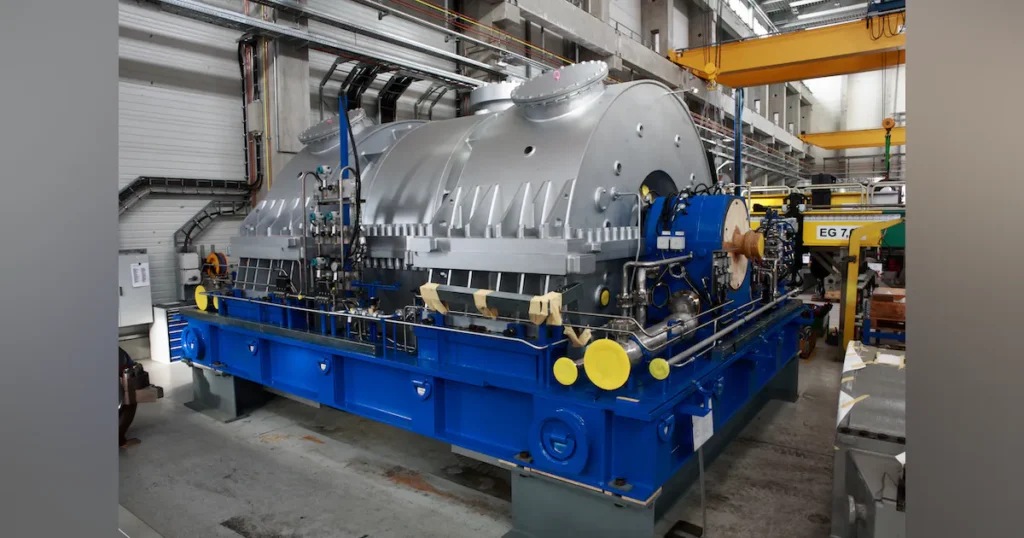
Turbine in Power Plant: In a power plant, a turbine is a key component used to convert energy from a high-pressure steam or gas flow into mechanical energy, which then drives an electric generator to produce electricity. Here’s a breakdown of how turbines operate within various types of power plants and the role they play in electricity generation:
1. Function and Mechanism of a Turbine
- Conversion of Energy: The turbine’s primary function is to convert thermal or kinetic energy into mechanical energy. In fossil fuel and nuclear power plants, steam generated from heating water passes through the turbine. In wind power, the kinetic energy of wind drives the turbine directly.
- Rotation: As the steam or gas flows through the turbine blades, it causes them to spin. This spinning motion is transferred to a shaft connected to the generator, which then produces electricity.
2. Types of Turbines in Power Plants
- Steam Turbines: Commonly found in coal, natural gas, and nuclear power plants, steam turbines use high-pressure steam to turn the blades. They are categorized into:
- Impulse Turbines: Steam jets strike the turbine blades