence on imported fossil fuels, ensuring greater energy independence.
Future Directions in Micro Nuclear Efficiency
As micro nuclear technology continues to evolve, several new advancements promise even greater efficiency gains:
- Ultra-High Temperature Microreactors: Future designs aim to exceed 900°C operating temperatures, enabling highly efficient power conversion and expanded industrial applications.
- Fusion-Powered Microreactors: While still in early development, small-scale fusion reactors could provide even greater energy efficiency with virtually unlimited fuel supply and minimal waste.
- Closed-Loop Nuclear Fuel Cycles: Advanced recycling techniques may allow micro nuclear plants to reuse spent fuel, reducing waste and increasing sustainability.
- AI-Optimized Energy Distribution: Smart grid integration with AI-driven distribution systems will further enhance energy efficiency by ensuring power is delivered where and when it is needed most.
Conclusion
Micro nuclear plants are at the forefront of the next energy revolution, offering a combination of efficiency, flexibility, and sustainability that is unmatched by conventional power sources. Through advancements in fuel technology, energy conversion systems, autonomous operation, and hybrid energy integration, these reactors are achieving new levels of performance while reducing environmental impact and operational costs. As innovation continues, micro nuclear plants will play a crucial role in delivering efficient, resilient, and low-carbon energy solutions for a wide range of applications, from industrial power to remote communities and disaster relief efforts.
The Future of Ultra-Efficient Micro Nuclear Plants
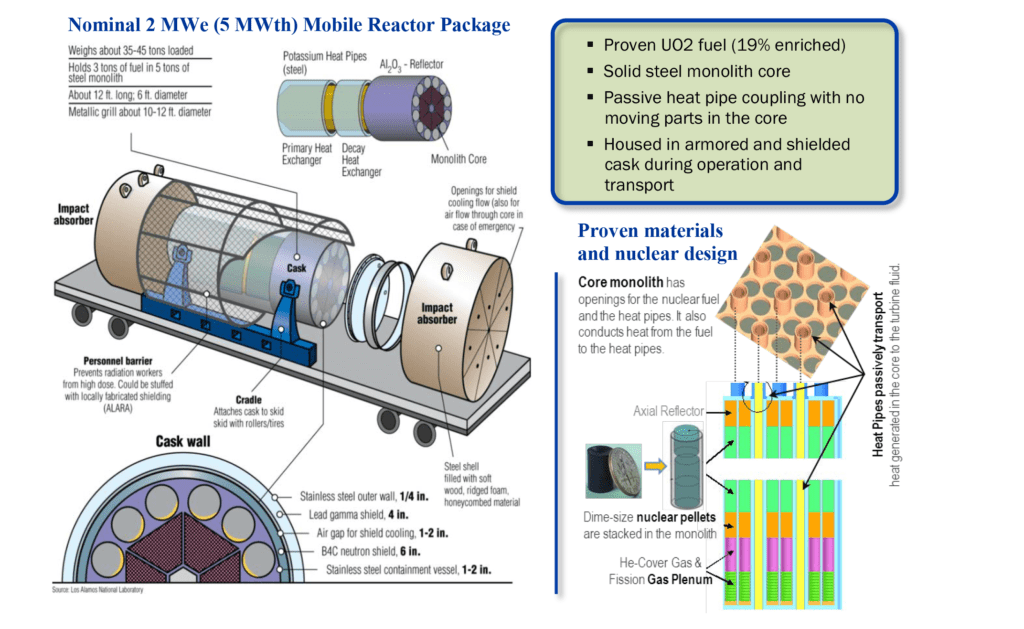
As the global energy landscape shifts toward cleaner and more resilient power sources, micro nuclear plants are emerging as a game-changing solution. Their ability to provide stable, high-efficiency power in a compact and scalable format makes them an attractive alternative to fossil fuels and a key enabler of decentralized energy systems. With continuous advancements in reactor technology, energy conversion efficiency, and system automation, micro nuclear plants are poised to redefine the future of power generation across industrial, remote, and hybrid energy applications.
Next-Generation Reactor Designs for Maximum Efficiency
One of the biggest drivers of efficiency in micro nuclear plants is the ongoing development of next-generation reactor designs that maximize heat extraction and minimize waste. Several advanced reactor technologies are pushing the boundaries of what small nuclear power systems can achieve:
- Fast Neutron Reactors (FNRs): Unlike conventional thermal reactors that use slow neutrons, fast reactors improve fuel utilization by burning nuclear waste and unused uranium, extending fuel life and reducing the need for refueling.
- High-Temperature Gas-Cooled Reactors (HTGRs): HTGRs use helium as a coolant, enabling reactor cores to operate at extremely high temperatures (>900°C) for superior energy conversion efficiency.
- Molten Salt Reactors (MSRs): MSRs operate with liquid fuel dissolved in molten salt, allowing for continuous fuel reprocessing and high thermal efficiency. Their inherent safety features also reduce operational risks.
- Micro Modular Reactors (MMRs): Small, factory-built reactors designed for sealed, self-contained operation—capable of running for decades without refueling, making them ideal for remote or off-grid applications.
Each of these reactor types is designed to optimize energy extraction, reduce fuel waste, and improve overall efficiency compared to traditional water-cooled reactors.
Breakthroughs in Energy Conversion for Higher Efficiency
While nuclear reactors generate immense thermal energy, the efficiency of a micro nuclear plant largely depends on how effectively it converts heat into electricity. To improve power output, advanced energy conversion technologies are being integrated into microreactor designs:
- Supercritical CO₂ (sCO₂) Brayton Cycles:
- sCO₂ turbines achieve significantly higher efficiency compared to traditional steam turbines.
- Their compact size and lower operational temperature requirements make them perfect for micro nuclear applications.
- sCO₂ cycles operate at efficiencies above 50%, compared to the ~33% efficiency of conventional Rankine steam cycles.
- Direct Heat-to-Electricity Conversion:
- Thermionic and thermoelectric generators can convert nuclear heat directly into electricity, eliminating the need for moving parts and mechanical losses.
- These systems provide ultra-quiet, maintenance-free power, ideal for space applications and military operations.
- Hybrid Brayton-Rankine Cycles:
- Some micro nuclear plants combine gas turbines (Brayton cycle) and steam turbines (Rankine cycle) to maximize power output across different temperature ranges.
- This hybrid approach allows microreactors to achieve greater energy utilization while providing flexible power output.
By implementing these cutting-edge energy conversion techniques, micro nuclear plants can generate more power from the same amount of fuel, reducing costs and improving sustainability.
AI-Driven Optimization for Continuous Efficiency Improvements
Artificial intelligence (AI) and digital automation are revolutionizing nuclear power operations by enhancing real-time monitoring, predictive maintenance, and energy optimization:
- Machine Learning for Reactor Performance:
- AI continuously analyzes reactor conditions, making real-time adjustments to maintain optimal efficiency and safety.
- Predictive analytics prevent unplanned downtime by detecting system irregularities before they become critical issues.
- Self-Regulating Reactor Control Systems:
- Some microreactors are designed with autonomous control mechanisms, reducing the need for human oversight.
- AI-enhanced self-regulating reactors can adapt their output to fluctuating energy demands, ensuring stable power supply in hybrid energy grids.
- Digital Twin Technology:
- A virtual replica of a micro nuclear plant is used to simulate operations, test efficiency improvements, and predict maintenance needs.
- Operators can fine-tune performance and reduce energy waste before making physical adjustments to the actual reactor.
These innovations allow micro nuclear plants to operate with maximum efficiency, minimal downtime, and enhanced safety, reducing operational costs and extending the life of critical reactor components.
Micro Nuclear Integration with Decentralized Energy Systems
Micro nuclear plants are increasingly being integrated into hybrid and decentralized energy networks, enhancing their role in resilient, low-carbon energy systems:
- Microgrids and Distributed Generation:
- Microreactors can operate independently or in conjunction with other energy sources to provide stable, localized power.
- They are particularly useful in remote communities, military bases, and island nations where traditional grid infrastructure is unavailable.
- Pairing Micro Nuclear with Renewables:
- Load Balancing: Micro nuclear plants can provide consistent power when solar and wind output fluctuates.
- Energy Storage Compatibility: Excess power can be stored in batteries or converted into hydrogen fuel for later use.
- Industrial Heat and Co-Generation:
- Many industrial processes require both electricity and high-temperature heat, which micro nuclear plants can supply efficiently.
- Sectors like steel production, hydrogen generation, and chemical refining benefit from using nuclear heat instead of fossil fuels.
By integrating micro nuclear plants with advanced grid technologies, they can function as key enablers of future smart energy systems that prioritize efficiency, sustainability, and resilience.
Economic and Environmental Benefits of Highly Efficient Micro Nuclear Plants
The efficiency gains in modern micro nuclear plants translate directly into economic and environmental advantages:
✅ Lower Operational Costs:
- Longer fuel cycles reduce refueling costs and reactor downtime.
- Autonomous operation minimizes the need for a large workforce.
✅ Significant Carbon Reduction:
- Replacing diesel generators in remote areas with micro nuclear plants eliminates CO₂ emissions.
- Industrial decarbonization efforts benefit from zero-emission heat and power.
✅ Rapid Deployment and Scalability:
- Modular microreactors can be deployed within months, compared to years for large-scale nuclear plants.
- Their factory-built, transportable design allows for rapid installation in diverse locations.
✅ Increased Energy Security:
- Micro nuclear plants reduce dependence on fossil fuel imports, ensuring stable energy supply even in geopolitically unstable regions.
- Military and critical infrastructure applications benefit from uninterruptible power in high-risk areas.
The Road Ahead: Pushing Efficiency Even Further
The future of micro nuclear efficiency will be shaped by continued advancements in reactor design, materials science, and energy storage integration:
- Ultra-High-Temperature Reactors (UHTRs): Developing reactors capable of operating at 1,000°C or higher for even more efficient power conversion.
- Closed-Loop Nuclear Fuel Recycling: Reusing spent fuel to extend reactor lifespan and reduce waste.
- Fusion-Powered Microreactors: Exploring small-scale nuclear fusion as a potential next-generation power source.
With these innovations, micro nuclear plants will continue to improve in efficiency, affordability, and sustainability, ensuring they play a central role in the global transition to clean, resilient, and decentralized energy systems.
Conclusion
Micro nuclear plants represent the future of high-efficiency, low-carbon power generation, providing flexible, scalable, and ultra-reliable energy solutions. By leveraging advanced reactor designs, cutting-edge energy conversion technologies, AI-driven optimization, and hybrid energy integration, these small reactors are redefining what is possible in nuclear power.
As the world moves toward a sustainable energy future, micro nuclear plants will play a critical role in decarbonization, energy security, and industrial transformation, ensuring efficient, clean, and resilient power for generations to come. 🚀⚛️
Micro reactors offer low-carbon electricity
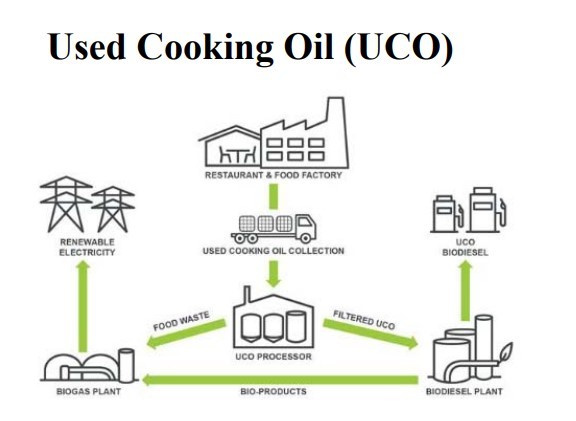
Micro reactors provide a low-carbon, reliable, and efficient source of electricity, making them an essential part of the transition to clean energy. Unlike fossil fuel-based power generation, micro nuclear reactors produce zero direct CO₂ emissions, offering a sustainable alternative for industries, remote communities, and decentralized energy systems. Their compact and modular design allows for rapid deployment, making them well-suited for applications where traditional grid infrastructure is unavailable or unreliable.
One of the primary advantages of micro reactors is their high energy density, meaning they generate a significant amount of electricity from a small amount of fuel. Advanced nuclear fuels, such as TRISO (Tri-structural Isotropic) fuel and High-Assay Low-Enriched Uranium (HALEU), enhance safety and efficiency, allowing micro reactors to operate for years or even decades without refueling. This longevity reduces operational costs and minimizes the logistical challenges associated with fuel supply chains.
Unlike intermittent renewable energy sources such as solar and wind, micro reactors provide continuous and dispatchable power. This makes them an ideal companion for hybrid energy systems, where they can serve as a baseload power source or step in to stabilize the grid when renewables fluctuate. Additionally, they can support energy storage technologies, such as hydrogen production or battery systems, enhancing overall energy system resilience.
Micro reactors also offer significant environmental benefits beyond their low-carbon footprint. Their small land footprint is advantageous compared to large-scale renewables that require vast areas for deployment. Moreover, many next-generation micro reactors are designed with passive safety features, reducing the risk of accidents and ensuring safe, autonomous operation.
Industries that require both electricity and high-temperature heat, such as hydrogen production, desalination, and heavy manufacturing, stand to benefit greatly from micro nuclear technology. Their ability to produce low-carbon process heat helps reduce dependence on fossil fuels, further cutting greenhouse gas emissions.
As governments and industries prioritize clean energy investments, micro reactors are emerging as a scalable and practical solution for achieving net-zero emissions. With ongoing advancements in reactor design, fuel recycling, and energy integration, they are poised to become a cornerstone of the future low-carbon energy landscape.
Micro reactors are increasingly recognized as a scalable, adaptable, and low-carbon energy solution capable of supporting a variety of applications, from remote power generation to industrial decarbonization. Their ability to operate independently from traditional energy grids makes them particularly valuable in regions with limited infrastructure, ensuring access to clean and stable electricity.
Decentralized and Resilient Energy Supply
A key advantage of micro reactors is their decentralized energy capability, which allows for localized electricity generation without reliance on large-scale power grids. This makes them an ideal solution for off-grid communities, military bases, island nations, and disaster-prone regions where energy security is critical. Unlike fossil fuel-based power plants that depend on continuous fuel supply chains, micro reactors can operate for years without refueling, reducing logistical challenges and enhancing energy independence.
Integration with Renewables for Carbon-Free Energy Systems
While renewables such as solar and wind are essential for decarbonization, they are intermittent and require backup solutions to maintain a stable energy supply. Micro reactors can function as complementary power sources, providing consistent, dispatchable energy when renewable output fluctuates due to weather conditions. This integration creates a resilient hybrid energy system that maximizes the use of renewables while ensuring continuous, reliable power.
Additionally, micro reactors can be used to store excess renewable energy in the form of hydrogen, batteries, or other energy carriers. By converting surplus electricity into green hydrogen, they help enable a zero-carbon fuel economy, supporting industries such as transportation, steel production, and chemical manufacturing.
High-Efficiency Power and Heat for Industrial Applications
Beyond electricity generation, micro reactors are uniquely suited for industrial applications requiring both power and high-temperature heat. Many sectors—such as cement production, refining, and desalination—depend on intense heat, which is typically produced by burning fossil fuels. By using nuclear heat instead, micro reactors help industries cut CO₂ emissions while improving energy efficiency.
- Hydrogen Production: Micro reactors can generate heat and electricity for electrolysis or thermochemical processes, making green hydrogen more cost-effective.
- Desalination: They provide constant power and thermal energy for large-scale water desalination, ensuring a stable supply of clean water in arid regions.
- Process Heat for Manufacturing: Industries requiring heat-intensive operations, such as steelmaking, ammonia production, and petrochemicals, can transition away from coal and gas by adopting nuclear heat.
Safety, Efficiency, and Sustainability Innovations
Modern micro reactors incorporate advanced safety features that make them inherently safer than traditional large reactors. Many designs rely on passive cooling systems, meaning they automatically shut down and dissipate heat without human intervention in case of an emergency. This self-regulating capability enhances safety and reduces the need for extensive operator oversight.
Furthermore, advancements in reactor fuel technology, such as TRISO fuel and molten salt fuels, enhance the efficiency and longevity of micro reactors. These fuel types have higher heat tolerance and lower proliferation risks, making them both safer and more sustainable.
As nuclear innovation progresses, fuel recycling technologies are also being developed to reuse spent fuel, further reducing nuclear waste. With closed-loop fuel cycles and next-generation reactor designs, micro reactors can significantly minimize environmental impact while maximizing efficiency.
The Future of Micro Nuclear Technology in a Low-Carbon World
Micro reactors represent a transformative step toward a low-carbon future, offering clean, efficient, and scalable energy solutions. Their ability to provide stable electricity, industrial heat, and hybrid energy integration makes them a cornerstone of decarbonization strategies worldwide. As more countries and industries commit to net-zero goals, the adoption of micro reactors will continue to expand, reinforcing their role in the global energy transition.
With ongoing advancements in reactor design, safety, and energy conversion technologies, micro reactors are poised to deliver sustainable, cost-effective, and highly reliable power for generations to come.
Micro Reactors: Enabling a Reliable and Low-Carbon Energy Future
Micro reactors are reshaping the energy landscape by offering continuous, low-carbon power with enhanced flexibility, efficiency, and scalability. Their small size, modular construction, and long fuel cycles make them an attractive alternative to fossil fuels, especially in applications where energy reliability is paramount. Whether for off-grid industries, remote communities, military operations, or hybrid energy systems, micro reactors provide a decentralized and resilient solution for the growing global demand for clean energy.
Advanced Fuel Technologies for Long-Term Operation
One of the key strengths of micro reactors is their ability to operate for years or even decades without refueling, significantly reducing maintenance and logistical challenges. This is made possible by advancements in high-assay low-enriched uranium (HALEU) fuel, TRISO fuel, and molten salt fuel technologies:
- HALEU Fuel:
- Increases fuel efficiency and reactor longevity.
- Enables smaller reactor cores with higher power output.
- Reduces the need for frequent refueling, making micro reactors highly autonomous.
- TRISO Fuel (Tri-structural Isotropic Particles):
- Encapsulates uranium in a multi-layered ceramic coating, making it extremely robust and meltdown-proof.
- Allows reactors to operate at higher temperatures, increasing efficiency.
- Offers superior radiation resistance and waste reduction.
- Molten Salt Fuel:
- Operates in a liquid state, enhancing thermal efficiency and allowing continuous fuel reprocessing.
- Provides inherent safety features, as the fuel solidifies in case of leaks or system failures.
- Eliminates the risk of high-pressure steam explosions, making the reactor inherently safer.
These fuel innovations enable micro reactors to function safely, efficiently, and with minimal environmental impact, making them a future-proof energy source for carbon-neutral power generation.
Micro Reactors and the Role of Hybrid Energy Systems
In an era where renewable energy is gaining momentum, micro reactors serve as a critical backbone for hybrid energy systems, ensuring a stable and uninterrupted power supply when solar and wind generation fluctuate. By integrating micro nuclear power with battery storage, green hydrogen production, and smart grid technologies, energy systems can achieve:
- Grid Stability: Micro reactors provide a steady baseload, balancing out the intermittency of wind and solar.
- Peak Shaving: When energy demand spikes, micro reactors can adjust output to meet peak consumption needs.
- Energy Storage Support: Excess power can be stored in batteries or converted into hydrogen fuel, ensuring energy availability even when nuclear output is reduced.
- Industrial Electrification: Industries that require constant, high-temperature heat and electricity, such as steel, cement, and chemical manufacturing, can use micro reactors to replace fossil fuels.
These hybrid systems not only enhance grid resilience but also help accelerate the transition to a fully decarbonized energy infrastructure.
Reducing Carbon Emissions in Critical Sectors
Micro reactors offer a game-changing solution for industries that are historically difficult to decarbonize, providing a clean, efficient replacement for coal, oil, and natural gas. Their impact extends across multiple sectors:
- Transportation & Shipping: Micro reactors can be used for port electrification and hydrogen fuel production for long-haul transport and maritime industries.
- Remote Mining Operations: Many mining sites rely on diesel generators, producing high carbon emissions. Micro reactors offer a zero-emission alternative, reducing costs and environmental impact.
- Agriculture & Food Production: Micro reactors provide stable energy for irrigation, processing, and refrigeration, reducing reliance on fossil-fuel-powered equipment.
By integrating micro nuclear energy into these sectors, carbon emissions can be significantly reduced while maintaining economic productivity and energy security.
Scalability and Rapid Deployment of Micro Reactors
Unlike traditional nuclear plants that require decades to plan, build, and operate, micro reactors are designed for rapid deployment with minimal infrastructure requirements. Their factory-built, modular design allows for:
- Scalability: Multiple micro reactors can be deployed together to create a customized power solution for different energy demands.
- Transportability: Some micro reactor designs can be shipped in standard containers, enabling quick installation in remote locations.
- Plug-and-Play Operation: Many micro reactors come pre-fueled and factory-sealed, requiring little on-site maintenance.
These features make micro reactors an ideal solution for emergency energy deployment, disaster relief, and remote industrial operations, ensuring power reliability under any circumstances.
Looking Ahead: The Future of Micro Nuclear Energy
As governments and industries seek long-term, low-carbon energy solutions, micro reactors are positioned to play a transformative role in the clean energy transition. Key areas of future development include:
- Advanced Reactor Materials: New materials that enhance heat tolerance, radiation resistance, and fuel longevity.
- Autonomous Operation & AI Integration: AI-driven controls that optimize performance, predict maintenance needs, and ensure safety.
- Closed-Loop Fuel Cycles: Technologies that recycle spent nuclear fuel, reducing waste and improving sustainability.
With continued research and investment, micro reactors will become even more efficient, safer, and cost-effective, making them a cornerstone of the global push for carbon-free energy. Their ability to deliver reliable, scalable, and clean power ensures they will remain a key player in industrial decarbonization, energy security, and next-generation energy systems.
Micro Reactors: A Sustainable and Scalable Energy Solution
Micro reactors are emerging as a disruptive innovation in clean energy, offering a highly efficient, flexible, and sustainable alternative to traditional power sources. Their ability to operate autonomously for years, generate low-carbon electricity and process heat, and integrate with renewables and energy storage systems makes them a key player in the global energy transition. As demand for resilient, decentralized, and emission-free energy grows, micro reactors are poised to transform the way electricity is generated and distributed.
Decentralized Power for a Resilient Energy Infrastructure
One of the greatest advantages of micro reactors is their ability to function as standalone power units, reducing dependency on large-scale, centralized power plants. In an era where grid security, energy independence, and climate resilience are becoming top priorities, micro reactors provide localized energy generation that is both reliable and carbon-free.
- Remote & Off-Grid Applications: Micro reactors can deliver continuous power to isolated communities, military bases, research stations, and disaster recovery zones, eliminating reliance on costly diesel generators.
- Urban and Industrial Microgrids: Cities and large industrial operations can integrate micro reactors into localized power grids, ensuring a stable energy supply without strain on national grids.
- Emergency Backup Power: In regions vulnerable to natural disasters, grid failures, or cyberattacks, micro reactors serve as an independent, uninterrupted power source for critical infrastructure.
This decentralized model reduces transmission losses, enhances energy security, and provides a stable foundation for future smart grids and distributed energy networks.
Enhancing Economic and Environmental Benefits
Micro reactors offer long-term cost advantages over conventional fossil fuel power systems. While the initial capital costs may be higher than those of gas or coal plants, the low operational expenses, long fuel cycles, and minimal maintenance requirements make them a highly cost-effective solution over time. Key economic benefits include:
- Reduced Fuel and Transportation Costs: Unlike fossil fuel plants that require constant fuel deliveries, micro reactors can operate for years without refueling, drastically cutting supply chain costs.
- Lower Carbon Taxes and Compliance Costs: As governments tighten carbon regulations, industries that adopt micro nuclear energy can avoid penalties, receive incentives, and maintain compliance with emission targets.
- Job Creation in Advanced Nuclear Technology: The deployment of micro reactors fosters high-skilled employment in engineering, manufacturing, and nuclear operations, supporting local and global economies.
Supporting Global Decarbonization Goals
Micro reactors align with international efforts to achieve net-zero emissions, serving as a stable, emission-free alternative to fossil fuels. Their role in key energy-intensive sectors will be crucial in reaching global climate targets:
- Industrial Heat & Energy: Manufacturing processes that rely on coal and gas can transition to zero-carbon nuclear heat, drastically reducing emissions in steel, aluminum, and chemical industries.
- Carbon-Free Hydrogen Production: Micro reactors enable the production of green hydrogen through high-temperature electrolysis, supporting clean transportation and energy storage solutions.
- Replacing Diesel in Remote Locations: Isolated regions and industries currently dependent on diesel generators can switch to micro nuclear power, reducing costs and environmental impact.
By complementing renewable energy sources and storage technologies, micro reactors provide continuous, low-carbon power, ensuring energy stability in a rapidly decarbonizing world.
Advancing Safety and Regulatory Adaptation
Modern micro reactors incorporate cutting-edge safety features that make them more secure and easier to regulate than traditional nuclear plants. These advances include:
- Passive Safety Mechanisms: Many designs use natural convection cooling, meltdown-resistant fuel, and passive heat dissipation, eliminating the risk of reactor overheating.
- Autonomous Operation: AI-powered systems can monitor and adjust reactor performance in real-time, ensuring safe and efficient operation with minimal human intervention.
- Smaller Environmental Footprint: Unlike large nuclear plants, micro reactors require minimal land, water, and infrastructure, making them ideal for urban integration and small-scale deployment.
Regulatory frameworks are evolving to accommodate and accelerate the deployment of micro reactors. Governments and energy agencies worldwide are streamlining licensing processes, providing incentives for small-scale nuclear development, and encouraging public-private partnerships to drive innovation.
The Road Ahead: Widespread Adoption and Innovation
As the global energy landscape continues to evolve, micro reactors are positioned to play a critical role in the future of clean power generation. Ongoing research and investment will further enhance their capabilities, with key developments in:
- Advanced Fuel Recycling: Reducing nuclear waste and improving fuel sustainability through closed-loop fuel cycles.
- Next-Generation Modular Designs: Making reactors smaller, more affordable, and faster to deploy in various environments.
- Integration with Smart Energy Grids: Enhancing grid stability and efficiency through AI-driven energy management and predictive maintenance.
With growing support from policymakers, energy developers, and industries, micro reactors will become a foundational component of low-carbon, resilient, and decentralized energy systems. Their ability to deliver stable, emission-free power at any scale ensures they will remain a driving force in the clean energy revolution for decades to come.
Portable Nuclear Plants: Transforming Energy Access for Remote Sites
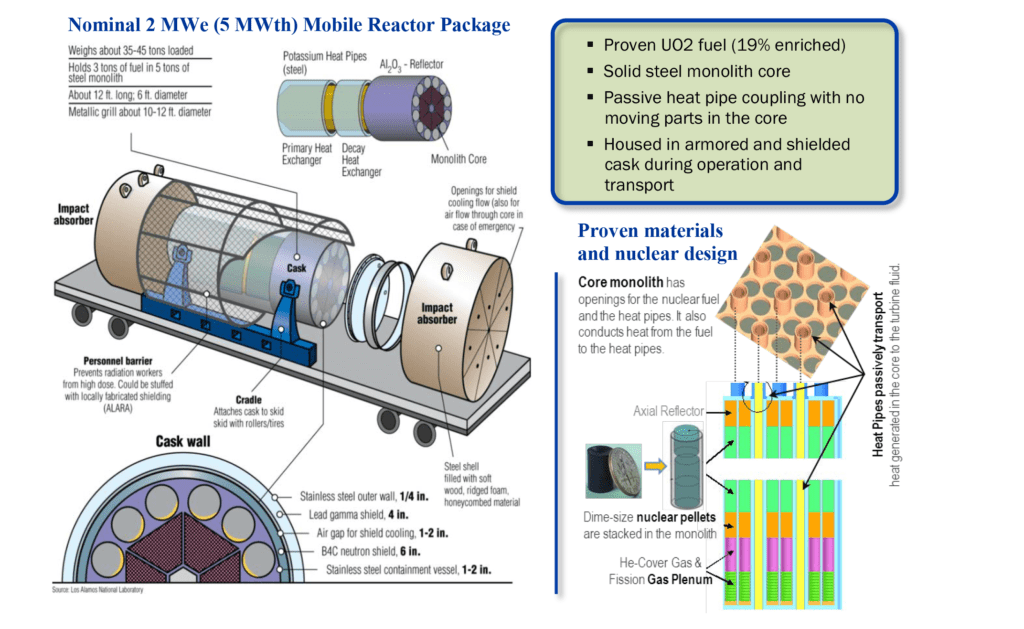
Portable nuclear plants are a game-changing innovation for delivering reliable, low-carbon energy to remote locations, off-grid industries, military bases, and disaster-stricken areas. Unlike traditional power sources that require extensive infrastructure, fuel supply chains, or intermittent renewable generation, portable nuclear plants provide continuous, self-sustaining energy in areas where conventional power solutions are impractical.
Advantages of Portable Nuclear Plants
1. Reliable, Long-Term Power Supply
Portable nuclear reactors can operate for years without refueling, making them ideal for locations with limited fuel access or extreme environmental conditions. Unlike diesel generators, which require constant fuel deliveries, portable nuclear plants eliminate the logistical and financial burden of fuel transportation.
2. Modular and Rapid Deployment
These reactors are designed for easy transport and installation, often fitting into standard shipping containers or deployable units. Their modular nature allows them to be delivered by truck, rail, or ship, providing instant energy solutions in remote industrial sites, mining operations, Arctic communities, or forward military bases.
3. Carbon-Free and Environmentally Friendly
Unlike fossil-fuel-based power generation, portable nuclear plants produce no greenhouse gas emissions, supporting global decarbonization efforts. They provide a sustainable alternative to diesel or coal power, which is commonly used in remote locations but is expensive and highly polluting.
4. Resilient and Autonomous Operation
Modern portable nuclear plants are designed to operate with minimal human intervention. Many feature autonomous control systems and passive safety mechanisms, ensuring they function safely even in the harshest environments. Their resilience to extreme weather conditions and energy demand fluctuations makes them ideal for isolated regions.
5. Powering Industrial and Humanitarian Operations
Portable reactors can provide power for a variety of applications, including:
- Mining and Resource Extraction – Continuous, high-energy output for equipment, processing facilities, and worker accommodations.
- Disaster Relief and Emergency Response – Rapid deployment of clean energy for recovery operations after natural disasters.
- Military and Defense – Remote forward bases require secure and independent power sources for radar, communication, and logistics.
- Scientific Research Stations – Arctic, Antarctic, and deep-space research facilities benefit from long-term, reliable energy.
Future of Portable Nuclear Technology
With advancements in reactor miniaturization, passive safety designs, and advanced nuclear fuels, portable nuclear plants are becoming an increasingly viable solution for global energy security. Governments and private industries are investing in next-generation microreactors that are:
- Smaller, safer, and more efficient
- Faster to deploy with factory-built modular components
- Designed for recycling spent fuel and reducing waste
As the world seeks resilient, clean, and decentralized energy solutions, portable nuclear plants are poised to revolutionize power generation in remote and underserved regions, ensuring stable, emission-free electricity anywhere on the planet.
Expanding the Role of Portable Nuclear Plants in Remote Energy Systems
Portable nuclear plants are revolutionizing remote energy access, providing continuous, low-carbon power where traditional infrastructure is absent or unreliable. Their ability to function autonomously, withstand extreme conditions, and eliminate the need for frequent fuel deliveries makes them an essential solution for industries, governments, and humanitarian efforts. As global energy demands rise and the need for carbon-free alternatives grows, portable nuclear technology is set to play an increasingly significant role in sustainable development, security, and industrial operations.
Technological Innovations Driving Portable Nuclear Power
Recent advancements in nuclear reactor miniaturization, modular construction, and advanced fuel cycles have made portable nuclear plants more efficient, transportable, and scalable. Key innovations include:
1. High-Assay Low-Enriched Uranium (HALEU) Fuel
Many portable reactors are designed to use HALEU fuel, which allows for:
- Longer operational lifetimes without refueling (ranging from several years to decades).
- Higher efficiency and reduced waste production compared to conventional nuclear fuels.
- Enhanced safety features, as HALEU fuels are designed for inherent stability under various conditions.
2. Passive Safety Systems and Autonomous Operation
Portable reactors integrate advanced passive safety mechanisms, reducing the need for constant human monitoring. Features include:
- Self-regulating core designs, which automatically adjust power output based on demand.
- Passive cooling systems, eliminating the need for active coolant circulation.
- Automated shutdown protocols, ensuring safety in case of external disruptions.
3. Compact, Modular, and Transportable Designs
Unlike large-scale nuclear plants, which require complex infrastructure and long construction periods, portable nuclear plants are designed for quick deployment and relocation. Some models are built to fit within standardized shipping containers, enabling transportation via land, sea, or air. These compact reactors can be set up and operational within days or weeks, compared to the years required for traditional nuclear facilities.
Strategic Applications of Portable Nuclear Power
The flexibility of portable nuclear reactors allows them to be deployed in a variety of settings, each with unique energy needs:
1. Remote Industrial and Mining Operations
Mining operations in Arctic regions, deserts, and deep jungles often rely on expensive diesel generators. Portable nuclear plants provide a more stable and cost-effective power source, enabling:
- Continuous electricity for heavy machinery, processing plants, and worker accommodations.
- Reduced fuel transport costs and emissions, cutting reliance on fossil fuels.
- Sustained power for critical infrastructure in isolated locations.
2. Military and Defense Installations
Military bases in hostile or remote regions require secure, independent power sources for:
- Command and control systems, radar, and communications.
- Logistics hubs and field hospitals in conflict zones.
- Electrification of defense outposts without vulnerable supply chains.
By using portable nuclear plants, energy security is enhanced, reducing dependence on fuel convoys, which are often targeted in conflict zones.
3. Humanitarian and Disaster Relief Efforts
Following natural disasters, hurricanes, or earthquakes, local power grids can remain inoperable for weeks or months. Portable nuclear plants can be rapidly deployed to provide emergency electricity for:
- Medical facilities, water purification, and refugee camps.
- Restoring essential infrastructure in disaster-stricken regions.
- Powering telecommunications and emergency response units.
4. Scientific and Space Exploration Missions
Research stations in Antarctica, deep-sea facilities, or space habitats require long-term, uninterrupted power supplies in harsh environments. Portable reactors ensure:
- Sustained operations for climate research and deep-space exploration.
- Self-sufficient energy solutions in extreme conditions.
- Reduced dependence on fossil fuel-based generators, enhancing sustainability.
Environmental and Economic Benefits of Portable Nuclear Plants
1. Zero Carbon Emissions and Sustainability
Unlike diesel generators, which release large amounts of CO₂ and air pollutants, portable nuclear plants generate zero direct emissions, helping to:
- Combat climate change by reducing reliance on fossil fuels.
- Support global decarbonization goals in industries and isolated regions.
- Improve air quality in remote communities that currently depend on carbon-heavy power sources.
2. Cost Savings and Economic Viability
Although initial investment costs for portable nuclear reactors are higher than those of diesel or gas power systems, the long-term savings are significant due to:
- Lower fuel costs, as reactors operate for years without refueling.
- Reduced logistics expenses, eliminating the need for constant fuel transportation.
- Minimal maintenance requirements, decreasing operational costs over time.
Future Outlook: The Expansion of Portable Nuclear Technology
As governments, industries, and defense agencies recognize the strategic and environmental benefits of portable nuclear energy, investment in this technology is rapidly expanding. The future of portable nuclear plants will likely see:
- Advanced reactor designs with even greater efficiency and transportability.
- Increased regulatory support for streamlined deployment and operation.
- Integration with renewable energy systems, allowing hybrid energy solutions for off-grid applications.
- Global adoption in underserved regions, providing electricity access to millions.
By offering clean, autonomous, and scalable energy, portable nuclear plants are set to reshape the global energy landscape, ensuring that even the most remote locations have access to stable, low-carbon power.
Scaling Portable Nuclear Plants for Global Energy Needs
As the demand for resilient, clean, and scalable energy solutions grows, portable nuclear plants are emerging as a key technology for addressing energy access challenges in remote, industrial, and emergency settings. Their ability to deliver stable, long-term power without relying on complex infrastructure or fuel supply chains positions them as a transformative innovation in the global energy sector. With ongoing advancements in reactor design, modular manufacturing, and regulatory adaptation, portable nuclear plants are poised to become a mainstream solution for off-grid energy generation.
Advanced Reactor Designs Enhancing Portability and Efficiency
The next generation of portable nuclear plants is being designed to maximize efficiency, safety, and scalability, incorporating cutting-edge advancements such as:
1. Micro Modular Reactors (MMRs) with Extended Lifespans
MMRs are compact, factory-built reactors that can be rapidly deployed and operated for decades without refueling. Key features include:
- Fuel cycles lasting 10-20 years, eliminating the need for frequent refueling logistics.
- Advanced cooling systems, using liquid metal, molten salt, or high-temperature gas for enhanced thermal efficiency.
- Scalability, allowing multiple reactors to be combined for increased power output.
2. Transportable Reactor Cores for Rapid Relocation
Some designs focus on reactor cores that can be easily removed and replaced, enabling:
- Faster redeployment to different locations based on changing energy needs.
- Minimal downtime, as a new core can be installed while the used core is transported for refueling.
- Reduced decommissioning costs, making portable nuclear plants more economically viable.
3. Hybrid Systems Integrating Renewables and Storage
Portable nuclear plants can be combined with solar, wind, and battery storage to create hybrid microgrids, ensuring:
- Stable energy supply by balancing nuclear baseload power with intermittent renewables.
- Grid resilience, allowing systems to function autonomously in off-grid environments.
- Lower overall costs, optimizing the energy mix for different operational scenarios.
Expanding Use Cases for Portable Nuclear Power
The adaptability of portable nuclear plants allows them to serve an expanding range of applications, addressing both economic and humanitarian energy challenges.
1. Powering Extreme Environments and Space Missions
- Lunar and Martian Colonization – NASA and other space agencies are exploring portable reactors for off-world bases, ensuring continuous power for life support, scientific research, and habitat construction.
- Deep-Sea Exploration – Underwater research stations and autonomous submersibles can benefit from long-lasting, high-density energy sources.
- Antarctic and Arctic Facilities – Research outposts in polar regions, where solar power is unreliable, can achieve full energy independence with microreactors.
2. Supporting Remote Economic Development
Portable nuclear plants can empower economic growth in underserved regions, providing:
- Electricity for rural communities, reducing reliance on expensive diesel generators.
- Power for infrastructure projects, enabling roads, water treatment, and communication networks.
- Energy access for emerging markets, supporting industrialization in Africa, Southeast Asia, and South America.
3. Enhancing National Security and Energy Sovereignty
- Decentralized Energy Resilience – Countries can use portable nuclear plants to ensure energy independence in strategic locations.
- Military Base Sustainability – Forward operating bases can function without vulnerable fuel supply lines, reducing risk and operational costs.
- Cybersecurity and Grid Stability – Small, modular nuclear grids are less susceptible to cyberattacks and large-scale grid failures.
Addressing Regulatory and Public Perception Challenges
Despite their benefits, portable nuclear plants face regulatory, safety, and public acceptance challenges that must be addressed for widespread deployment. Efforts to overcome these barriers include:
- Simplified Licensing Frameworks – Governments are working to streamline approval processes for microreactors to speed up deployment.
- Public Awareness Campaigns – Educating communities about the safety, reliability, and environmental benefits of nuclear energy.
- International Collaboration – Countries are forming partnerships to standardize safety protocols and export reactor designs globally.
Conclusion: The Future of Portable Nuclear Power
As technology continues to advance, portable nuclear plants will become a mainstay in global energy infrastructure, delivering carbon-free, reliable power to regions where traditional solutions are impractical. Whether powering remote industries, military operations, disaster recovery efforts, or even future space colonies, portable reactors represent a clean, efficient, and scalable energy revolution that will shape the future of sustainable development and energy security worldwide.
Global Adoption and the Future Impact of Portable Nuclear Plants
With growing energy demands and increasing pressure to reduce carbon emissions, portable nuclear plants are set to become a fundamental component of global energy infrastructure. Their ability to provide stable, long-term, and carbon-free power in remote, industrial, and emergency settings makes them a highly valuable innovation. As governments, industries, and research institutions continue to invest in advanced nuclear technologies, the future of portable nuclear power looks promising, with expanded applications, enhanced safety features, and greater public acceptance.
Scaling Up Deployment: Overcoming Challenges and Driving Innovation
The transition from experimental and pilot-stage portable reactors to mainstream deployment requires overcoming technical, regulatory, and societal challenges. Ongoing developments in the nuclear industry are addressing these challenges by focusing on:
1. Advancements in Reactor Design for Greater Portability and Efficiency
Future portable reactors are expected to feature:
- Smaller, lighter reactor cores that can be transported with minimal logistical complexity.
- Rapid deployment capabilities, allowing nuclear energy to be set up in days rather than months or years.
- Enhanced modularity, enabling seamless integration with existing energy systems, including microgrids and hybrid renewable setups.
2. Strengthening International Regulatory Frameworks
Regulatory challenges have traditionally slowed down nuclear deployment. To accelerate portable reactor adoption, governments and international organizations are:
- Standardizing nuclear safety protocols across different regions.
- Developing fast-track approval processes for microreactors to streamline licensing and deployment.
- Ensuring rigorous safety testing while maintaining efficiency in the regulatory process.
3. Public Awareness and Addressing Nuclear Perception
Despite the proven safety of modern nuclear technology, public perception remains a challenge. To promote widespread acceptance, nuclear stakeholders are:
- Launching educational campaigns to inform the public about the safety, reliability, and environmental benefits of portable reactors.
- Demonstrating successful case studies of portable nuclear plants providing clean, uninterrupted power.
- Highlighting the role of nuclear energy in global decarbonization and energy security.
Strategic Applications and the Expanding Role of Portable Nuclear Energy
As portable nuclear plants become more accessible and scalable, their applications will expand beyond traditional off-grid solutions.
1. Large-Scale Industrial Applications
Industries that require constant, high-output energy will benefit from portable nuclear plants, especially in:
- Hydrogen production – Nuclear reactors can provide the high-temperature heat needed for efficient hydrogen fuel generation.
- Steel and cement manufacturing – Industries that are traditionally carbon-intensive can shift to clean nuclear power to reduce emissions.
- Desalination plants – Portable reactors can power large-scale water purification systems, providing fresh water in arid regions.
2. Global Energy Support and Disaster Resilience
- Developing Nations – Portable nuclear plants can bring reliable electricity to off-grid villages, improving economic development and quality of life.
- Disaster Recovery Operations – In the event of a hurricane, earthquake, or other natural disaster, a deployable nuclear plant could restore electricity faster than rebuilding traditional grid infrastructure.
- Refugee Camps and Emergency Shelters – Secure and sustainable power sources are critical for humanitarian efforts, enabling access to clean water, medical facilities, and communication networks.
3. Space and Off-Planet Colonization
- Lunar and Martian Bases – Space agencies are actively researching nuclear power for sustained human presence on the Moon and Mars, ensuring continuous life support, research, and resource processing.
- Deep Space Probes – Future space exploration missions may rely on compact nuclear reactors to power spacecraft and exploration rovers for extended periods.
The Road Ahead: A New Era for Portable Nuclear Technology
As advancements continue, portable nuclear plants will transition from a niche innovation to a widely adopted energy solution. The combination of technological improvements, regulatory adaptation, and global acceptance will drive:
- More widespread deployment in industrial, military, and humanitarian sectors.
- Greater integration with existing energy infrastructure, ensuring a seamless shift to nuclear-powered microgrids.
- Continued investment in reactor safety, efficiency, and modularization, making nuclear energy more accessible than ever.
By providing carbon-free, reliable, and scalable energy, portable nuclear plants will play a crucial role in reshaping the global energy landscape, ensuring that even the most remote and challenging locations have access to stable, sustainable power.
Steam Turbines: Converting Micro Nuclear Heat into Power
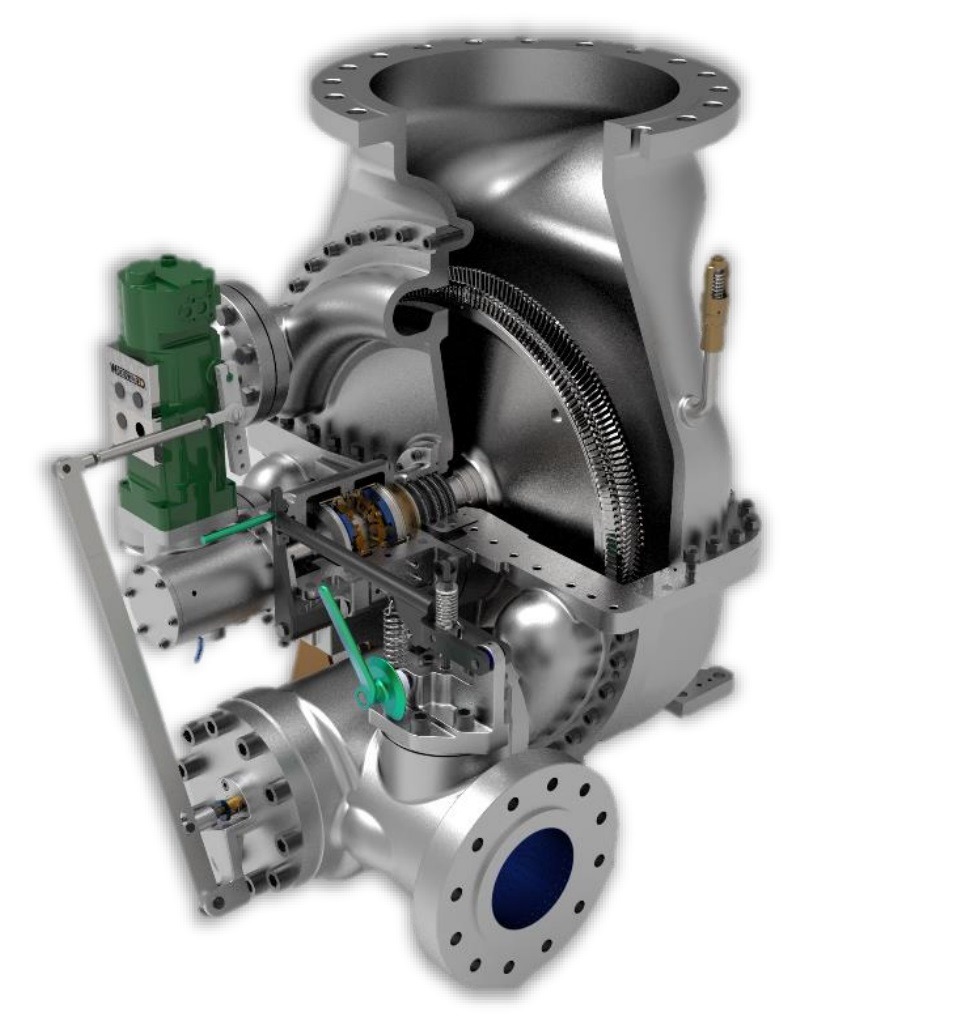
Micro nuclear reactors generate high-temperature heat, which is efficiently converted into electricity using steam turbines. These turbines are an essential component in most nuclear power systems, including small modular reactors (SMRs) and microreactors, as they enable the transformation of thermal energy into mechanical and electrical power.
How Steam Turbines Work in Micro Nuclear Systems
- Heat Generation from the Micro Nuclear Reactor
- A microreactor’s core produces heat through nuclear fission, where atoms split and release large amounts of energy.
- This heat is transferred to a working fluid, typically water, in a primary loop.
- Some designs use helium, molten salt, or liquid metal as the heat transfer medium to reach higher temperatures.
- Steam Production in a Heat Exchanger
- The primary loop transfers heat to a secondary loop containing water.
- The water in this loop turns into high-pressure steam, which is then directed toward the steam turbine.
- Mechanical Power Generation via the Steam Turbine
- The high-pressure steam flows into the turbine’s blades, causing them to rotate.
- The rotating motion is transferred to a connected generator, producing electricity.
- As steam expands and loses pressure, it moves through multiple turbine stages for maximum energy extraction.
- Condensation and Recycling of Steam
- After passing through the turbine, the low-pressure steam is condensed back into water using a cooling system.
- The condensed water is pumped back into the system to be reheated, completing the cycle.
- This closed-loop system minimizes water loss and enhances efficiency.
Advantages of Steam Turbines in Micro Nuclear Applications
- Efficient Power Conversion – Steam turbines provide high thermal efficiency, converting more heat into electricity compared to other methods.
- Scalability – Modern steam turbines are designed to work with compact, modular nuclear units, making them ideal for off-grid, industrial, and emergency power applications.
- Reliable and Continuous Operation – Unlike solar or wind energy, steam turbines in micro nuclear plants provide stable, 24/7 power output.
- Lower Emissions and Sustainability – Steam-based nuclear power is a zero-carbon alternative to fossil fuel-based steam plants.
Future of Steam Turbines in Micro Nuclear Energy
With advances in high-temperature reactors, supercritical steam cycles, and integrated turbine designs, micro nuclear plants are expected to achieve even greater efficiency and flexibility. By integrating new materials and cooling technologies, future steam turbines will optimize energy output, reduce maintenance needs, and expand nuclear power accessibility worldwide.
Steam turbines play a crucial role in converting heat from micro nuclear reactors into usable electricity. These turbines operate by harnessing the high-temperature thermal energy produced during nuclear fission and transforming it into mechanical motion, which in turn drives an electric generator. In micro nuclear reactors, the compact core generates intense heat, which is transferred to a working fluid, typically water, in a controlled loop. This heat exchange process causes the water to turn into high-pressure steam, which is then directed toward the steam turbine. As the steam flows through the turbine, it strikes a series of blades, causing them to rotate and drive a generator, ultimately producing electricity. The steam then moves through multiple turbine stages, expanding and losing pressure while extracting maximum energy.
After passing through the turbine, the low-pressure steam is cooled and condensed back into water using a heat exchanger or cooling system, allowing it to be recycled in a closed-loop process. This method ensures efficient energy use while minimizing water loss. Steam turbines in micro nuclear applications offer numerous advantages, including high thermal efficiency, stable and continuous operation, and a zero-carbon energy footprint. Unlike solar or wind power, nuclear-generated steam turbines provide a consistent power output, making them ideal for off-grid, industrial, and remote energy needs.
Advances in high-temperature reactor designs, supercritical steam cycles, and integrated turbine systems continue to enhance the efficiency of these power generation units, paving the way for scalable, resilient, and sustainable micro nuclear energy solutions that can be deployed worldwide. As technology progresses, steam turbines in micro nuclear systems will become even more optimized, allowing for greater flexibility, longer operational lifespans, and reduced maintenance requirements, reinforcing their role in the future of low-carbon, decentralized power generation.
The integration of steam turbines with micro nuclear reactors is transforming the way decentralized and off-grid power is generated, offering a reliable, efficient, and low-carbon alternative to conventional energy sources. As microreactors produce heat through nuclear fission, this thermal energy is transferred to a working fluid, typically water or an advanced heat transfer medium such as helium or molten salt, depending on the reactor design. The heated fluid then generates high-pressure steam, which drives the steam turbine, converting thermal energy into mechanical rotation. This rotational energy powers an electric generator, producing a steady supply of electricity. The efficiency of steam turbines in micro nuclear applications is enhanced by multi-stage expansion processes, where steam undergoes successive pressure drops while maximizing energy extraction.
After passing through the turbine, the steam is condensed back into liquid form using cooling systems, enabling it to be recirculated in a closed-loop cycle, improving efficiency and minimizing resource consumption. The closed-loop design also ensures that micro nuclear plants operate with minimal water loss, making them highly suitable for remote and arid locations where water availability is limited. These systems provide a continuous power supply, unaffected by weather conditions or fuel supply disruptions, making them an attractive solution for isolated communities, military bases, and industrial operations requiring stable and long-term energy. Unlike fossil-fuel-powered steam turbines, those driven by micro nuclear reactors generate electricity without greenhouse gas emissions, significantly reducing the environmental impact of energy production.
Moreover, advancements in microreactor design, such as high-temperature gas-cooled reactors and liquid-metal-cooled reactors, further improve the efficiency and durability of steam turbine systems by enabling higher operating temperatures and improved thermal conversion rates. As the demand for decentralized and low-carbon energy solutions grows, the role of steam turbines in micro nuclear applications will continue to expand, driving innovation in turbine materials, cooling technologies, and compact generator systems to optimize performance and sustainability. The adaptability of these systems makes them a key component in the transition to resilient, emission-free power sources that can be deployed in diverse settings, from remote industrial sites to disaster-stricken regions in need of rapid and reliable energy access.
Steam turbines in micro nuclear systems are poised to become a cornerstone of decentralized energy production, offering an efficient and sustainable means of converting nuclear heat into electricity. These compact power systems rely on nuclear fission to generate high-temperature heat, which is then transferred to a working fluid such as water, helium, or molten salt, depending on the reactor’s design. In conventional designs using water as the working fluid, the heat exchanger produces high-pressure steam that is directed through a steam turbine, causing its blades to spin and generate mechanical energy. This rotational force is then converted into electricity by a connected generator, providing a continuous and stable power output.
The efficiency of this process is maximized through multi-stage expansion, where steam undergoes successive pressure drops to extract the highest possible amount of energy before being cooled and condensed back into liquid form for reuse in a closed-loop cycle. This closed system minimizes waste and ensures consistent operation, making micro nuclear plants particularly advantageous for remote and off-grid locations where traditional power infrastructure is impractical. The ability to provide steady, emission-free electricity without reliance on fossil fuels makes micro nuclear steam turbines an attractive alternative to diesel generators, especially for critical applications such as military operations, industrial facilities, space missions, and disaster relief efforts.
Recent advancements in reactor technology, including high-temperature gas-cooled reactors and molten salt reactors, have further improved the performance of these turbines by enabling higher operating temperatures, increasing thermal efficiency, and reducing maintenance needs. Additionally, innovations in steam turbine materials and cooling methods are helping to extend operational lifespans and improve energy conversion rates, making these systems more viable for widespread deployment. As the global demand for reliable, low-carbon energy grows, micro nuclear reactors coupled with steam turbines will play an increasingly vital role in meeting energy needs while reducing environmental impact. Their ability to integrate seamlessly with microgrids and hybrid renewable energy systems further enhances their flexibility, ensuring that they can provide resilient and adaptable power solutions in a variety of challenging environments. With ongoing research and development, the future of steam turbines in micro nuclear applications looks promising, paving the way for a new era of efficient, decentralized, and sustainable power generation.
As micro nuclear technology advances, steam turbines continue to play a critical role in harnessing nuclear heat for efficient and sustainable power generation. These turbines operate by utilizing the immense thermal energy produced within microreactors, where controlled nuclear fission releases heat that is transferred to a working fluid. In most designs, this fluid is water, which, upon heating, transforms into high-pressure steam that drives the turbine blades. The mechanical energy produced from this rotational motion is then converted into electricity via a generator, providing a stable and continuous power source.
One of the key advantages of steam turbines in micro nuclear applications is their ability to achieve high thermal efficiency through multi-stage expansion, where steam is gradually decompressed to extract maximum energy before being condensed and recycled in a closed-loop system. This not only enhances the overall efficiency of the power cycle but also reduces water consumption, making micro nuclear plants highly viable for deployment in remote or arid environments where water resources are scarce.
The reliability and scalability of steam turbines in micro nuclear systems offer significant advantages over traditional energy sources. Unlike fossil fuel-powered plants, which require a constant supply of fuel and produce greenhouse gas emissions, nuclear steam turbines operate with minimal fuel input and generate zero carbon emissions, aligning with global efforts to transition to clean energy solutions. Additionally, their compact design allows them to be transported and deployed in locations where grid infrastructure is weak or nonexistent, providing a resilient and independent power supply. This makes them particularly valuable for critical applications such as remote industrial sites, isolated communities, military installations, and disaster relief operations, where consistent and reliable electricity is essential.
Technological advancements in reactor design, turbine materials, and cooling methods are continuously improving the efficiency and longevity of these systems. High-temperature gas-cooled reactors, molten salt reactors, and liquid-metal-cooled reactors are enabling higher steam temperatures and pressures, leading to greater power output and improved turbine performance. At the same time, developments in supercritical steam cycles and advanced heat exchanger designs are pushing the boundaries of thermal efficiency, further optimizing the energy conversion process. The integration of micro nuclear plants with microgrids and hybrid renewable energy systems is also expanding their role in decentralized power generation, allowing for greater flexibility in energy distribution and storage.
As the demand for clean and resilient energy solutions continues to grow, steam turbines in micro nuclear applications are set to play an increasingly prominent role in the global energy transition. Their ability to provide stable, low-carbon electricity in a variety of settings makes them an attractive option for industries and governments looking to secure reliable power while minimizing environmental impact. Ongoing research and development will further refine these systems, ensuring they remain at the forefront of sustainable and efficient energy production for years to come.
Micro nuclear plants enable off-grid power

Micro nuclear plants are transforming the landscape of off-grid power by providing a stable, long-term energy source that operates independently of traditional electrical grids. These compact reactors generate heat through nuclear fission, which is then converted into electricity using efficient power cycles, such as steam turbines or direct heat-to-electricity conversion methods. Unlike diesel generators or renewable energy sources like solar and wind, micro nuclear plants offer continuous power output, unaffected by fuel supply disruptions or weather conditions. This makes them an ideal solution for remote locations, industrial operations, military installations, and disaster relief efforts where reliable energy is critical. Their small size and modular design allow for rapid deployment and scalability, ensuring that power can be delivered where and when it is needed.
One of the key advantages of micro nuclear plants is their fuel efficiency and longevity. A single fuel load can last for several years, significantly reducing the need for refueling logistics in isolated areas. This is particularly beneficial for Arctic research stations, deep-sea mining operations, and space exploration missions, where traditional fuel delivery is costly and logistically complex. Additionally, these reactors produce zero direct carbon emissions, making them an attractive alternative to fossil fuel-based power generation in remote areas where environmental concerns are paramount.
Technological advancements in microreactor design have further enhanced their viability for off-grid applications. High-temperature gas-cooled reactors, liquid-metal-cooled reactors, and molten salt reactors offer improved thermal efficiency and safety, while passive cooling systems eliminate the need for extensive water supplies, making them more adaptable to arid or water-scarce environments. Some designs also incorporate heat storage and hybrid integration, allowing them to work alongside renewable sources like solar and wind, balancing energy output based on demand.
As global energy needs continue to evolve, micro nuclear plants will play an essential role in decentralized power generation, enabling industries, governments, and communities to achieve energy independence. Their ability to provide scalable, resilient, and low-carbon electricity makes them a cornerstone of future off-grid energy solutions, ensuring reliable power in even the most challenging environments.
Micro nuclear plants are redefining off-grid power by offering a reliable, long-term energy solution that operates independently of centralized power networks. These compact reactors use nuclear fission to generate heat, which is then converted into electricity through advanced power cycles such as steam turbines or direct heat-to-electricity technologies. Unlike conventional off-grid power sources such as diesel generators, which require constant fuel deliveries, or solar and wind power, which depend on weather conditions, micro nuclear plants provide a steady and uninterrupted supply of electricity. This makes them particularly valuable for remote industrial sites, isolated communities, military operations, and emergency response efforts where stable energy is essential. Their small footprint and modular design allow for rapid deployment and scalability, ensuring that power can be tailored to specific needs without requiring extensive infrastructure.
One of the major benefits of micro nuclear plants is their ability to operate for years without refueling, significantly reducing logistical challenges in remote locations. Unlike conventional power sources that require frequent fuel deliveries, a single microreactor fuel load can last for up to a decade, making them highly efficient for Arctic bases, mining operations, space missions, and offshore facilities. Additionally, micro nuclear plants produce electricity with zero direct carbon emissions, providing an environmentally friendly alternative to fossil fuel-based power generation in areas where sustainability is a priority. The development of advanced reactor technologies, including high-temperature gas-cooled reactors, molten salt reactors, and liquid-metal-cooled reactors, has further increased their efficiency, safety, and adaptability. Many modern designs feature passive cooling systems that do not require large water supplies, allowing for deployment in arid regions and other challenging environments.
The integration of micro nuclear plants with microgrids and hybrid renewable energy systems expands their potential for decentralized energy production. These reactors can operate independently or in conjunction with solar, wind, and battery storage systems to optimize energy availability and efficiency. Their ability to deliver continuous power enhances grid resilience and energy security, particularly in disaster-prone or geopolitically unstable regions. As advancements in reactor miniaturization, materials, and safety mechanisms continue, micro nuclear plants will become an increasingly viable solution for off-grid power generation. Their capacity to provide scalable, long-term, and low-carbon electricity will make them a key component in global efforts to expand reliable energy access, reduce dependency on fossil fuels, and support sustainable development in even the most remote and extreme environments.
Micro nuclear plants are emerging as a game-changing solution for off-grid power generation, offering a continuous and independent electricity supply to remote and energy-insecure locations. Unlike traditional energy sources that depend on frequent fuel shipments or favorable weather conditions, these small-scale reactors harness nuclear fission to produce heat, which is then converted into electricity through highly efficient power cycles. This ability to generate consistent power for extended periods without refueling makes micro nuclear plants ideal for locations where energy reliability is critical, such as Arctic research stations, mining operations, military installations, space missions, and disaster relief zones. Their compact, modular design allows for rapid deployment and scalability, ensuring that power can be supplied where it’s needed without the need for an extensive transmission infrastructure.
One of the defining advantages of micro nuclear plants is their long operational lifespan and minimal maintenance requirements. Many designs are engineered to run for several years—sometimes a decade or more—without the need for refueling, drastically reducing logistical challenges in remote regions. This is a stark contrast to diesel generators, which require constant fuel resupply and are subject to supply chain vulnerabilities. Additionally, micro nuclear plants produce zero direct carbon emissions, making them a sustainable alternative to fossil fuel-based power generation, particularly in areas focused on reducing environmental impact and meeting decarbonization goals. Advanced reactor designs, including high-temperature gas-cooled reactors, molten salt reactors, and liquid-metal-cooled reactors, further enhance efficiency and safety by enabling higher thermal outputs and incorporating passive cooling systems that eliminate the need for large water sources.
Beyond standalone deployment, micro nuclear plants can be integrated into microgrids, allowing them to work in conjunction with renewable energy sources such as solar and wind to create a stable, hybrid energy network. This synergy enhances energy security, reduces storage needs, and allows for better management of electricity demand. In regions prone to natural disasters or geopolitical instability, micro nuclear plants provide a resilient, self-sustaining power source, reducing dependence on fragile grid infrastructure and mitigating the risks associated with supply chain disruptions. As advancements in reactor miniaturization, digital monitoring, and passive safety features continue, micro nuclear plants will play an increasingly significant role in global energy transition efforts, offering a scalable, carbon-free, and long-lasting power solution for diverse applications across the world.
Micro nuclear plants are revolutionizing off-grid power by offering a resilient, long-lasting, and low-maintenance energy solution for remote locations, industrial operations, and emergency response scenarios. Unlike conventional power sources, which rely on continuous fuel deliveries or favorable environmental conditions, micro nuclear plants provide a stable and self-sustaining electricity supply, making them invaluable for critical infrastructure in isolated and energy-insecure regions. These compact reactors operate by harnessing nuclear fission, generating heat that is converted into electricity through highly efficient cycles such as steam turbines or direct thermoelectric conversion. Their small, modular design allows for easy transportation and rapid deployment, ensuring that energy can be supplied to areas where traditional grid connections are unfeasible or impractical.
A key advantage of micro nuclear plants is their extraordinarily long operational lifespan. Unlike diesel generators that require constant refueling, micro nuclear plants can operate for years—sometimes a decade or more—without the need for intervention. This eliminates fuel supply chain vulnerabilities, reduces logistical costs, and ensures a continuous power supply in regions where fuel transport is expensive or unreliable, such as Arctic stations, offshore facilities, and deep-space missions. Moreover, these reactors produce zero direct carbon emissions, making them an environmentally friendly alternative to fossil fuel-based off-grid power systems. Advanced reactor designs, including high-temperature gas-cooled reactors, molten salt reactors, and liquid-metal-cooled reactors, have further increased efficiency, thermal output, and safety, allowing these systems to operate autonomously with minimal maintenance.
The ability to integrate micro nuclear plants into microgrids is another major advantage, enabling them to function alongside solar, wind, and battery storage to create a hybrid, decentralized energy network. By providing consistent baseload power, micro nuclear plants stabilize intermittent renewable energy sources, reducing reliance on expensive battery storage and ensuring uninterrupted electricity supply even in adverse weather conditions. This makes them an ideal solution for critical infrastructure, remote military bases, disaster relief zones, and high-energy industrial applications where energy reliability is non-negotiable.
As nuclear technology advances, next-generation microreactors will become even more compact, efficient, and safer, incorporating features such as passive cooling, digital automation, and enhanced fuel cycles. These innovations will further expand their applicability, ensuring that micro nuclear plants continue to lead the transition toward decentralized, carbon-free energy solutions. Whether deployed in remote villages, research stations, offshore oil platforms, or lunar colonies, micro nuclear reactors will define the future of off-grid power, offering a scalable, resilient, and sustainable solution for the world’s most challenging energy needs.
Compact nuclear reactors drive steam turbines
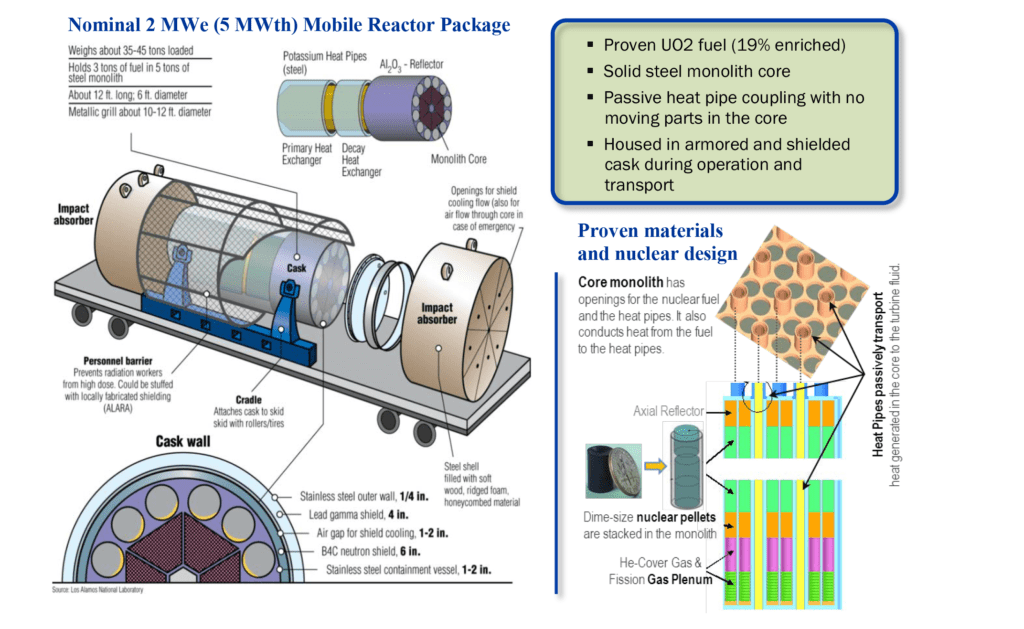
Compact nuclear reactors are increasingly being used to drive steam turbines, providing a highly efficient and reliable source of electricity for a variety of applications, from remote industrial operations to decentralized power grids. These small reactors generate heat through nuclear fission, which is then transferred to a working fluid—typically water or a specialized coolant—that produces high-pressure steam. This steam is directed into a steam turbine, where it expands and spins the turbine blades, converting thermal energy into mechanical energy. A connected generator then transforms this rotational motion into electricity, delivering a stable and continuous power supply.
One of the primary advantages of using compact nuclear reactors to drive steam turbines is their ability to produce consistent, high-output energy without reliance on fossil fuels. Unlike traditional coal or natural gas plants, compact nuclear systems do not emit carbon dioxide, making them a cleaner and more sustainable alternative. Additionally, these reactors require significantly less frequent refueling compared to conventional thermal power plants, with some designs capable of running for years or even decades on a single fuel load. This makes them particularly attractive for off-grid locations, where fuel transportation is challenging and costly.
Advancements in high-temperature gas-cooled reactors (HTGRs), molten salt reactors (MSRs), and liquid-metal-cooled reactors have further improved the efficiency and safety of steam-driven nuclear power systems. These technologies enable higher operating temperatures, which increase the efficiency of steam generation and turbine performance. Additionally, many modern compact nuclear reactors feature passive cooling systems, reducing the need for large amounts of water and making them viable for deployment in arid or water-scarce regions.
Beyond standalone applications, compact nuclear reactors driving steam turbines can be integrated into microgrids or hybrid energy systems, complementing renewable sources like solar and wind to ensure a steady baseload supply. Their scalability allows them to power anything from small communities and military bases to large industrial complexes and space exploration missions. As modular reactor designs continue to evolve, they will offer even greater flexibility, enabling faster deployment, lower costs, and enhanced safety features.
In the future, compact nuclear reactors will play a crucial role in decentralized power generation, offering high-efficiency, low-carbon, and long-lasting energy solutions for diverse applications. Their ability to drive steam turbines with minimal fuel requirements and maximum reliability ensures they will remain a key component of the global transition toward clean and resilient energy systems.
Compact nuclear reactors driving steam turbines are poised to revolutionize energy generation by providing a highly efficient, low-carbon, and resilient power source for various applications. These small-scale reactors generate immense heat through controlled nuclear fission, which is used to convert water into high-pressure steam. The steam then expands through multi-stage steam turbines, spinning their blades to produce mechanical energy, which is subsequently transformed into electricity via a generator. This process mimics the operation of large-scale nuclear power plants but is designed for greater flexibility, rapid deployment, and decentralized power generation.
One of the biggest advantages of using compact nuclear reactors for steam turbine operation is their ability to deliver continuous baseload power with minimal fuel requirements. Unlike conventional fossil-fuel plants that rely on constant fuel deliveries and combustion, compact nuclear systems operate with a single fuel load that can last several years, reducing logistical challenges and ensuring energy security. This is especially beneficial in remote locations, military installations, and industrial zones, where consistent power supply is critical. Additionally, since nuclear reactors do not burn fuel, they emit zero greenhouse gases, positioning them as a clean alternative in the global effort to reduce carbon emissions.
Advanced reactor designs, such as high-temperature gas-cooled reactors (HTGRs), molten salt reactors (MSRs), and liquid-metal-cooled reactors, have significantly enhanced the efficiency and safety of steam turbine-driven nuclear power. These next-generation technologies allow for higher operating temperatures and pressures, leading to improved steam cycle performance and greater energy conversion efficiency. Many of these modern designs incorporate passive safety features, such as self-regulating cooling systems, which eliminate the need for extensive water supplies and enhance overall operational reliability. This makes compact nuclear reactors ideal for water-scarce regions and environments where conventional cooling methods are impractical.
Beyond standalone applications, compact nuclear reactors integrated with microgrids and hybrid energy systems are reshaping decentralized energy production. These reactors can work alongside renewable sources like solar and wind, compensating for their intermittency and ensuring a stable electricity supply without requiring extensive battery storage. This ability to provide reliable baseload power while complementing renewables makes them a valuable solution for industries, governments, and communities seeking energy independence and resilience against grid failures and supply disruptions.
As innovations in reactor miniaturization, fuel efficiency, and digital automation continue, compact nuclear reactors will expand their role in off-grid and urban power solutions, offering a sustainable, long-term alternative to fossil fuel-based generation. Their ability to drive high-performance steam turbines with unparalleled efficiency ensures they will remain a cornerstone of the future energy mix, supporting everything from industrial facilities and data centers to remote settlements and space missions.
Compact nuclear reactors are increasingly recognized as a high-efficiency, long-term solution for powering steam turbines, delivering continuous, carbon-free electricity for diverse applications. These small-scale reactors generate heat through nuclear fission, which is transferred to a working fluid—typically water or molten salt—to create high-pressure steam. This steam then drives steam turbines with optimized thermodynamic cycles, converting thermal energy into rotational motion, which is subsequently transformed into electricity via generators. This well-established process, used in large-scale nuclear power plants, is now being adapted to smaller, modular, and transportable reactor designs that offer greater flexibility and faster deployment.
One of the primary advantages of using compact nuclear reactors for steam turbines is their high fuel efficiency and long operational life. Traditional fossil-fuel plants require constant fuel resupply, while compact nuclear reactors can operate for years—sometimes over a decade—without refueling. This extended fuel cycle makes them particularly well-suited for remote locations, industrial operations, military applications, and emergency backup power, where access to fuel supply chains is limited or costly. Unlike diesel generators, which are subject to fluctuating fuel prices and logistical constraints, compact nuclear reactors provide stable, predictable, and cost-effective energy over their lifespan.
Advancements in reactor technology have led to more efficient steam production, enhancing the performance of nuclear-driven steam turbines. High-temperature gas-cooled reactors (HTGRs), for instance, operate at significantly higher temperatures than traditional light-water reactors, improving the thermodynamic efficiency of the Rankine cycle. Molten salt reactors (MSRs) and liquid-metal-cooled reactors further optimize heat transfer, allowing for more compact turbine systems with higher energy conversion rates. Additionally, modern designs incorporate passive cooling mechanisms, eliminating the need for large cooling towers or extensive water supplies, making them ideal for arid regions and mobile applications.
The ability of compact nuclear reactors to integrate seamlessly into decentralized power systems is another key advantage. These reactors can function independently or as part of microgrids, providing resilient and stable baseload power in areas where traditional grids are unreliable or nonexistent. They can also complement renewable energy sources like wind and solar by stabilizing grid fluctuations, reducing the need for large-scale battery storage. This hybrid approach ensures maximum energy security and efficiency, particularly in regions with variable weather conditions or high energy demand fluctuations.
As technological innovations continue, the next generation of compact nuclear reactors will become even more efficient, safer, and cost-effective, expanding their role in global energy infrastructure. Their ability to power high-performance steam turbines with unmatched reliability positions them as a cornerstone technology for the future of clean energy, capable of supporting industrial production, remote settlements, space exploration, and emergency disaster response efforts.
Compact nuclear reactors are driving a transformation in energy generation by efficiently powering steam turbines to deliver stable, long-lasting, and carbon-free electricity. These advanced reactors generate immense heat through controlled nuclear fission, which is transferred to a working fluid—typically water, molten salt, or a gas like helium—to create high-pressure steam. This steam expands through turbine blades, converting thermal energy into rotational motion, which then drives a generator to produce electricity. Unlike conventional large-scale nuclear plants, compact nuclear reactors are designed for flexibility, rapid deployment, and off-grid power generation, making them a game-changer for remote locations, industrial applications, and mobile energy needs.
One of the greatest benefits of compact nuclear reactors in steam turbine applications is their inherent efficiency and long operational lifespan. Traditional power plants relying on coal, natural gas, or diesel require frequent fuel resupply, making them vulnerable to supply chain disruptions and fuel price fluctuations. In contrast, many compact nuclear reactors can operate for 10 years or more on a single fuel load, eliminating logistical challenges and reducing overall operating costs. This makes them an ideal solution for military bases, mining operations, Arctic research stations, offshore platforms, and even space exploration missions, where continuous, low-maintenance power is essential.
Recent advancements in reactor design have led to significant improvements in steam turbine efficiency. High-temperature gas-cooled reactors (HTGRs) operate at much higher temperatures than traditional pressurized water reactors, increasing thermal efficiency and reducing system complexity. Molten salt reactors (MSRs) and liquid-metal-cooled reactors provide even greater heat transfer efficiency, enabling smaller, more compact turbine-generator systems with optimized power output. Additionally, many of these modern reactor designs feature passive safety mechanisms, such as self-regulating cooling systems, reducing the need for human intervention and external cooling infrastructure. These advancements make compact nuclear reactors viable for deployment in extreme environments where conventional power plants would struggle to operate.
Beyond standalone energy generation, compact nuclear reactors are increasingly being integrated into hybrid energy systems and microgrids, enabling them to work in tandem with renewable sources like wind and solar. Because nuclear reactors provide a steady, uninterrupted power supply, they act as a stabilizing force in microgrid networks, balancing the intermittency of renewables and reducing the need for costly battery storage. This hybrid approach enhances energy resilience, making it particularly valuable in regions prone to natural disasters, extreme weather, or geopolitical instability.
As compact nuclear technology continues to evolve, its role in global energy infrastructure will only expand. The future of energy generation will increasingly depend on scalable, carbon-free, and highly efficient solutions, and compact nuclear reactors—driving high-performance steam turbines—are poised to become a critical component of the transition to a cleaner, more resilient power grid. Their ability to provide long-term, low-maintenance, and high-output power makes them a cornerstone technology for industries, governments, and communities seeking energy independence and sustainability in the decades to come.
Micro nuclear plants power remote areas
Micro nuclear plants are transforming energy access in remote areas by providing stable, long-lasting, and low-maintenance power solutions where traditional grid connections are impractical or non-existent. These small-scale reactors use nuclear fission to generate heat, which is converted into electricity through steam turbines or direct thermoelectric systems. Unlike diesel generators or renewable sources that rely on fuel supply chains or weather conditions, micro nuclear plants operate continuously for years on a single fuel load, ensuring uninterrupted power even in the most challenging environments.
One of the biggest advantages of micro nuclear plants is their compact, modular design, allowing for easy transportation and rapid deployment to isolated communities, Arctic stations, offshore platforms, mining operations, military bases, and disaster relief zones. These reactors eliminate the need for frequent fuel deliveries, reducing logistical costs and environmental risks. Their long operational lifespan—often exceeding a decade without refueling—makes them particularly valuable for locations where fuel transportation is costly or unreliable.
Modern micro nuclear reactors incorporate advanced safety features, including passive cooling systems and self-regulating reactor cores, minimizing the need for constant human oversight. These innovations make them well-suited for autonomous or lightly staffed operations, further increasing their viability in remote settings. Additionally, because they produce zero direct carbon emissions, micro nuclear plants offer a clean energy alternative to diesel and coal, supporting sustainability efforts while delivering reliable baseload power.
Beyond standalone use, micro nuclear plants can be integrated into microgrids, working alongside solar, wind, and battery storage to create resilient hybrid energy systems. This setup ensures stable electricity supply, compensating for the intermittency of renewables while reducing reliance on fossil fuels. As technology advances, next-generation microreactors will become even smaller, safer, and more efficient, making nuclear-powered remote energy solutions more accessible and cost-effective.
With their ability to provide consistent, scalable, and carbon-free power, micro nuclear plants are redefining energy independence for remote regions. Whether powering isolated villages, research facilities, industrial operations, or space missions, these reactors represent a reliable, sustainable, and future-ready solution for addressing global energy challenges.
Micro nuclear plants are revolutionizing energy access in remote areas by providing continuous, self-sustaining, and carbon-free electricity where traditional grid infrastructure is unavailable or impractical. These compact reactors generate heat through nuclear fission, which is then converted into electrical power using steam turbines or alternative heat conversion systems. Unlike conventional power sources that rely on frequent fuel deliveries or favorable weather conditions, micro nuclear plants operate for years without refueling, ensuring a stable and reliable power supply in even the most extreme environments.
One of the key advantages of micro nuclear plants is their small, modular design, which enables quick deployment and easy transport to remote islands, mining outposts, Arctic research stations, military bases, offshore drilling platforms, and disaster relief operations. Traditional power solutions in such areas often depend on diesel generators, which require constant fuel resupply and are susceptible to high fuel costs and logistical challenges. In contrast, micro nuclear plants offer decades-long operational capability on a single fuel load, drastically reducing maintenance and refueling requirements while providing a cost-stable energy alternative.
Modern micro nuclear technologies incorporate advanced passive safety systems, ensuring that these reactors can self-regulate and safely shut down in case of anomalies. Unlike older nuclear designs, many new microreactors use high-temperature gas-cooled, molten salt, or liquid-metal-cooled technologies, which provide higher efficiency and enhanced safety features. These improvements make them viable for unattended or minimally staffed operations, further reducing the operational burden in remote settings.
Beyond standalone deployment, micro nuclear plants are increasingly being integrated into microgrids, where they function as stable baseload power sources alongside renewable energy systems such as solar and wind. Because microreactors produce continuous electricity regardless of weather conditions, they stabilize hybrid energy networks, reducing reliance on expensive battery storage and diesel backup systems. This makes them particularly attractive for off-grid villages, industrial operations, and research facilities, where energy resilience and independence are critical.
As micro nuclear technology advances, next-generation reactors will become even smaller, more efficient, and more cost-effective, expanding their role in global energy solutions. These reactors represent a breakthrough in decentralized, long-term power generation, making remote settlements, industrial sites, and critical infrastructure less dependent on fossil fuels and vulnerable supply chains. With zero direct emissions and an extended operational lifespan, micro nuclear plants are paving the way for a sustainable, resilient, and energy-secure future in the world’s most challenging locations.
Micro nuclear plants are emerging as a transformative energy solution for remote regions, offering a stable, long-term, and carbon-free power source that operates independently of traditional fuel supply chains. These small-scale reactors utilize nuclear fission to generate heat, which is then converted into electricity through steam turbines or alternative heat conversion systems. Their ability to provide continuous, uninterrupted energy for years without refueling makes them particularly valuable for off-grid communities, military installations, research facilities, mining operations, and disaster recovery zones where reliable electricity is critical.
One of the key advantages of micro nuclear plants is their modular and transportable design, allowing for quick deployment and easy relocation. Traditional fossil fuel-based energy sources in remote areas, such as diesel generators, are heavily dependent on fuel deliveries, which are not only costly but also vulnerable to logistical disruptions, extreme weather, and geopolitical instability. In contrast, micro nuclear plants can function for a decade or more on a single fuel load, significantly reducing operational costs and minimizing supply chain risks. This is especially beneficial in harsh environments like the Arctic, deep-sea oil platforms, and isolated industrial zones, where access to conventional fuel sources is both difficult and expensive.
Modern microreactor designs are built with enhanced safety features, ensuring fail-safe operation with minimal human intervention. Many of these reactors utilize advanced cooling technologies, such as molten salt, liquid metal, or helium gas, which not only improve efficiency but also eliminate the need for large-scale water cooling infrastructure. This makes them particularly well-suited for desert regions, mountainous terrains, and other water-scarce environments. Additionally, passive safety mechanisms, such as self-regulating reactors and automated shutdown systems, further enhance reliability, reducing the risk of operational failures and making them ideal for unmanned or minimally staffed locations.
Beyond their standalone capabilities, micro nuclear plants are ideal for integration into microgrids, allowing them to function alongside solar, wind, and battery storage. Their ability to deliver consistent baseload power helps balance the intermittency of renewables, reducing reliance on expensive and environmentally damaging backup systems. This hybrid approach enhances grid stability, energy security, and cost efficiency, making micro nuclear technology a valuable asset for developing regions, remote infrastructure projects, and emergency response efforts.
As micro nuclear technology continues to advance, future generations of these reactors will become even more compact, affordable, and efficient, allowing for broader deployment in off-grid, rural, and industrial settings. Their long operational lifespan, minimal maintenance requirements, and zero direct emissions make them a sustainable and scalable energy solution for addressing the growing global demand for reliable, clean electricity. With the ability to operate independently for years, micro nuclear plants are redefining energy accessibility, resilience, and sustainability in the most challenging locations worldwide.
Micro nuclear plants are revolutionizing power generation in remote areas by offering long-term, stable, and carbon-free electricity where conventional energy sources struggle to operate. These small, self-sustaining nuclear reactors generate heat through nuclear fission, which is then used to produce steam that drives high-efficiency turbines, converting thermal energy into electricity. Their ability to run for years without refueling makes them ideal for isolated communities, military outposts, research stations, mining operations, and critical infrastructure projects, where a reliable power supply is essential.
Unlike diesel generators, which depend on constant fuel deliveries and are vulnerable to supply chain disruptions, micro nuclear plants provide continuous power without logistical constraints. Their modular, factory-built design enables rapid deployment and easy transportation to even the most challenging environments, including Arctic regions, islands, offshore facilities, and disaster-stricken areas. Once operational, these reactors require minimal maintenance, reducing the need for large staffing teams and ensuring greater energy security in isolated locations.
Micro nuclear reactors also come with advanced safety systems, incorporating passive cooling, self-regulating reactor cores, and meltdown-proof designs. Many utilize molten salt, gas-cooled, or liquid-metal cooling systems, eliminating the need for large external water supplies, which is particularly beneficial in desert or high-altitude regions. These next-generation designs ensure that micro nuclear plants can operate independently, safely, and efficiently, even in extreme environments.
Beyond standalone power generation, micro nuclear plants are being integrated into microgrids, providing a dependable baseload energy source alongside solar, wind, and battery storage. By ensuring continuous power availability, they reduce the reliance on fossil fuel backups and mitigate the intermittency issues of renewables, creating a more resilient and cost-effective energy system. This is especially valuable for developing regions, remote industries, and emergency response efforts, where energy stability is a top priority.
As technology progresses, future micro nuclear reactors will become even more compact, efficient, and affordable, enabling broader adoption in off-grid communities, industrial hubs, and critical infrastructure projects. Their ability to operate for decades without refueling, coupled with their zero direct emissions and minimal environmental impact, positions them as a key solution for sustainable, resilient, and accessible energy in the world’s most remote and energy-insecure regions.
Steam turbines make nuclear energy usable power
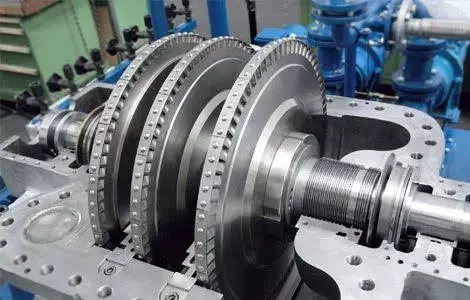
Steam turbines play a crucial role in converting nuclear energy into usable, reliable electricity by transforming the heat generated from nuclear fission into mechanical energy, which is then converted into electrical power. In a nuclear reactor, controlled fission reactions release vast amounts of heat, which is used to produce high-pressure steam from water. This steam is then directed onto the blades of a steam turbine, causing it to spin at high speeds. The turbine’s rotational energy is transferred to a generator, which produces the electricity that powers homes, industries, and cities.
Unlike fossil-fuel power plants, which burn coal or natural gas to create steam, nuclear power plants generate heat without carbon emissions, making them a clean and efficient energy source. The process relies on a closed-loop system, where the steam is condensed back into water and recirculated, ensuring minimal waste and maximum energy efficiency. Modern steam turbines in nuclear power plants are designed for high durability, precision, and efficiency, allowing them to operate continuously for years with minimal maintenance.
The efficiency of a nuclear steam turbine system is determined by several factors, including steam temperature, pressure, and turbine design. Many advanced nuclear plants use high-temperature reactors that improve thermal efficiency, allowing steam turbines to extract more energy from each unit of heat produced. Multi-stage turbines further enhance efficiency by expanding steam through multiple blade sections, maximizing power output.
In addition to traditional large-scale nuclear plants, small modular reactors (SMRs) and micro nuclear reactors are being developed with compact, high-efficiency steam turbine systems, allowing for decentralized, off-grid power generation. These smaller reactors, often designed for remote areas, industrial facilities, and military applications, rely on advanced turbine technology to convert nuclear heat into dependable, long-lasting electricity.
As the demand for clean and sustainable energy continues to grow, steam turbines will remain an essential component of nuclear power generation, ensuring that the heat from fission reactions is efficiently harnessed to produce safe, reliable, and carbon-free electricity for global energy needs.
Steam turbines are at the heart of nuclear power generation, transforming the intense heat from nuclear fission into mechanical energy that drives generators to produce usable electricity. This process begins in a nuclear reactor, where the controlled splitting of uranium or other fissile materials releases thermal energy, heating water into high-pressure steam. This steam is then directed at precision-engineered turbine blades, causing them to spin at high speeds. The rotational motion is transferred to a generator, where electromagnetic induction converts it into electrical power that can be distributed through the grid.
The efficiency of steam turbines in nuclear power plants depends on factors such as steam temperature, pressure, and turbine configuration. Most modern nuclear plants use multi-stage steam turbines, where steam expands through a series of high-, intermediate-, and low-pressure turbines before being condensed and recycled. This staged expansion maximizes energy extraction, improving overall efficiency. To further enhance performance, reheating and moisture-separation techniques are often used to maintain the quality of the steam and prevent turbine blade erosion.
Unlike fossil fuel plants that rely on combustion, nuclear reactors provide a continuous, emission-free heat source, ensuring that steam turbines operate at a steady and predictable rate. This makes nuclear power an ideal baseload energy provider, delivering stable electricity 24/7 without fluctuations in fuel availability. Additionally, the closed-loop cooling systems used in nuclear plants allow for efficient steam condensation and water recirculation, minimizing water waste and improving sustainability.
With advancements in nuclear technology, small modular reactors (SMRs) and micro nuclear reactors are incorporating compact, high-efficiency steam turbines designed for decentralized, flexible power generation. These smaller systems are particularly valuable for remote regions, industrial applications, and military installations, where access to reliable energy is limited. Some next-generation reactors, such as molten salt and high-temperature gas-cooled reactors, also aim to produce superheated steam, which can drive turbines at even higher efficiencies.
As the global demand for clean and sustainable energy continues to grow, steam turbines remain an essential component of nuclear power plants. Their ability to efficiently convert nuclear heat into usable electricity ensures that nuclear energy remains a stable, long-term solution for meeting industrial, commercial, and residential energy needs while reducing reliance on fossil fuels.
Steam turbines remain the key technology that makes nuclear energy a practical source of large-scale, reliable electricity. By harnessing the immense heat generated from nuclear fission, steam turbines efficiently convert thermal energy into rotational motion, which is then used to drive electric generators. This process allows nuclear power plants to supply continuous, stable electricity to national grids, making them a crucial part of the world’s baseload energy supply.
In a nuclear reactor, uranium or other fissile materials undergo controlled fission reactions, releasing massive amounts of heat. This heat is transferred to water, converting it into high-pressure steam. The steam is directed into a series of turbine stages, where it expands and accelerates through precisely engineered turbine blades. The rotational force generated in the turbine is then transferred via a shaft to an electrical generator, where electromagnetic induction produces high-voltage electricity for distribution.
To maximize efficiency, nuclear power plants use multi-stage steam turbines, where steam is expanded through a sequence of high-, intermediate-, and low-pressure turbines before being condensed back into water for reuse. The condensed water is then reheated in the reactor, creating a closed-loop system that ensures minimal waste and efficient steam utilization. Additionally, modern nuclear power plants use moisture separators, reheaters, and superheated steam cycles to improve the quality of steam and reduce energy losses.
Nuclear steam turbines are designed to operate continuously for years, providing a steady and predictable power output. Unlike fossil fuel plants, where fuel supply fluctuations and price volatility can impact electricity production, nuclear power plants benefit from long-term fuel stability, allowing them to maintain high operational efficiency with minimal interruptions. This makes nuclear-generated electricity cost-effective and resilient to market disruptions.
As nuclear technology advances, next-generation reactors are being developed to work with high-efficiency steam turbines. Concepts like supercritical water reactors, molten salt reactors, and high-temperature gas-cooled reactors aim to produce higher-temperature steam, which increases turbine efficiency and enhances power output. Additionally, small modular reactors (SMRs) and microreactors are integrating compact, lightweight steam turbines, allowing nuclear energy to be deployed in remote locations, industrial sites, and microgrids where traditional large-scale plants are impractical.
The future of nuclear power depends on continued advancements in steam turbine technology, ensuring that nuclear plants remain a high-efficiency, low-carbon energy solution. By continuously improving turbine designs, materials, and thermodynamic cycles, nuclear energy can remain one of the most reliable and sustainable sources of electricity, supporting global decarbonization efforts and long-term energy security.
Steam turbines continue to play a critical role in converting nuclear heat into usable electricity with high efficiency and reliability. The ability of nuclear power plants to generate continuous, large-scale energy relies on the seamless operation of steam-driven turbine systems, making them a cornerstone of modern nuclear energy production. By using high-pressure steam produced from nuclear fission, these turbines extract thermal energy and transform it into mechanical motion, which is then converted into electrical power for widespread distribution.
At the core of this process is the nuclear reactor, where uranium, plutonium, or thorium fuel undergoes controlled fission, releasing intense heat. This heat is transferred to a coolant, typically water, which absorbs the energy and turns into high-pressure steam in the steam generator or reactor vessel. The steam is then directed onto the blades of a steam turbine, causing it to spin at speeds of thousands of revolutions per minute. The rotating turbine is connected to an electrical generator, which produces electricity through electromagnetic induction, supplying power to homes, industries, and infrastructure.
To enhance efficiency, modern nuclear power plants utilize multi-stage steam turbines, in which steam expands through high-, intermediate-, and low-pressure stages to extract as much energy as possible before being condensed back into liquid form. This closed-loop system ensures minimal waste and allows for efficient steam recycling, improving overall plant performance. Advanced nuclear reactors are also exploring superheated and supercritical steam cycles, which enable higher operating temperatures and improve turbine efficiency, reducing thermal energy losses.
The durability and longevity of steam turbines in nuclear plants are key factors in their widespread use. Unlike fossil fuel-based turbines, which experience fluctuations in fuel supply and combustion-related wear, nuclear steam turbines benefit from a steady, long-term fuel source, allowing for uninterrupted operation for years with minimal maintenance. This stability makes nuclear power a reliable baseload energy source, capable of delivering constant electricity output without carbon emissions.
Beyond conventional large-scale reactors, small modular reactors (SMRs) and microreactors are emerging as next-generation solutions for decentralized power. These smaller nuclear systems integrate compact steam turbines, designed to efficiently convert nuclear heat into electricity on a smaller scale. Such reactors are being developed for remote locations, industrial applications, and off-grid power solutions, offering flexible, low-carbon alternatives to traditional energy sources.
As nuclear energy continues to evolve, the role of steam turbines will remain essential in ensuring the efficient conversion of nuclear heat into practical, sustainable electricity. Advancements in turbine materials, thermal efficiency, and integrated cooling systems will further optimize nuclear power plants, making them even more efficient, reliable, and adaptable to future energy needs. By refining steam turbine technology, nuclear energy will continue to be a cornerstone of global clean power generation, supporting long-term energy security and decarbonization efforts.
Electricity from nuclear heat via steam turbines
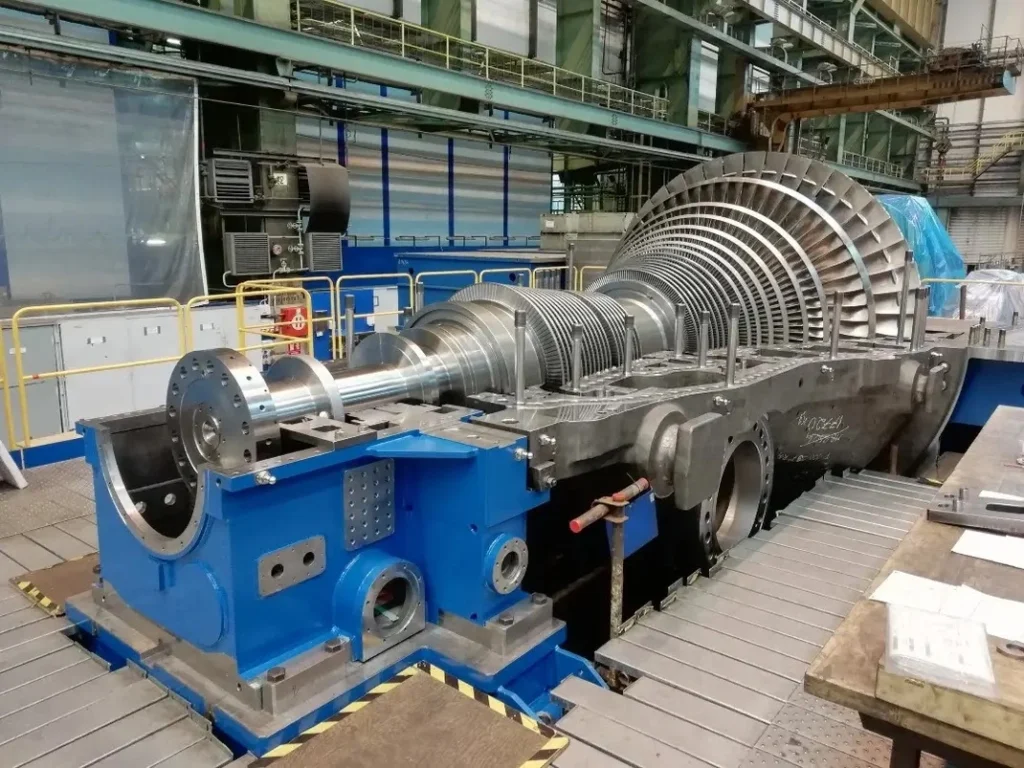
Electricity generation from nuclear heat via steam turbines is a highly efficient process that enables continuous, large-scale power production with zero carbon emissions. This method relies on nuclear fission, where atoms of uranium, plutonium, or thorium split within a controlled reactor environment, releasing enormous amounts of heat energy. This heat is transferred to a coolant—typically water—which then boils into high-pressure steam. The steam is directed onto the blades of a steam turbine, causing it to spin at high speeds. The mechanical motion from the spinning turbine is then converted into usable electricity through an electrical generator, which sends power into the grid for industrial, commercial, and residential use.
The efficiency of this process is optimized through multi-stage steam turbines, where steam expands through a series of high-, intermediate-, and low-pressure turbines before being condensed back into water. This closed-loop system allows for steam recycling, ensuring minimal energy waste and continuous electricity production. Additionally, advanced nuclear reactors are incorporating superheated steam cycles and supercritical water reactors, which operate at higher temperatures and pressures to improve overall energy conversion efficiency.
Nuclear power plants provide a stable and reliable baseload energy source, unlike renewable sources such as solar and wind, which can be intermittent. Because nuclear fuel lasts for years, steam turbines in nuclear plants can operate continuously with minimal refueling, making them a cost-effective, long-term power solution.
With the development of small modular reactors (SMRs) and microreactors, steam turbines are being adapted for smaller-scale, decentralized nuclear power solutions. These advanced systems enable off-grid electricity production, making nuclear power accessible for remote locations, industrial sites, and microgrids. Future innovations in high-efficiency turbine design and thermal energy management will continue to enhance the performance of nuclear power plants, ensuring sustainable, carbon-free electricity for the future.
Electricity generation from nuclear heat via steam turbines remains one of the most efficient and reliable methods of producing large-scale, continuous power. By harnessing the immense heat from nuclear fission reactions, this process enables the transformation of thermal energy into mechanical motion, which is then converted into electricity. This method has been the foundation of commercial nuclear power plants for decades and continues to evolve with advanced reactor designs and turbine technologies.
At the heart of this process is the nuclear reactor, where fissionable material such as uranium or plutonium undergoes controlled splitting of atomic nuclei. This reaction releases enormous amounts of heat, which is transferred to a coolant—typically water, molten salt, or gas—depending on the reactor type. In most conventional nuclear power plants, the heat energy boils water into steam, which is then used to drive high-efficiency steam turbines. The expanding steam spins the turbine blades at thousands of revolutions per minute, converting the thermal energy into rotational kinetic energy.
This rotational motion is transferred to a generator, where electromagnetic induction takes place. As the generator spins, it produces high-voltage electricity, which is then transformed and distributed through the power grid. The steam, having completed its work in the turbine, is cooled down in a condenser, converting it back into liquid water, which is then recirculated into the system. This closed-loop process maximizes efficiency and minimizes waste, making nuclear power one of the most effective energy sources.
To optimize energy extraction, modern nuclear power plants utilize multi-stage steam turbines that incorporate high-pressure, intermediate-pressure, and low-pressure sections. This allows the steam to expand gradually, extracting as much energy as possible before it is condensed and reused. Moisture separators and reheaters are also integrated into many systems to enhance the steam quality, prevent erosion of turbine blades, and improve overall performance.
One of the key advantages of nuclear-powered steam turbines is their steady and uninterrupted power output. Unlike fossil fuel plants, which are subject to fuel price fluctuations and supply chain issues, nuclear reactors operate with long-term fuel availability, allowing them to generate power for years without interruption. Additionally, because nuclear fission does not produce carbon emissions, these plants provide clean and sustainable electricity, supporting global efforts to reduce reliance on fossil fuels.
With the development of next-generation nuclear reactors, including small modular reactors (SMRs) and microreactors, steam turbines are being adapted for compact, decentralized energy solutions. These smaller, factory-built reactors integrate high-efficiency steam turbine systems that can be deployed in remote locations, industrial sites, and microgrids, enabling reliable off-grid electricity. Some advanced designs, such as supercritical water reactors and molten salt reactors, aim to produce higher-temperature steam, further increasing turbine efficiency and electricity output.
As nuclear energy continues to evolve, improvements in steam turbine materials, aerodynamics, and thermal cycle optimization will ensure that nuclear plants remain a cornerstone of global clean energy production. With a combination of high reliability, energy density, and zero-carbon emissions, nuclear steam turbines will continue to provide efficient, large-scale electricity generation for decades to come.
Electricity generation from nuclear heat via steam turbines remains one of the most advanced and efficient methods of producing large-scale, continuous, and low-carbon power. By converting the immense heat from nuclear fission into high-pressure steam, steam turbines enable the extraction of maximum energy from the thermal cycle, ensuring high-efficiency power production. This technology forms the backbone of commercial nuclear power plants and is continually being refined to enhance performance, reduce operational costs, and increase sustainability.
The process begins in a nuclear reactor, where fissionable material such as uranium-235 or plutonium-239 undergoes a controlled chain reaction. As the atomic nuclei split, they release massive amounts of heat energy, which is transferred to a coolant—typically water in pressurized or boiling water reactors (PWRs and BWRs). In most traditional designs, this heat energy is used to convert water into high-pressure steam, which is then directed onto the blades of a steam turbine. The force of the steam drives the turbine blades at high rotational speeds, converting thermal energy into mechanical motion.
The rotating turbine shaft is connected to an electrical generator, where the mechanical energy is converted into usable electricity through electromagnetic induction. This process ensures that nuclear power plants can generate massive amounts of electricity efficiently and consistently. The exhausted steam, having released most of its energy, is then cooled and condensed back into liquid water using a heat exchanger or cooling system, such as a wet cooling tower, dry cooling system, or natural water body. The condensed water is then recirculated into the reactor system, creating a closed-loop cycle that minimizes waste and conserves resources.
To maximize efficiency, nuclear power plants are equipped with multi-stage steam turbines, which consist of high-, intermediate-, and low-pressure sections. This staged approach allows steam to expand gradually through the turbine, extracting as much energy as possible before it reaches the condenser. Many plants also incorporate moisture separators and reheaters to maintain steam quality, prevent turbine blade erosion, and improve thermodynamic efficiency.
A key advantage of nuclear-generated electricity is its ability to provide consistent, baseload power. Unlike renewable sources such as wind and solar, which can experience fluctuations due to weather conditions, nuclear steam turbines operate continuously and reliably, making them an ideal foundation for national energy grids. Additionally, because nuclear fission does not produce carbon dioxide emissions, nuclear power is a critical component of global clean energy strategies aimed at reducing dependence on fossil fuels.
With the rise of advanced nuclear reactors, including small modular reactors (SMRs) and microreactors, steam turbines are being downsized and optimized for decentralized, flexible power generation. These next-generation reactors incorporate compact, high-efficiency turbines that can be used in remote areas, industrial settings, and military applications, where traditional large-scale power plants are impractical. Some emerging reactor designs, such as molten salt reactors and supercritical water reactors, operate at higher temperatures, allowing for improved steam cycles and greater energy conversion efficiency.
As the global energy landscape continues to evolve, ongoing advancements in turbine technology—including better materials, aerodynamics, and thermal cycle optimizations—will further enhance the efficiency and longevity of nuclear power plants. By refining the design of steam turbines and associated thermal systems, nuclear energy will remain a cornerstone of clean, high-efficiency electricity generation, supporting efforts to achieve long-term energy security and decarbonization goals.
Electricity generation from nuclear heat via steam turbines remains one of the most efficient and scalable methods of producing continuous, carbon-free power. The process relies on harnessing thermal energy from controlled nuclear fission reactions, converting it into mechanical motion through steam turbines, and ultimately transforming it into electrical energy via generators. This technology has been a cornerstone of global energy infrastructure for decades and continues to evolve with advancements in reactor designs, turbine efficiency, and thermal cycle optimization.
The fundamental principle of nuclear steam power begins in the reactor core, where uranium or plutonium fuel undergoes fission, releasing tremendous amounts of heat. This heat is absorbed by a coolant medium, typically water in light-water reactors (such as PWRs and BWRs), or in advanced designs, liquid metal, molten salt, or supercritical CO₂. In conventional systems, the coolant transfers the heat to a steam generator, which converts water into high-pressure steam. The expanding steam is then directed onto turbine blades, causing the turbine to spin at thousands of revolutions per minute.
The rotational motion of the turbine is mechanically coupled to an electrical generator, where it induces an electrical current. This high-voltage electricity is then stepped up via transformers and distributed to power grids for industrial, commercial, and residential consumption. Once the steam has passed through the turbine, it enters a condenser, where it is cooled and converted back into liquid water, completing the closed-loop cycle. This ensures minimal waste and continuous steam recycling, making nuclear plants highly efficient and sustainable.
To further enhance performance, modern nuclear power plants use multi-stage steam turbines. These systems feature high-, intermediate-, and low-pressure sections, allowing for progressive energy extraction as steam expands and cools. Reheating and moisture separation technologies are also integrated to improve steam quality, prevent erosion, and optimize thermodynamic efficiency.
One of the most significant advantages of nuclear-powered steam turbines is their ability to provide stable baseload electricity. Unlike fossil fuel plants, which are subject to supply fluctuations and carbon emissions, or renewables like wind and solar, which depend on weather conditions, nuclear plants operate continuously for months or even years without refueling. This makes them an essential component of national energy security and decarbonization efforts.
With the rise of advanced nuclear technologies, including small modular reactors (SMRs) and microreactors, steam turbine technology is being adapted for flexible and decentralized power generation. These compact, factory-built reactors integrate high-efficiency turbine systems, enabling on-demand electricity production in remote locations, industrial facilities, and microgrids. Advanced designs, such as molten salt reactors and high-temperature gas-cooled reactors, allow for higher steam temperatures and pressures, increasing turbine efficiency and enabling direct thermal applications like desalination and hydrogen production.
Looking ahead, innovations in turbine materials, aerodynamics, and thermal cycle integration will further optimize the efficiency of nuclear plants. New advancements in supercritical CO₂ turbines and closed Brayton cycle systems promise higher energy conversion rates, reducing plant footprint while increasing output. As nuclear energy continues to evolve, steam turbines will remain a key enabler of clean, reliable, and large-scale electricity generation, reinforcing their role in the global transition toward low-carbon energy solutions.
Steam Turbines Transform Nuclear Heat into Energy
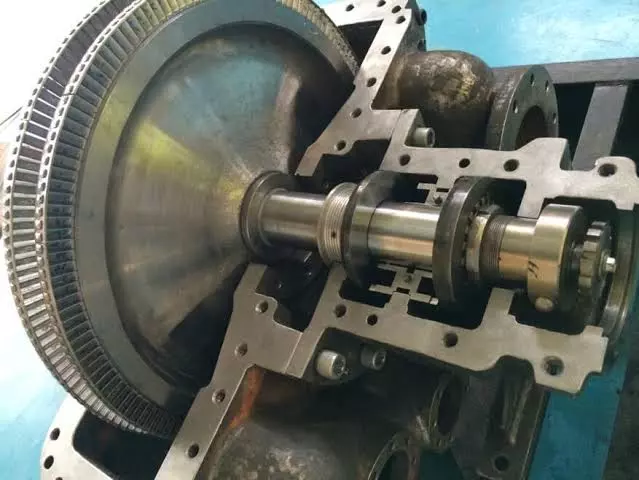
Steam turbines play a vital role in converting nuclear heat into usable electrical energy, forming the backbone of nuclear power generation. The process starts with nuclear fission, where atoms of uranium-235 or plutonium-239 split in a controlled reaction inside the reactor core, releasing massive amounts of heat energy. This heat is absorbed by a coolant, typically water in conventional reactors or advanced molten salt and gas-cooled systems. In pressurized water reactors (PWRs) and boiling water reactors (BWRs), this heat is used to generate steam, which is then directed toward steam turbines to produce electricity.
High-pressure superheated steam is channeled through the turbine blades, causing them to rotate at thousands of revolutions per minute. The spinning turbine is connected to an electrical generator, where mechanical energy is transformed into electricity through electromagnetic induction. The exhausted steam is then cooled and condensed back into water using a condenser, completing a closed-loop cycle that maximizes efficiency and minimizes water loss.
To optimize performance, modern nuclear power plants employ multi-stage steam turbines with high-, intermediate-, and low-pressure sections, allowing for gradual energy extraction from the expanding steam. Some plants integrate reheaters and moisture separators to enhance steam quality and prevent turbine blade erosion, ensuring long-term reliability.
Unlike fossil-fuel plants, which rely on combustion, nuclear plants provide a stable, carbon-free power source that is not dependent on weather conditions, making them a key solution for baseload electricity generation. With advancements in small modular reactors (SMRs) and microreactors, steam turbine technology is being scaled down and optimized for decentralized energy systems, enabling nuclear power to be deployed in remote locations, industrial sites, and microgrids.
Looking to the future, innovations in supercritical CO₂ turbines, high-efficiency thermal cycles, and advanced materials will further improve conversion efficiency and sustainability. As nuclear energy continues to evolve, steam turbines will remain at the heart of nuclear power plants, ensuring reliable, efficient, and large-scale electricity production for a low-carbon world.
Steam Turbines Transform Nuclear Heat into Energy
Steam turbines remain at the core of nuclear power generation, efficiently converting thermal energy from nuclear fission into mechanical motion and, ultimately, electricity. The process begins within the nuclear reactor, where controlled fission reactions in fuel rods—typically containing uranium-235 or plutonium-239—release immense heat. This heat is absorbed by a coolant, usually water, molten salt, or gas, depending on the reactor design. In most conventional reactors, this thermal energy is transferred to a secondary water loop, generating high-pressure steam without direct contact with radioactive materials.
The steam is then directed into a high-efficiency steam turbine, where it expands rapidly, imparting kinetic energy to the turbine blades, causing them to spin at thousands of revolutions per minute. This rotational energy is transferred to an electrical generator, where it is converted into electricity via electromagnetic induction. The spent steam, now at a lower pressure, is sent to a condenser, where it is cooled and converted back into water using a heat exchanger or cooling tower. The condensed water is then recirculated into the system, making it a closed-loop cycle that minimizes waste and optimizes efficiency.
Modern nuclear plants use multi-stage steam turbines, which consist of high-, intermediate-, and low-pressure sections. These turbines extract maximum energy by gradually expanding and cooling the steam as it passes through successive turbine stages. Reheaters and moisture separators are integrated into many designs to improve steam quality, reduce blade erosion, and increase efficiency.
One of the most significant advantages of nuclear-powered steam turbines is their ability to deliver consistent, large-scale baseload electricity. Unlike solar and wind power, which can be intermittent, nuclear plants provide continuous and predictable power generation, making them a critical component of national energy grids. Furthermore, because nuclear fission does not produce direct carbon emissions, it serves as a low-carbon alternative to fossil fuels, supporting global decarbonization efforts.
The next generation of nuclear reactors, including small modular reactors (SMRs) and microreactors, is further advancing steam turbine applications. These compact, factory-built reactors incorporate highly efficient turbine systems, enabling off-grid power generation in remote areas, industrial facilities, and military installations. Advanced designs, such as molten salt reactors and high-temperature gas-cooled reactors, allow for higher operating temperatures, increasing turbine efficiency and power output.
Looking ahead, emerging innovations such as supercritical CO₂ turbines, advanced Brayton cycle systems, and high-performance turbine materials will further enhance the efficiency and reliability of nuclear power plants. As nuclear energy continues to evolve, steam turbines will remain a key component of sustainable, large-scale electricity generation, ensuring a stable and clean energy future.
Steam Turbines Transform Nuclear Heat into Energy
Steam turbines are the critical link between nuclear reactors and the electricity grid, ensuring that the immense heat produced by nuclear fission is efficiently converted into usable electrical energy. This process relies on precisely engineered thermodynamic cycles, where high-temperature steam drives multi-stage turbine systems, ultimately producing a stable, high-output power source.
In a nuclear reactor, the fission of uranium-235 or plutonium-239 atoms releases a tremendous amount of thermal energy. This heat is absorbed by a coolant, which in most traditional reactors—such as pressurized water reactors (PWRs) and boiling water reactors (BWRs)—is water. In PWRs, the coolant remains under high pressure to prevent boiling, and the heat is transferred to a steam generator, creating a secondary loop of pressurized steam that powers the turbine. In BWRs, water directly boils within the reactor vessel, generating steam that is sent straight to the turbines.
The superheated, high-pressure steam enters the steam turbine, where it expands and accelerates, striking the turbine’s blades and causing them to rotate at thousands of revolutions per minute. This mechanical motion is transferred to an electrical generator, where it is converted into usable electricity via electromagnetic induction. As the steam moves through the turbine, it gradually loses pressure and temperature, transferring its energy in stages. To maximize efficiency, nuclear plants utilize multi-stage turbines, which include high-, intermediate-, and low-pressure sections, ensuring the most effective conversion of thermal energy into mechanical work.
Once the steam has passed through the turbine, it moves into a condenser, where it is cooled down—often using a heat exchanger, cooling tower, or natural water source—and converted back into liquid water. This water is then pumped back into the reactor system, creating a closed-loop cycle that conserves resources and maintains efficiency. Some plants incorporate reheaters and moisture separators, which improve steam quality, reduce turbine blade erosion, and optimize long-term performance.
One of the greatest strengths of nuclear-powered steam turbines is their ability to provide stable, continuous, large-scale electricity production. Unlike renewable sources such as solar and wind, which can experience intermittent generation, nuclear power plants deliver constant baseload power, ensuring grid stability and energy security. Moreover, nuclear fission produces zero direct carbon emissions, making it a crucial technology for reducing reliance on fossil fuels and combating climate change.
The rise of advanced nuclear reactors, including small modular reactors (SMRs) and microreactors, is bringing steam turbine technology into new, decentralized energy applications. These reactors feature smaller, highly efficient turbine designs that enable deployment in remote areas, industrial sites, and microgrids. Additionally, some next-generation reactors—such as molten salt reactors (MSRs) and high-temperature gas-cooled reactors (HTGRs)—operate at higher thermal efficiencies, producing steam at higher temperatures and pressures, further increasing turbine output.
Looking ahead, ongoing advancements in supercritical CO₂ turbines, advanced Brayton cycle systems, and high-performance turbine materials are poised to further improve nuclear energy conversion efficiency. As nuclear power continues to evolve, steam turbines will remain a fundamental component of sustainable, large-scale electricity generation, ensuring a reliable, carbon-free energy future for global power systems.
Steam Turbines Transform Nuclear Heat into Energy
Steam turbines are at the heart of nuclear power generation, converting immense thermal energy from nuclear fission into reliable electricity. This process relies on the fundamental principles of thermodynamics and fluid dynamics, where heat is used to produce high-pressure steam that drives turbines, creating mechanical power that is then converted into electrical energy.
The process begins within the nuclear reactor core, where uranium-235 or plutonium-239 undergoes fission, splitting atoms and releasing intense heat. This heat is transferred to a coolant, typically water in light-water reactors (LWRs), which then produces high-temperature steam in a controlled system. In pressurized water reactors (PWRs), the coolant remains under extreme pressure to prevent boiling, and heat is exchanged through steam generators to create a separate loop of pressurized steam. In boiling water reactors (BWRs), water boils directly in the reactor, generating steam that flows directly to the turbines.
Once generated, the superheated steam is directed through high-pressure steam turbines, where it expands and accelerates, striking turbine blades and causing them to spin at high rotational speeds. The turbine shaft is connected to an electrical generator, where this mechanical energy is converted into usable electricity through electromagnetic induction.
To maximize efficiency, nuclear power plants use multi-stage turbines, consisting of high-, intermediate-, and low-pressure sections. As steam passes through each stage, it gradually cools and expands, transferring energy at different pressure levels. Reheaters and moisture separators improve steam quality by preventing condensation, which can cause turbine blade erosion. This system ensures that nuclear plants extract the maximum possible energy from the steam before it reaches the condenser, where it is cooled and returned to liquid form for recycling in a closed-loop cycle.
Nuclear-powered steam turbines have a key advantage over fossil fuel-based turbines because they operate continuously, without the need for constant fuel replenishment. Unlike coal or gas plants, which are subject to fuel price fluctuations and supply chain issues, nuclear reactors use long-lasting fuel rods that can sustain operations for months or even years before refueling is needed. This makes nuclear energy a stable and predictable source of baseload electricity, capable of supporting large-scale industrial and national grid demands.
Furthermore, nuclear steam turbines play a crucial role in global decarbonization efforts. Since nuclear plants do not burn fossil fuels, they produce zero direct carbon emissions, making them a key component of clean energy strategies. As countries seek to reduce greenhouse gas emissions and transition away from coal and natural gas, nuclear power remains an essential low-carbon energy source that can support large-scale electrification while complementing intermittent renewables like wind and solar.
With the rise of advanced nuclear technologies, including small modular reactors (SMRs) and microreactors, steam turbine systems are being reimagined for decentralized energy generation. These compact reactors integrate high-efficiency steam turbines into smaller, modular designs, enabling flexible deployment in remote locations, industrial zones, and off-grid applications. Next-generation reactors, such as molten salt reactors (MSRs), high-temperature gas-cooled reactors (HTGRs), and supercritical water reactors (SCWRs), aim to increase steam temperatures and pressures, boosting turbine efficiency and overall power output.
Looking to the future, advances in supercritical CO₂ turbines, closed Brayton cycle systems, and high-performance turbine materials will further enhance the efficiency, reliability, and sustainability of nuclear power plants. As nuclear technology continues to evolve, steam turbines will remain an indispensable element of large-scale energy production, ensuring a stable, carbon-free power supply for the global energy grid.
Nuclear Plants Rely on Steam Turbines for Power
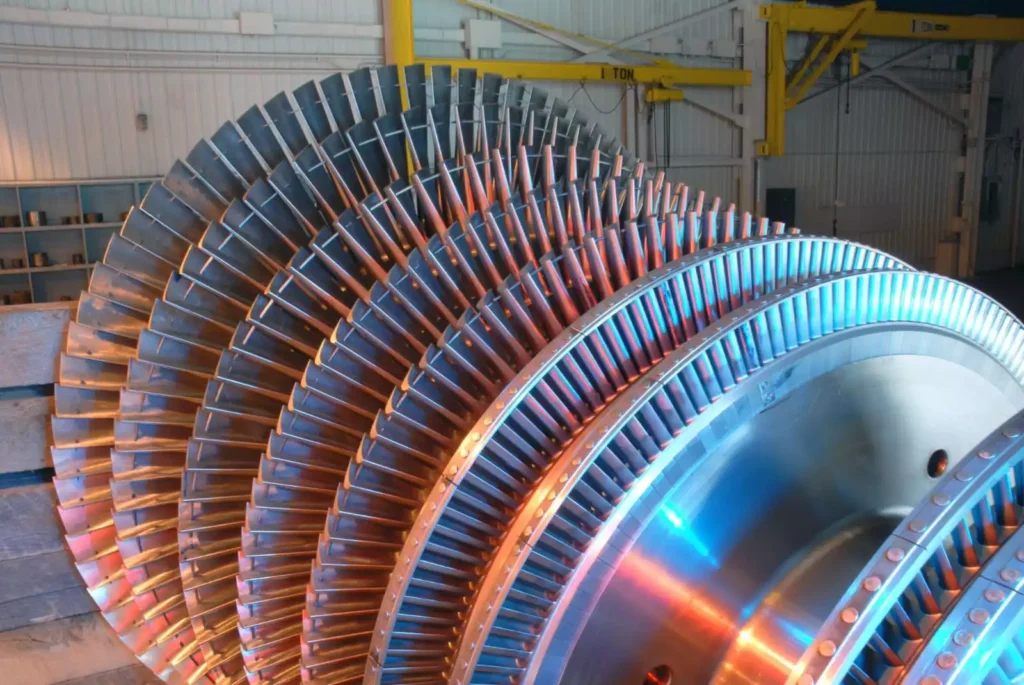
Nuclear power plants depend on steam turbines as the primary mechanism for converting nuclear heat into usable electricity. The process begins inside the reactor core, where nuclear fission splits atoms of uranium-235 or plutonium-239, releasing an immense amount of heat. This thermal energy is transferred to a coolant, usually water, which is then used to generate high-pressure steam. This steam is directed into a steam turbine, where it expands and causes the turbine blades to rotate at high speeds. The turbine’s spinning shaft is connected to an electrical generator, where mechanical motion is converted into electricity through electromagnetic induction.
To maximize efficiency, nuclear power plants use multi-stage steam turbines, which include high-, intermediate-, and low-pressure sections. As the steam passes through each stage, its energy is gradually extracted, allowing for optimized power generation. After passing through the turbine, the steam moves into a condenser, where it is cooled and converted back into liquid water before being pumped back into the system, completing a closed-loop cycle.
One of the key advantages of nuclear-powered steam turbines is their ability to provide continuous and reliable baseload power. Unlike solar and wind energy, which are intermittent and depend on weather conditions, nuclear plants operate 24/7, ensuring a stable electricity supply. Additionally, nuclear fission produces zero direct carbon emissions, making it a low-carbon alternative to fossil fuels.
As nuclear technology advances, next-generation reactors such as small modular reactors (SMRs) and microreactors are being developed with high-efficiency turbine systems. These compact reactors can be deployed in remote areas, industrial facilities, and microgrids, offering flexible and decentralized power solutions. Innovations in supercritical CO₂ turbines, advanced Brayton cycle systems, and high-performance turbine materials are further enhancing the efficiency and sustainability of nuclear power plants.
With growing global demand for clean and reliable energy, steam turbines will continue to be an essential component of nuclear power generation, ensuring a stable and sustainable energy future.
Nuclear Plants Rely on Steam Turbines for Power
Steam turbines serve as the essential link between nuclear reactors and the electrical grid, ensuring that the immense thermal energy produced by nuclear fission is efficiently converted into electricity. The process starts inside the reactor core, where controlled fission reactions release intense heat. This heat is transferred to a coolant—typically water in most commercial reactors—which is then used to generate high-pressure steam.
This superheated steam is directed into a steam turbine, where it expands rapidly and causes the turbine blades to rotate at high speeds. The turbine’s spinning shaft is mechanically connected to an electrical generator, which converts the kinetic energy of the rotating turbine into usable electricity through electromagnetic induction. The steam, having transferred its energy, then moves into a condenser, where it is cooled and returned to liquid form for recirculation in a closed-loop system.
Most nuclear power plants utilize multi-stage turbines, consisting of high-, intermediate-, and low-pressure sections to extract maximum energy from the steam. Some systems also include moisture separators and reheaters to enhance efficiency by preventing condensation and maintaining optimal steam conditions. These design improvements help extend the lifespan of turbine blades, improve power output, and ensure long-term reliability.
The key advantage of nuclear-powered steam turbines is their ability to generate continuous, large-scale baseload power. Unlike intermittent renewable sources such as solar and wind, which depend on weather conditions, nuclear power plants can operate 24/7, providing a stable and predictable electricity supply. Furthermore, because nuclear fission does not produce direct carbon emissions, nuclear energy is a low-carbon alternative to fossil fuels, contributing to global climate change mitigation efforts.
With the advancement of next-generation nuclear reactors, including small modular reactors (SMRs) and microreactors, steam turbine technology is being adapted for smaller, more flexible power generation systems. These advanced reactors incorporate compact, high-efficiency turbine designs that enable deployment in off-grid locations, industrial zones, and decentralized power systems. Additionally, emerging technologies such as supercritical CO₂ turbines, advanced Brayton cycle systems, and high-temperature gas-cooled reactors (HTGRs) aim to increase the thermal efficiency of nuclear power plants, reducing operational costs and improving overall performance.
As the global demand for clean, reliable energy continues to grow, steam turbines will remain a cornerstone of nuclear power generation, ensuring a sustainable and resilient energy future for both large-scale grids and smaller, independent power systems.
Nuclear Plants Rely on Steam Turbines for Power
Steam turbines play a critical role in nuclear power generation, acting as the primary means of converting thermal energy from nuclear fission into mechanical energy and ultimately electricity. The process begins within the reactor core, where controlled fission reactions of uranium-235 or plutonium-239 release immense heat. This heat is transferred to a coolant—typically water in pressurized water reactors (PWRs) and boiling water reactors (BWRs)—which then generates high-pressure steam.
The steam is then directed into a steam turbine, where it expands and pushes against a series of blades, causing the turbine shaft to rotate at high speeds. This mechanical motion is transferred to an electrical generator, where it is converted into electricity through electromagnetic induction. To optimize energy extraction, nuclear plants use multi-stage turbines, which include high-pressure, intermediate-pressure, and low-pressure stages. These stages allow the steam to gradually release its energy while maintaining efficient power production.
After passing through the turbine, the steam enters a condenser, where it is cooled using water from cooling towers, lakes, or oceans. The cooled steam condenses back into liquid water and is pumped back into the reactor system, completing a closed-loop cycle. This design ensures minimal waste and efficient resource utilization, making nuclear power one of the most energy-dense and sustainable forms of electricity generation.
One of the primary advantages of nuclear-powered steam turbines is their ability to provide constant baseload power. Unlike renewable energy sources such as solar and wind, which are dependent on weather conditions, nuclear plants operate continuously and deliver a stable supply of electricity to the grid. Additionally, nuclear fission does not emit carbon dioxide (CO₂) during power generation, making it a key solution for reducing global greenhouse gas emissions.
As nuclear technology advances, small modular reactors (SMRs) and microreactors are emerging as flexible, scalable alternatives to traditional large nuclear plants. These next-generation reactors integrate high-efficiency steam turbines into compact designs, allowing them to be deployed in remote locations, industrial facilities, and decentralized power grids. Innovations in supercritical CO₂ turbines, advanced Brayton cycle systems, and high-temperature reactor designs further enhance the efficiency and adaptability of nuclear power for various applications.
Looking ahead, steam turbines will continue to be a cornerstone of nuclear power generation, supporting the global shift toward clean, reliable, and low-carbon energy. As the demand for sustainable energy solutions grows, nuclear-powered steam turbines will remain a key technology in ensuring long-term energy security and grid stability.
Nuclear Plants Rely on Steam Turbines for Power
Steam turbines are the workhorses of nuclear power plants, responsible for converting thermal energy from nuclear fission into mechanical power and then into electricity. The process starts deep within the reactor core, where controlled fission reactions release immense heat. This heat is used to generate high-pressure steam, which is directed into a steam turbine system designed for maximum efficiency and energy extraction.
Once the superheated steam enters the turbine, it expands rapidly, causing rotational motion of the turbine blades. These blades are arranged in a multi-stage configuration, allowing the steam to gradually transfer its energy through high-pressure, intermediate-pressure, and low-pressure sections. The turbine is mechanically connected to an electrical generator, where the rotational motion induces an electric current, ultimately delivering power to the grid.
After completing its journey through the turbine, the steam enters a condenser, where it is cooled down and returned to liquid form. This condensed water is then pumped back into the reactor system, ensuring a continuous and closed-loop cycle that enhances efficiency and minimizes water waste. Some nuclear plants incorporate reheaters and moisture separators to further optimize steam conditions, improving overall turbine performance and extending the lifespan of critical components.
One of the greatest strengths of nuclear-powered steam turbines is their ability to provide constant, large-scale electricity generation. Unlike fossil-fuel power plants, which rely on continuous fuel combustion, or renewable energy sources, which are subject to weather fluctuations, nuclear plants operate 24/7, supplying stable and predictable baseload power. This reliability makes nuclear energy a foundational element of modern electrical grids, ensuring uninterrupted power for industries, businesses, and residential areas.
Additionally, nuclear power plants do not produce direct carbon emissions, making them a low-carbon energy source that supports global efforts to reduce greenhouse gas emissions. With increasing focus on climate change mitigation and decarbonization, nuclear power—driven by high-efficiency steam turbines—is positioned as a key solution for sustainable energy production.
As nuclear technology evolves, next-generation reactors, including small modular reactors (SMRs) and microreactors, are revolutionizing the way nuclear energy is deployed. These compact and scalable reactor designs integrate advanced steam turbine systems that can be installed in remote areas, industrial zones, and off-grid locations. Emerging innovations such as supercritical CO₂ turbines, direct Brayton cycle systems, and high-temperature reactor designs promise to increase efficiency, reduce costs, and enhance operational flexibility.
Looking forward, nuclear steam turbines will continue to be a vital part of global energy infrastructure, ensuring a steady supply of clean, reliable power. With advancements in turbine design, reactor efficiency, and grid integration, nuclear energy will remain a cornerstone of sustainable electricity generation, supporting the world’s transition to a low-carbon future.
Steam Turbines Power Grids Using Nuclear Energy
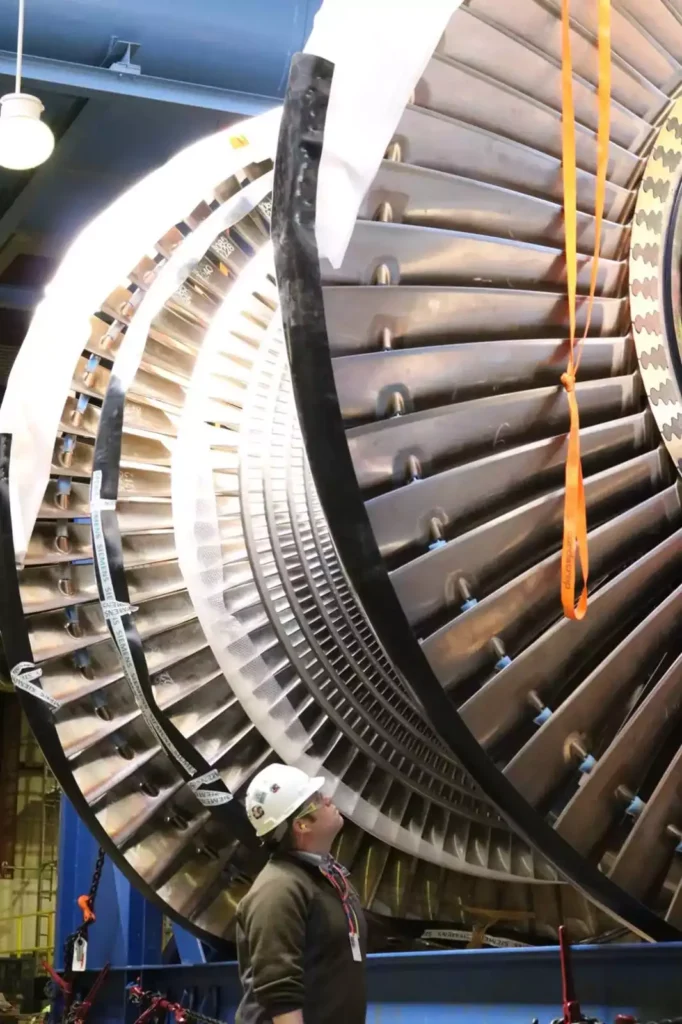
Steam turbines serve as the driving force behind nuclear power generation, enabling the transformation of nuclear heat into usable electricity that powers modern electrical grids. At the core of this process is nuclear fission, where atoms of uranium-235 or plutonium-239 split within a reactor core, releasing an immense amount of heat. This thermal energy is used to convert water into high-pressure steam, which is then channeled into a steam turbine system to generate mechanical motion.
The steam expands through multiple turbine stages, typically consisting of high-pressure, intermediate-pressure, and low-pressure sections. As the steam passes through each stage, it imparts kinetic energy to the turbine blades, causing them to rotate at high speeds. The turbine is mechanically connected to an electrical generator, where the rotational energy is converted into electrical power through electromagnetic induction. This electricity is then fed into the power grid, ensuring a continuous supply of energy for homes, industries, and businesses.
Once the steam has passed through the turbine, it enters a condenser, where it is cooled down and returned to liquid form. This process, often aided by cooling towers or water bodies, allows the closed-loop system to efficiently recycle water back into the reactor, maintaining consistent power generation while minimizing water consumption.
A key advantage of nuclear-powered steam turbines is their ability to provide stable baseload electricity. Unlike solar or wind power, which are dependent on weather conditions, nuclear plants operate continuously, ensuring a reliable and predictable energy output. This makes nuclear power an indispensable part of modern energy infrastructure, particularly in regions that require high-capacity, long-term power generation.
Beyond large nuclear power plants, advancements in nuclear technology are leading to the development of small modular reactors (SMRs) and microreactors, which integrate compact and high-efficiency steam turbines. These next-generation reactors are designed to be scalable and flexible, allowing for deployment in remote locations, industrial sites, and microgrids. Emerging technologies such as supercritical CO₂ turbines and advanced Brayton cycle systems promise to further enhance the efficiency and sustainability of nuclear power plants.
As global energy demand increases and the need for low-carbon power sources becomes more urgent, nuclear steam turbines will continue to play a critical role in ensuring a stable, clean, and resilient power grid. With ongoing advancements in reactor design, turbine efficiency, and grid integration, nuclear energy remains a key pillar of the world’s transition to a sustainable energy future.
Steam Turbines Power Grids Using Nuclear Energy
Steam turbines are at the heart of nuclear power plants, converting nuclear heat into mechanical energy and ultimately into electricity that drives modern power grids. The process begins inside the reactor core, where nuclear fission releases an immense amount of heat by splitting uranium or plutonium atoms. This heat energy is transferred to a coolant—typically pressurized water or molten salt, depending on the reactor type—which then generates high-pressure steam.
This steam is then directed into a steam turbine system, where it undergoes controlled expansion through a series of blades and stages. The steam’s force causes the turbine blades to spin rapidly, converting the thermal energy into mechanical energy. The turbine is directly connected to a generator, where this mechanical motion is transformed into electricity through electromagnetic induction. This high-voltage electricity is then sent to the grid, supplying industries, businesses, and households with continuous power.
To maximize efficiency, nuclear power plants utilize multi-stage steam turbines, which consist of high-pressure, intermediate-pressure, and low-pressure sections. These different sections allow the turbine to extract the maximum amount of energy from the steam, ensuring optimal performance and extended turbine lifespan. After passing through the turbine, the steam enters a condenser, where it is cooled and converted back into liquid water. This water is then pumped back into the system, completing a closed-loop cycle that ensures efficient water usage and minimal thermal losses.
One of the most significant advantages of nuclear-powered steam turbines is their ability to provide constant and large-scale electricity generation. Unlike intermittent renewable energy sources such as solar and wind, which depend on weather conditions, nuclear plants operate continuously, delivering a stable and predictable power supply. This makes nuclear energy a cornerstone of modern energy grids, especially in regions that require high-capacity, long-term power solutions.
As nuclear technology advances, the next generation of reactors, including small modular reactors (SMRs) and microreactors, are integrating high-efficiency steam turbines into more compact and scalable systems. These smaller and more flexible reactors enable nuclear power to be deployed in remote areas, industrial zones, and microgrid networks, supporting decentralized energy solutions. Additionally, emerging innovations such as supercritical CO₂ turbines and advanced Brayton cycle designs are enhancing steam turbine efficiency, leading to greater energy output and reduced operational costs.
With global energy demand continuing to rise and the push for low-carbon electricity sources becoming more urgent, nuclear-powered steam turbines will remain a key player in the energy transition. By combining proven reliability with cutting-edge advancements, steam turbines will continue to support the growth of nuclear power as a sustainable, scalable, and carbon-free energy source, ensuring a stable and resilient power grid for the future.
Steam Turbines Power Grids Using Nuclear Energy
Steam turbines remain the cornerstone of nuclear power generation, converting thermal energy from nuclear fission into mechanical motion and ultimately electrical power for the grid. This process begins in the reactor core, where nuclear fuel—typically uranium-235 or plutonium-239—undergoes controlled fission reactions, releasing massive amounts of heat. This heat energy is transferred to pressurized water or other coolant mediums, which then produce high-pressure steam used to drive turbines.
The steam turbine system is composed of multiple stages, designed to extract maximum energy from steam expansion. In a high-pressure turbine, the steam enters at extremely high temperatures and pressures, where it rapidly expands and forces the turbine blades to spin. This rotation converts thermal energy into mechanical work. The partially expanded steam is then reheated and passed through intermediate-pressure and low-pressure turbines, ensuring greater efficiency and power output before entering the generator.
In the generator, the mechanical rotation of the turbine shaft drives an electromagnetic field, inducing an electric current that is then fed into the power grid. This process allows nuclear power plants to continuously supply electricity at a stable and predictable rate, making them a vital component of baseload power generation. Unlike fossil fuel plants, nuclear reactors do not require constant fuel combustion, and unlike renewable sources such as wind and solar, they are not affected by weather conditions, ensuring a steady and uninterrupted flow of energy.
After the steam passes through the turbines, it enters a condenser, where it is rapidly cooled using water from cooling towers, lakes, or seawater systems. This cooling process converts steam back into liquid water, which is then pumped back into the reactor system, completing a closed-loop cycle that optimizes water usage and maintains efficiency. Advanced designs incorporate heat recovery systems, improving overall plant performance and reducing thermal pollution.
As energy demand rises and the need for clean, low-carbon electricity increases, nuclear power is evolving with next-generation technologies. Small modular reactors (SMRs) and microreactors integrate high-efficiency steam turbines into compact and scalable designs, making nuclear power more accessible and flexible for a wide range of applications. These reactors are particularly useful for remote locations, industrial plants, and military bases, offering on-site, stable power generation with minimal environmental impact.
Further advancements in supercritical CO₂ turbines, Brayton cycle designs, and direct energy conversion systems are pushing steam turbine efficiency even higher. These technologies enable higher power densities, improved thermodynamic efficiency, and reduced operational costs, making nuclear energy even more competitive in the global market.
With the global shift towards sustainable energy solutions, nuclear-powered steam turbines will continue to play a crucial role in ensuring grid stability, energy security, and decarbonization efforts. As innovation drives greater efficiency and flexibility, nuclear energy remains a key pillar of the world’s clean energy future, providing reliable, large-scale, and long-term power generation for decades to come.
Steam Turbines Power Grids Using Nuclear Energy
Steam turbines serve as the critical link between nuclear fission and the electricity that powers homes, businesses, and industries. Their role in nuclear power plants is to convert heat energy into mechanical energy and then into electrical energy, ensuring a steady supply of power to the grid. This process begins in the reactor core, where nuclear fission reactions generate immense heat, raising the temperature of a coolant—typically water, molten salt, or gas, depending on the reactor type. This heat is then used to produce high-pressure steam, which is directed into a steam turbine system to drive power generation.
Once inside the high-pressure section of the turbine, the steam rapidly expands, forcing turbine blades to spin at high speeds. This rotational energy is transferred to a generator, where electromagnetic induction creates an electric current. The partially expanded steam is then reheated—either by residual reactor heat or separate reheater systems—and continues its journey through intermediate-pressure and low-pressure turbines, further enhancing energy extraction. This multi-stage expansion process ensures maximum efficiency and power output, a necessity for large-scale electricity production.
After passing through the turbines, the steam moves to a condenser, where it is rapidly cooled using a heat exchanger system. Water from cooling towers, rivers, or seawater sources absorbs excess heat, causing the steam to condense back into liquid form. This water is then pumped back into the system for reuse in the reactor, maintaining a closed-loop cycle that optimizes efficiency and water conservation. Advanced nuclear plants incorporate waste heat recovery systems to further improve thermal efficiency and reduce heat losses to the environment.
Nuclear-powered steam turbines offer a major advantage over other power sources by providing constant, large-scale baseload electricity. Unlike fossil fuel plants, they do not rely on continuous combustion, reducing fuel dependency and greenhouse gas emissions. Moreover, unlike wind or solar power, which are weather-dependent, nuclear power remains unaffected by climate variations, ensuring a stable and uninterrupted energy supply. This makes nuclear energy an essential part of modern power grids, balancing demand fluctuations and supporting critical infrastructure.
As nuclear technology advances, the next generation of reactors is improving steam turbine efficiency and adaptability. Small modular reactors (SMRs) and microreactors are integrating compact, high-performance steam turbines to deliver flexible, scalable power solutions. These reactors can be deployed in remote locations, industrial plants, and military installations, providing on-site, decentralized power generation with minimal environmental impact.
New breakthroughs in supercritical CO₂ turbines, direct Brayton cycle systems, and high-temperature reactor designs are further enhancing energy conversion efficiency. These technologies allow higher thermal efficiencies, reduced mechanical losses, and lower operational costs, making nuclear energy even more competitive in an evolving energy market.
With growing global efforts toward clean energy adoption and carbon neutrality, nuclear-powered steam turbines will continue to play a pivotal role in energy security and sustainability. As engineering innovations drive higher efficiency and reliability, nuclear energy will remain a cornerstone of future power grids, ensuring a dependable and environmentally responsible electricity supply for generations to come.
Nuclear Reactors Generate Steam for Turbines
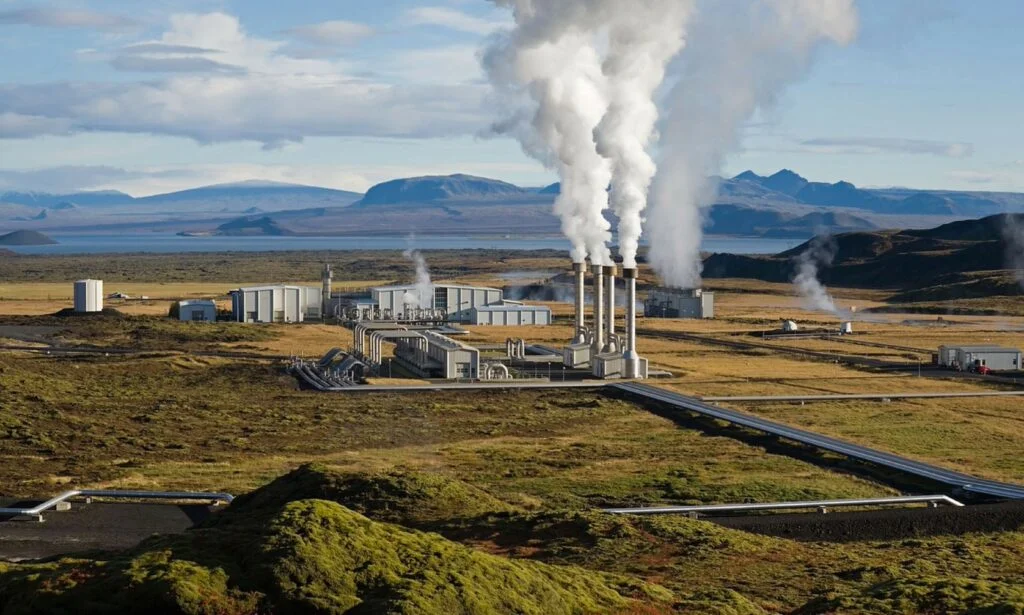
Nuclear reactors serve as the primary heat source in nuclear power plants, generating the high-pressure steam necessary to drive steam turbines and produce electricity. At the core of this process is nuclear fission, where atoms of uranium-235 or plutonium-239 split, releasing a tremendous amount of heat. This heat is transferred to a coolant medium, typically water, liquid metal, or gas, depending on the reactor type.
In most commercial nuclear power plants, pressurized water reactors (PWRs) and boiling water reactors (BWRs) dominate the industry. In a PWR, water is kept under high pressure to prevent boiling and is circulated through a heat exchanger, where it transfers heat to a secondary water loop, producing steam. In a BWR, water is allowed to boil directly within the reactor core, generating steam that is sent straight to the turbines. This steam, now carrying the thermal energy from nuclear fission, expands through the turbine system, converting heat energy into mechanical energy by rotating the turbine blades.
As the turbine spins, it drives a generator, where the rotational motion is transformed into electricity through electromagnetic induction. This high-voltage electricity is then transmitted to the power grid, supplying energy for residential, industrial, and commercial use. After passing through the turbine, the steam enters a condenser, where it is cooled back into water using cooling towers or natural water sources. The condensed water is then pumped back into the reactor system, ensuring an efficient and closed-loop cycle that minimizes water consumption.
Nuclear reactors provide a continuous and stable supply of steam for turbines, unlike fossil fuel power plants that require constant combustion or renewable sources that depend on weather conditions. This reliability makes nuclear energy a key component of baseload electricity generation, ensuring grid stability and energy security.
With advancements in nuclear technology, next-generation reactors such as small modular reactors (SMRs) and high-temperature gas-cooled reactors (HTGRs) are improving steam production efficiency and reactor safety. These modern designs integrate innovative cooling systems and advanced turbine configurations, allowing for more flexible and scalable nuclear power solutions. Emerging technologies, such as supercritical CO₂ turbines and closed Brayton cycle systems, are also enhancing thermal efficiency, making nuclear power even more competitive in the evolving energy landscape.
As the world transitions toward low-carbon energy solutions, nuclear power remains a vital contributor to sustainable electricity production. By efficiently generating steam to drive turbines, nuclear reactors will continue to play a crucial role in reducing carbon emissions and ensuring a stable and resilient power grid for the future.
Nuclear Reactors Generate Steam for Turbines
Nuclear power plants rely on nuclear fission to generate the heat necessary to produce steam, which in turn drives steam turbines to generate electricity. This process begins inside the reactor core, where the controlled splitting of uranium-235 or plutonium-239 atoms releases a massive amount of thermal energy. This heat is absorbed by a coolant, typically water, liquid metal, or gas, depending on the reactor design.
In pressurized water reactors (PWRs), the most common type of nuclear reactor, water is kept at extremely high pressure to prevent it from boiling inside the reactor vessel. Instead, the heated water is circulated through a steam generator, where it transfers heat to a secondary water loop, producing steam that is then sent to the turbines. In boiling water reactors (BWRs), the water inside the reactor core is allowed to boil directly, generating steam that travels straight to the turbine system. Other advanced reactors, such as high-temperature gas-cooled reactors (HTGRs) and molten salt reactors (MSRs), use helium or liquid salts as coolants, operating at even higher efficiencies.
Once generated, the high-pressure steam expands as it moves through a series of turbine stages—starting with high-pressure turbines, followed by intermediate- and low-pressure turbines. The force of the steam causes the turbine blades to spin at high speeds, converting the thermal energy into mechanical energy. This mechanical energy is then used to rotate a generator, where electromagnetic induction produces electricity that is fed into the power grid.
After the steam passes through the turbine, it enters a condenser, where it is cooled and condensed back into liquid water using cooling towers or natural water sources such as rivers, lakes, or seawater. The cooled water is then pumped back into the reactor system, creating a closed-loop cycle that minimizes waste and maximizes efficiency.
Nuclear-generated steam provides several advantages over fossil fuel plants and renewable energy sources. Unlike coal or natural gas plants, nuclear reactors do not burn fuel, making them a low-carbon energy source with no greenhouse gas emissions during operation. Unlike wind and solar, nuclear power provides constant and reliable energy, unaffected by weather conditions or daylight availability. This makes nuclear power one of the most stable and scalable sources of electricity for modern power grids.
The next generation of nuclear reactors, including small modular reactors (SMRs) and microreactors, are improving steam generation efficiency with enhanced safety features and advanced turbine systems. These reactors are designed to be compact, scalable, and deployable in remote areas, providing decentralized, off-grid power solutions for industrial operations, military bases, and disaster recovery sites.
New technologies such as supercritical CO₂ turbines and direct Brayton cycle systems are also emerging to improve the efficiency of energy conversion, enabling nuclear power plants to generate more electricity from the same amount of heat. These advancements will help nuclear energy remain a key player in the global shift toward clean and sustainable power generation, ensuring a reliable and resilient energy future.
Nuclear Reactors Generate Steam for Turbines
Nuclear reactors play a fundamental role in producing steam for turbines, enabling the generation of large-scale, continuous electricity. At the heart of this process is nuclear fission, where atoms of uranium-235 or plutonium-239 split, releasing enormous amounts of heat. This heat is used to raise the temperature of a coolant, typically water, helium, liquid sodium, or molten salt, depending on the reactor type. The heated coolant then facilitates the production of high-pressure steam, which is directed into a steam turbine system to generate electricity.
In a pressurized water reactor (PWR), the most widely used reactor type, the coolant water is kept under extremely high pressure to prevent boiling. This hot water transfers heat through a steam generator, where a separate water loop is converted into steam. In a boiling water reactor (BWR), the reactor directly generates steam in the core, which is then sent straight to the turbine. Other advanced reactors, such as high-temperature gas-cooled reactors (HTGRs) and fast breeder reactors (FBRs), operate at even higher temperatures and efficiencies, using gases or liquid metals as coolants to generate steam more effectively.
Once steam is produced, it moves into the steam turbine system, where it expands through a series of turbine stages. It first enters the high-pressure turbine, where its rapid expansion forces turbine blades to spin at high speeds. This rotational motion is transferred along a shaft to a generator, where it is converted into electricity through electromagnetic induction. The steam then passes through intermediate- and low-pressure turbines, extracting additional energy before being condensed back into water in a condenser system.
The condenser plays a crucial role in ensuring an efficient, closed-loop operation. It rapidly cools the steam using external water sources—such as rivers, lakes, seawater, or cooling towers—allowing the steam to condense back into liquid water. This recirculated water is then pumped back into the system, ensuring minimal water loss and optimized thermal efficiency.
Nuclear power plants are known for their ability to provide stable, baseload electricity, unaffected by fuel supply fluctuations or weather conditions. Unlike coal and natural gas plants, nuclear reactors do not produce carbon dioxide emissions during operation, making them a key technology for reducing greenhouse gas emissions. Additionally, compared to wind and solar energy, which are intermittent and dependent on environmental conditions, nuclear power ensures a constant energy supply, supporting grid reliability and industrial demand.
As nuclear technology advances, small modular reactors (SMRs) and microreactors are emerging as a game-changing solution for distributed power generation. These reactors integrate high-efficiency steam turbines into compact, scalable designs, making them ideal for remote locations, military applications, and industrial sites. They offer enhanced safety, faster deployment, and lower operational costs, making nuclear power more adaptable to a wider range of applications.
Beyond traditional steam turbines, new developments in supercritical CO₂ power cycles, Brayton cycle turbines, and direct energy conversion systems are enhancing nuclear power plant thermal efficiency. These technologies enable higher power densities, reduced mechanical losses, and improved overall performance, positioning nuclear energy as an even more competitive and sustainable energy source.
As global energy demands increase and the need for low-carbon, stable power sources grows, nuclear reactors will remain essential for supplying high-efficiency steam to power turbines. With ongoing innovations and improvements in reactor and turbine design, nuclear energy continues to be a reliable, clean, and future-ready solution for the world’s electricity needs.
Nuclear Reactors Generate Steam for Turbines
Nuclear power plants rely on steam-driven turbines to convert nuclear heat into electricity, providing a steady and reliable source of power for modern grids. The key to this process is nuclear fission, in which the controlled splitting of uranium-235 or plutonium-239 nuclei releases immense amounts of thermal energy. This heat is used to raise the temperature of a coolant, typically water, gas, or liquid metal, which then facilitates the generation of high-pressure steam for turbines.
In pressurized water reactors (PWRs), the coolant water is maintained at extremely high pressure to prevent it from boiling within the reactor. Instead, the heat is transferred through a steam generator, which converts a secondary water loop into steam that then drives the turbines. In contrast, boiling water reactors (BWRs) allow water to boil directly within the reactor core, producing steam that is fed directly into the turbine system. Advanced reactors such as high-temperature gas-cooled reactors (HTGRs) and molten salt reactors (MSRs) utilize gases or liquid salts as coolants, enabling higher thermal efficiencies and improved safety features.
Once generated, the steam expands rapidly as it passes through the turbine system, which consists of multiple stages. It first enters a high-pressure turbine, where its thermal energy is converted into mechanical energy by spinning the turbine blades at high speeds. The steam then moves through intermediate- and low-pressure turbines, undergoing further expansion and energy extraction. This mechanical energy is transmitted to a generator, where electromagnetic induction creates electricity that is distributed to the power grid.
After passing through the turbines, the steam must be condensed back into water to maintain system efficiency. This process takes place in a condenser, where cooling systems—such as water from rivers, lakes, seawater, or cooling towers—remove excess heat, allowing the steam to condense into liquid form. The resulting water is then pumped back into the reactor loop, forming a continuous and efficient closed-cycle system.
Nuclear-generated steam provides several advantages over fossil fuel-based and renewable energy sources. Unlike coal or natural gas plants, nuclear reactors do not rely on continuous combustion, eliminating carbon dioxide emissions and reducing air pollution. Unlike wind and solar power, which are dependent on weather conditions, nuclear energy provides a stable and continuous supply of electricity, ensuring grid stability and reliability.
New advancements in small modular reactors (SMRs) and microreactors are enhancing steam generation efficiency and scalability. These reactors incorporate compact, high-performance steam turbines, making them ideal for off-grid applications, industrial power generation, and decentralized energy production. By offering faster deployment, improved safety features, and lower capital costs, SMRs and microreactors are reshaping the future of nuclear-powered steam generation.
Further improvements in turbine efficiency are being explored through supercritical CO₂ power cycles, closed Brayton cycle turbines, and next-generation heat exchangers. These technologies are increasing thermal conversion efficiency, reducing operational costs, and optimizing energy extraction from nuclear reactors.
As global energy demands continue to rise, nuclear power remains a key player in the transition toward low-carbon, high-efficiency electricity generation. By harnessing nuclear heat to generate high-quality steam for turbines, nuclear reactors will continue to play a vital role in ensuring sustainable and reliable energy production for decades to come.
Steam Turbines Convert Nuclear Heat into Power
Steam turbines play a crucial role in converting nuclear heat into usable electricity, enabling nuclear power plants to generate large-scale, stable, and carbon-free energy. This process begins within a nuclear reactor, where the controlled fission of uranium-235 or plutonium-239 atoms releases vast amounts of heat. This heat is transferred to a coolant, typically water, which is then used to produce high-pressure steam to drive turbines.
In pressurized water reactors (PWRs), the most common type of nuclear reactor, water is kept under high pressure to prevent it from boiling within the reactor vessel. Instead, the heated coolant transfers energy to a secondary water loop inside a steam generator, producing steam that is sent to the turbine system. In boiling water reactors (BWRs), the water inside the reactor itself boils, generating steam directly that is fed into the turbines. Advanced reactor designs, such as high-temperature gas-cooled reactors (HTGRs) and molten salt reactors (MSRs), use alternative coolants like helium or liquid salts to achieve even higher efficiencies and safety levels.
Once the steam is produced, it is directed into a steam turbine, where it expands through a series of rotating blades, converting its thermal energy into mechanical energy. The turbine is divided into multiple stages, starting with a high-pressure turbine, followed by intermediate- and low-pressure turbines. The rotational force of the turbine shaft is transferred to an electrical generator, where it is transformed into usable electricity through electromagnetic induction.
After passing through the turbines, the steam is cooled and condensed back into water inside a condenser. Cooling water from natural sources like rivers, lakes, seawater, or cooling towers removes excess heat, allowing the steam to condense and be recirculated back into the system. This creates an efficient closed-loop cycle, ensuring minimal water loss and optimized thermal efficiency.
Nuclear power provides several key advantages over other energy sources. Unlike fossil fuel plants, nuclear reactors do not rely on combustion, producing no carbon emissions during operation. Unlike intermittent renewables such as wind and solar, nuclear power delivers continuous, reliable baseload electricity, making it essential for maintaining grid stability.
The latest advancements in small modular reactors (SMRs) and microreactors are further improving steam turbine efficiency and expanding nuclear energy’s applications. These compact, scalable reactor designs integrate high-performance turbines and can be deployed in remote locations, industrial settings, and off-grid environments, offering clean, decentralized power generation.
Beyond conventional steam turbines, emerging technologies like supercritical CO₂ turbines and closed Brayton cycle systems are enhancing energy conversion efficiency, allowing nuclear power plants to extract more electricity from the same amount of heat. These innovations will help nuclear energy remain a key pillar of sustainable power generation, ensuring a stable, low-carbon energy future.
Steam Turbines Convert Nuclear Heat into Power
Steam turbines are the critical link between nuclear reactors and electricity generation, transforming nuclear heat into mechanical energy and ultimately into usable electrical power. At the core of this process is nuclear fission, where atoms of uranium-235 or plutonium-239 split, releasing enormous amounts of energy in the form of heat. This heat is transferred to a coolant, typically water, which is then used to produce high-pressure steam that drives the turbine system.
In pressurized water reactors (PWRs), which dominate the global nuclear industry, water is kept under high pressure to prevent boiling inside the reactor vessel. Instead, the coolant transfers its heat to a separate water loop in a steam generator, where it converts water into steam. Boiling water reactors (BWRs), on the other hand, allow water to boil directly in the reactor core, sending steam directly to the turbines. Advanced reactor technologies, such as high-temperature gas-cooled reactors (HTGRs) and molten salt reactors (MSRs), use alternative coolants like helium or liquid salts, which allow for higher operating temperatures and improved thermal efficiency.
Once the steam is produced, it is directed into a steam turbine, where it undergoes rapid expansion, forcing turbine blades to rotate at high speeds. This mechanical energy is transferred through a rotating shaft to an electrical generator, where it is converted into electricity through electromagnetic induction. The turbine system is designed with multiple stages, including high-pressure, intermediate-pressure, and low-pressure turbines, to extract as much energy as possible from the steam before it is cooled and condensed.
After passing through the turbines, the steam enters a condenser, where it is cooled using water from natural sources such as rivers, lakes, seawater, or cooling towers. The condensed steam is then pumped back into the reactor system, forming a closed-loop cycle that ensures maximum efficiency and minimal water loss.
Compared to fossil fuel power plants, nuclear steam turbines operate without carbon emissions, making them a crucial component of low-carbon energy production. Unlike wind and solar power, which depend on weather conditions, nuclear energy provides a stable and continuous electricity supply, ensuring grid reliability and energy security.
Innovations in small modular reactors (SMRs) and microreactors are advancing steam turbine technology, allowing for more efficient and flexible power generation. These compact, scalable reactors integrate advanced turbine systems that can be deployed in remote locations, industrial facilities, and off-grid communities, providing reliable and clean electricity where large power plants are not feasible.
Further developments in supercritical CO₂ turbines, closed Brayton cycle systems, and direct energy conversion technologies are pushing the boundaries of nuclear power efficiency. These emerging systems promise to increase power output, reduce mechanical losses, and improve overall plant performance, making nuclear energy an even more competitive and sustainable option for global electricity needs.
As the demand for low-carbon, high-efficiency power grows, steam turbines will remain a vital component of nuclear energy, ensuring reliable, large-scale electricity generation while supporting the transition to a more sustainable energy future.
Steam Turbines Convert Nuclear Heat into Power
Steam turbines remain the core technology that enables nuclear reactors to generate usable electricity. The process starts inside a nuclear reactor, where fission reactions release thermal energy by splitting uranium-235 or plutonium-239 atoms. This intense heat is absorbed by a coolant, typically water, which then carries the thermal energy to a steam generation system. The high-pressure steam produced in this step is the driving force behind the steam turbine, which in turn powers an electric generator.
In a pressurized water reactor (PWR), which is the most widely used reactor type, water is kept under high pressure to prevent it from boiling inside the reactor core. Instead, it transfers heat to a secondary loop in a steam generator, where water is converted into high-pressure steam. This steam then flows into the turbine system. In contrast, a boiling water reactor (BWR) generates steam directly within the reactor vessel, eliminating the need for a secondary loop and sending steam directly to the turbine. Advanced reactor technologies, such as gas-cooled and molten salt reactors, employ alternative coolants that operate at higher temperatures, improving thermal efficiency and increasing power output.
The steam turbine system consists of multiple stages, designed to extract the maximum amount of energy from the steam. First, the steam enters a high-pressure turbine, where it expands and transfers its energy to rotating blades. The partially expanded steam is then reheated or directed to intermediate- and low-pressure turbines, where additional energy extraction occurs. The rotational motion of the turbine blades drives a generator, where electromagnetic induction converts the mechanical energy into electricity that can be delivered to the grid.
After passing through the turbines, the steam must be cooled and condensed back into liquid form to maintain system efficiency. This process occurs in a condenser, where cooling water from an external source—such as a river, lake, seawater, or cooling towers—removes residual heat. The condensed water is then recirculated back into the reactor, ensuring a closed-loop, efficient operation with minimal water loss.
One of the key advantages of nuclear-powered steam turbines is their ability to provide stable, baseload electricity without emitting carbon dioxide or air pollutants. Unlike fossil fuel plants, which burn coal or gas, nuclear reactors do not rely on combustion, making them a clean energy source that supports global climate change mitigation efforts. Unlike solar and wind energy, which depend on weather conditions, nuclear energy delivers continuous, predictable power, ensuring grid stability and reliability.
Advancements in small modular reactors (SMRs) and microreactors are enhancing the efficiency and versatility of steam turbines. These compact reactors integrate advanced turbine technology, allowing for distributed power generation in remote locations, industrial sites, and off-grid communities. The deployment of smaller, factory-built nuclear units reduces construction times and costs while maintaining high safety and performance standards.
Emerging technologies such as supercritical CO₂ turbines, direct Brayton cycle systems, and next-generation heat exchangers are set to further optimize nuclear power efficiency. These innovations can achieve higher thermal conversion rates, minimize mechanical losses, and enhance overall plant performance, making nuclear energy even more competitive in the global energy landscape.
As the world transitions toward low-carbon, sustainable energy solutions, steam turbines will remain at the heart of nuclear power generation, ensuring a reliable and scalable electricity supply for decades to come.
Steam Turbines Convert Nuclear Heat into Power
Steam turbines remain essential to the operation of nuclear power plants, transforming nuclear-generated heat into mechanical energy, which is then converted into electricity. This process begins in a nuclear reactor, where controlled fission reactions release large amounts of heat as uranium-235 or plutonium-239 atoms split. This heat is absorbed by a coolant, typically water, which carries the thermal energy to a steam generator. The produced high-pressure steam then flows into the turbine system, where it is used to drive a series of rotating blades, producing the mechanical force needed to generate electricity.
In pressurized water reactors (PWRs), water is maintained under high pressure to prevent boiling, transferring heat to a secondary loop where steam is generated. In contrast, boiling water reactors (BWRs) allow water in the reactor vessel to boil, producing steam directly to power the turbine. Advanced reactors, such as high-temperature gas-cooled reactors (HTGRs) and molten salt reactors (MSRs), use alternative coolants like helium or liquid salts, enabling higher operating temperatures and improved thermal efficiency.
Once the steam is produced, it enters the steam turbine system, where it undergoes expansion. The high-pressure steam first passes through a high-pressure turbine, transferring energy to its rotating blades. The partially expanded steam is then directed to intermediate- and low-pressure turbines, where further energy extraction occurs. This multi-stage process ensures maximum efficiency in energy conversion. The rotational motion of the turbine shaft drives a generator, where the mechanical energy is converted into electrical power through electromagnetic induction.
After leaving the turbines, the steam is cooled and condensed into liquid form in a condenser, which typically uses cooling water from external sources such as rivers, lakes, seawater, or cooling towers. This condensed water is then recirculated back into the reactor system, creating an efficient closed-loop cycle that minimizes water loss and enhances overall performance.
Compared to fossil fuel-based power plants, nuclear reactors provide a carbon-free, low-emission energy source that contributes to global efforts to combat climate change. Unlike solar and wind power, which are subject to fluctuations based on weather conditions, nuclear energy provides a stable and continuous electricity supply, ensuring grid reliability and energy security.
The evolution of nuclear technology, particularly with the development of small modular reactors (SMRs) and microreactors, is making nuclear-powered steam turbines more efficient and adaptable. These compact reactor designs allow for decentralized power generation, supporting applications in remote locations, industrial operations, and off-grid communities. The ability to deploy factory-assembled, scalable nuclear units reduces costs, shortens construction timelines, and enhances safety.
Looking ahead, innovations in advanced turbine systems, including supercritical CO₂ turbines, closed Brayton cycle engines, and next-generation heat exchangers, are set to boost efficiency, increase power output, and reduce operational losses. These emerging technologies will allow nuclear power plants to extract more energy from the same amount of heat, further enhancing the role of nuclear energy in the global energy mix.
As the world shifts toward low-carbon, reliable power solutions, steam turbines will continue to be a critical component of nuclear energy, ensuring a stable and sustainable electricity supply while supporting the transition to a clean energy future.
Nuclear Power Drives Steam Turbines for Electricity
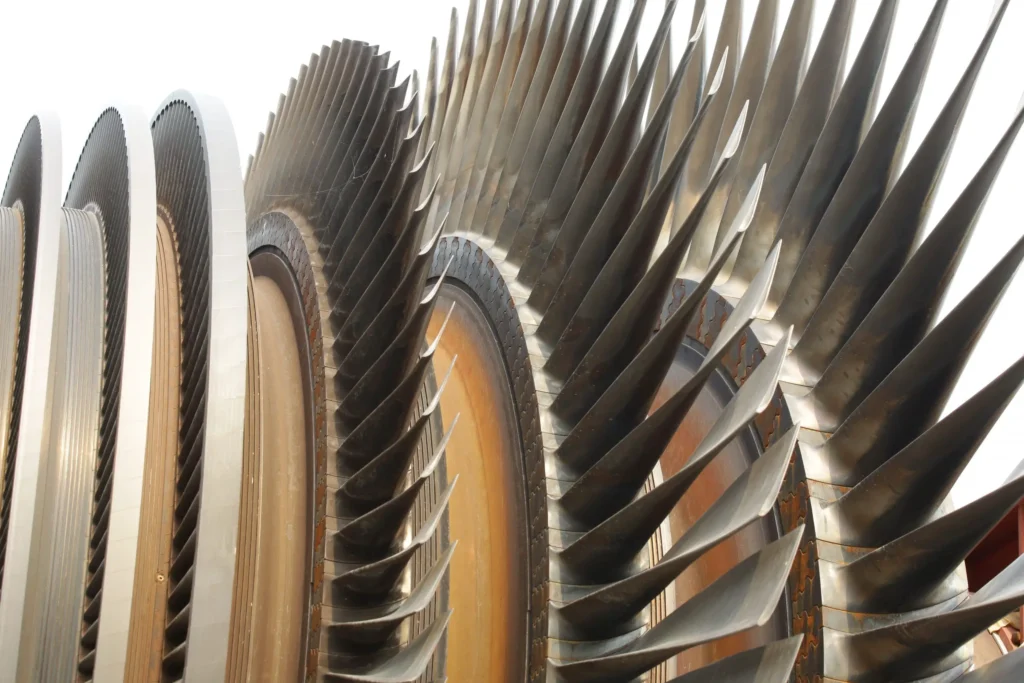
Nuclear power plants generate electricity by harnessing the heat produced from nuclear fission to create high-pressure steam, which drives steam turbines connected to electric generators. This process provides a steady, reliable, and low-carbon source of energy, making nuclear power a crucial component of the global energy mix.
At the core of a nuclear power plant is the reactor, where uranium-235 or plutonium-239 atoms split, releasing an immense amount of heat. This thermal energy is absorbed by a coolant, typically water, liquid metal, or molten salt, depending on the reactor type. In pressurized water reactors (PWRs), water remains under high pressure to prevent boiling, transferring heat to a secondary loop where it turns water into steam. In boiling water reactors (BWRs), the reactor water boils directly, producing steam that is fed into the turbines.
The steam turbine system is the key mechanism that converts thermal energy into mechanical motion. As high-pressure steam enters the turbine, it expands and pushes against a series of rotating blades, causing the turbine shaft to spin at high speeds. The turbine is designed in multiple stages, including high-pressure, intermediate-pressure, and low-pressure sections, to extract the maximum possible energy from the steam. The rotating shaft is directly connected to a generator, where the mechanical motion is converted into electrical power through electromagnetic induction.
After passing through the turbines, the steam must be condensed back into liquid form to maintain the system’s efficiency. This occurs in a condenser, where cooling water from rivers, lakes, seawater, or cooling towers absorbs excess heat. The condensed water is then pumped back into the reactor system, forming a closed-loop cycle that ensures continuous and efficient power generation.
Unlike fossil fuel power plants, which burn coal or natural gas, nuclear power produces zero carbon emissions during operation, making it a crucial technology for reducing greenhouse gas emissions and combating climate change. Unlike solar and wind energy, which depend on environmental conditions, nuclear power provides a stable, around-the-clock electricity supply, ensuring grid reliability and energy security.
As nuclear technology advances, small modular reactors (SMRs) and microreactors are emerging as next-generation solutions that enhance the flexibility and efficiency of steam turbines. These compact reactors can be deployed in remote locations, industrial sites, and off-grid communities, offering decentralized, scalable power solutions. Innovations in supercritical CO₂ turbines and advanced heat exchangers are further improving efficiency, enabling nuclear plants to extract more energy from the same amount of fuel.
With the growing demand for clean, reliable energy, steam turbines powered by nuclear reactors will continue to play a central role in global electricity generation, ensuring a sustainable energy future while meeting the world’s increasing power needs.
Nuclear Power Drives Steam Turbines for Electricity
Nuclear power plants operate by converting heat from nuclear fission into mechanical energy through steam turbines, which in turn generate electricity. This process is highly efficient, stable, and carbon-free, making nuclear energy a key contributor to global power generation.
At the heart of a nuclear power plant is the reactor core, where uranium-235 or plutonium-239 atoms undergo fission, releasing an immense amount of thermal energy. This heat is absorbed by a coolant, typically water, helium, liquid metal, or molten salt, depending on the reactor type. In the most common designs—pressurized water reactors (PWRs) and boiling water reactors (BWRs)—water is used to transfer this heat to a steam generator. In a PWR, water is kept under high pressure to prevent boiling, with heat transferred to a secondary loop where steam is produced. In a BWR, the water inside the reactor core boils directly, generating steam that is sent to the turbines.
The steam turbine is the central component in converting thermal energy into electricity. As high-pressure steam flows into the turbine, it expands and pushes against a series of precisely engineered rotating blades, causing the turbine shaft to spin. To maximize efficiency, nuclear steam turbines operate in multiple stages, beginning with high-pressure turbines, followed by intermediate- and low-pressure turbines that extract additional energy from the steam. This rotating shaft is connected to a generator, where electromagnetic induction converts the mechanical motion into usable electricity that is fed into the power grid.
After passing through the turbine system, the steam must be cooled and condensed back into liquid form for reuse. This occurs in a condenser, where cooling water from an external source—such as a river, lake, seawater, or cooling tower system—absorbs the remaining heat and facilitates condensation. The condensed water is then recirculated back into the reactor system, ensuring a closed-loop process that optimizes water usage and plant efficiency.
One of the key advantages of nuclear-powered steam turbines is their ability to provide continuous baseload electricity without producing greenhouse gases. Unlike fossil fuel power plants that burn coal or natural gas, nuclear reactors generate power without combustion, making them a clean energy solution for reducing carbon emissions. In contrast to solar and wind energy, which fluctuate based on weather conditions, nuclear power delivers consistent and predictable electricity, ensuring grid stability and energy security.
The evolution of nuclear technology is further enhancing the efficiency of steam turbines. Small modular reactors (SMRs) and microreactors represent a major innovation, enabling decentralized and scalable nuclear power generation. These compact reactors can be deployed in remote locations, industrial sites, and even military bases, reducing reliance on traditional large-scale power plants. Advanced developments, such as supercritical CO₂ turbines, direct Brayton cycle systems, and next-generation heat exchangers, are further improving the thermal efficiency of nuclear plants, allowing for higher energy output with reduced fuel consumption.
As global energy demand rises and nations seek low-carbon energy solutions, steam turbines powered by nuclear reactors will remain a crucial technology for meeting electricity needs. The ability to deliver clean, stable, and high-efficiency power makes nuclear energy an essential component of a sustainable and resilient energy future.
Nuclear Power Drives Steam Turbines for Electricity
Nuclear power plants rely on steam turbines to convert the thermal energy from nuclear fission into mechanical energy, which is then transformed into electricity. This process is a highly efficient and stable method of power generation, ensuring a continuous supply of clean energy without greenhouse gas emissions.
At the core of a nuclear power plant, uranium-235 or plutonium-239 atoms undergo fission, releasing intense heat. This heat is transferred to a coolant—typically water, helium, liquid metal, or molten salt—depending on the reactor design. In pressurized water reactors (PWRs), heat is transferred to a secondary loop where steam is produced, while in boiling water reactors (BWRs), the reactor water itself boils to generate steam directly. Other advanced reactor types, such as high-temperature gas-cooled reactors (HTGRs) and sodium-cooled fast reactors (SFRs), use alternative coolants that enable higher temperatures and increased efficiency.
The steam turbine system is the key component in converting this thermal energy into electricity. When high-pressure steam enters the turbine, it expands and pushes against the rotating blades, causing the turbine shaft to spin. This spinning motion is then used to drive an electric generator, where electromagnetic induction produces electricity. To maximize efficiency, modern steam turbines operate in multiple stages, including high-pressure, intermediate-pressure, and low-pressure turbines, extracting as much energy as possible from the steam.
Once the steam has passed through the turbine, it needs to be cooled and condensed back into liquid form for reuse. This occurs in a condenser, where an external cooling system—using water from a river, lake, ocean, or cooling towers—absorbs excess heat. The condensed water is then pumped back into the reactor system, forming a closed-loop cycle that optimizes resource use and enhances operational efficiency.
One of the biggest advantages of nuclear-powered steam turbines is their ability to deliver constant baseload power, unlike fossil fuel plants that rely on combustion or renewable sources like solar and wind, which depend on environmental conditions. Nuclear power provides grid stability and energy security, making it a reliable backbone of the electricity sector. Additionally, because nuclear plants do not burn fuel, they do not produce carbon emissions, making them a crucial component in global decarbonization efforts.
As nuclear technology advances, small modular reactors (SMRs) and microreactors are emerging as flexible and scalable solutions. These smaller reactors enable decentralized power generation, allowing for off-grid applications, industrial power needs, and remote community electrification. Innovations in supercritical CO₂ turbines, direct Brayton cycle systems, and next-generation steam cycles are further enhancing efficiency by enabling higher thermal conversion rates and reducing waste heat.
With the growing demand for clean, sustainable energy, nuclear power plants will continue to rely on steam turbines to generate electricity efficiently. The combination of high power density, long fuel cycles, and carbon-free operation ensures that nuclear energy remains a key player in the future energy landscape, supporting efforts to combat climate change and ensure global energy security.
Nuclear Power Drives Steam Turbines for Electricity
Nuclear power plants use steam turbines to convert thermal energy from nuclear fission into mechanical energy, which is then transformed into electricity. This process ensures a steady, reliable power supply while producing no direct carbon emissions, making nuclear energy a vital component of global energy systems.
At the core of a nuclear power plant, fission reactions in the reactor core generate extreme heat. This heat is transferred to a coolant, typically water, helium, liquid metal, or molten salt, depending on the reactor type. In pressurized water reactors (PWRs), this heat is transferred to a secondary loop, where it creates high-pressure steam. In boiling water reactors (BWRs), water boils directly inside the reactor vessel to produce steam. Advanced reactors, such as gas-cooled reactors and sodium-cooled fast reactors, operate at even higher temperatures, improving efficiency and enabling next-generation steam turbine designs.
The steam turbine system is responsible for transforming thermal energy into electricity. As the high-pressure steam enters the turbine, it expands and moves through a series of precision-engineered blades, causing the rotor to spin. To maximize energy extraction, nuclear steam turbines typically operate in multiple stages—starting with high-pressure turbines, followed by intermediate- and low-pressure turbines—before the steam is finally exhausted. This mechanical motion drives an electric generator, producing electricity that is then distributed to the power grid.
After the steam has passed through the turbines, it must be condensed back into liquid form to maintain system efficiency. This is achieved using a condenser, where a cooling medium—often water from a nearby river, ocean, or cooling tower system—absorbs excess heat. The condensed water is then recycled back into the system, ensuring a closed-loop process that minimizes water loss and optimizes power plant performance.
The advantage of nuclear-powered steam turbines lies in their ability to provide continuous, baseload electricity. Unlike fossil fuel plants that rely on combustion or renewable sources like wind and solar, which fluctuate based on environmental conditions, nuclear power ensures uninterrupted, large-scale electricity generation. Furthermore, because nuclear energy does not involve burning fuel, it does not produce carbon dioxide emissions, making it a key technology in efforts to combat climate change.
Advancements in nuclear technology are further enhancing the efficiency of steam turbines. Small modular reactors (SMRs) and microreactors are being developed to enable more flexible, scalable, and decentralized nuclear power generation. These reactors can be deployed in remote locations, industrial sites, and off-grid areas, providing reliable electricity where traditional large plants may not be feasible. Additionally, innovations such as supercritical CO₂ turbines, direct Brayton cycle systems, and next-generation steam cycles are pushing efficiency even further, allowing nuclear plants to extract more energy from the same amount of fuel while reducing overall operational costs.
As global energy demand continues to rise, and nations push for cleaner, more sustainable power solutions, nuclear steam turbine technology remains critical for ensuring long-term energy security and decarbonization. The combination of high power output, long fuel cycles, and carbon-free operation makes nuclear energy a cornerstone of the future electricity landscape, supporting a stable and resilient power grid worldwide.
EMS Power Machines
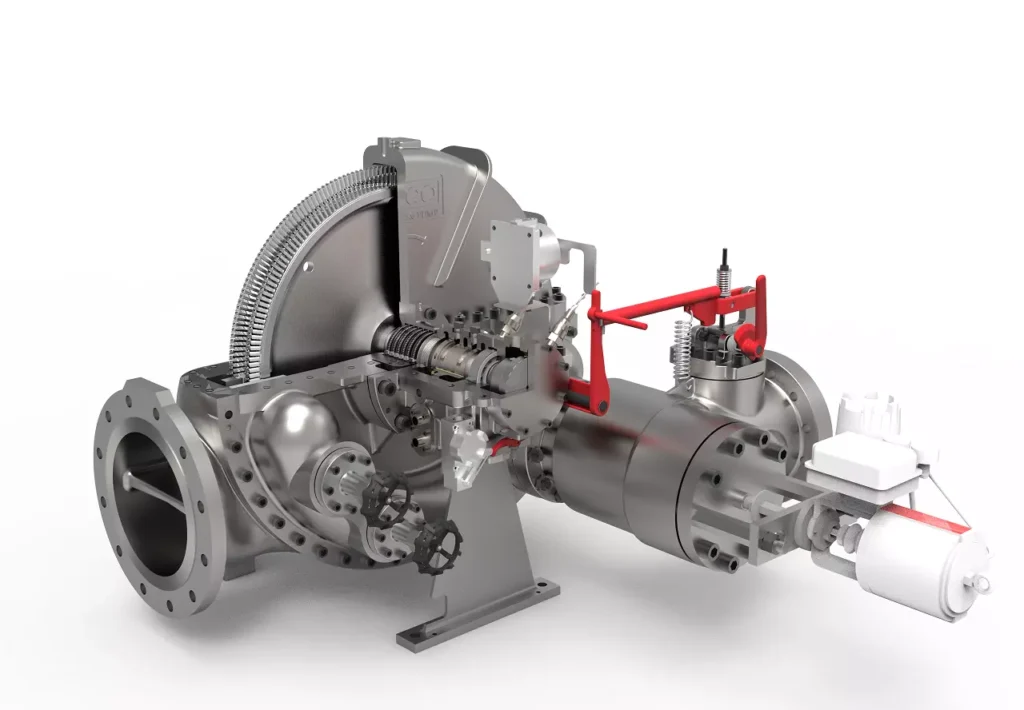
We design, manufacture and assembly Power Machines such as – diesel generators, electric motors, vibration motors, pumps, steam engines and steam turbines
EMS Power Machines is a global power engineering company, one of the five world leaders in the industry in terms of installed equipment. The companies included in the company have been operating in the energy market for more than 60 years.
EMS Power Machines manufactures steam turbines, gas turbines, hydroelectric turbines, generators, and other power equipment for thermal, nuclear, and hydroelectric power plants, as well as for various industries, transport, and marine energy.
EMS Power Machines is a major player in the global power industry, and its equipment is used in power plants all over the world. The company has a strong track record of innovation, and it is constantly developing new and improved technologies.
Here are some examples of Power Machines’ products and services:
- Steam turbines for thermal and nuclear power plants
- Gas turbines for combined cycle power plants and industrial applications
- Hydroelectric turbines for hydroelectric power plants
- Generators for all types of power plants
- Boilers for thermal power plants
- Condensers for thermal power plants
- Reheaters for thermal power plants
- Air preheaters for thermal power plants
- Feedwater pumps for thermal power plants
- Control systems for power plants
- Maintenance and repair services for power plants
EMS Power Machines is committed to providing its customers with high-quality products and services. The company has a strong reputation for reliability and innovation. Power Machines is a leading provider of power equipment and services, and it plays a vital role in the global power industry.
EMS Power Machines, which began in 1961 as a small factory of electric motors, has become a leading global supplier of electronic products for different segments. The search for excellence has resulted in the diversification of the business, adding to the electric motors products which provide from power generation to more efficient means of use.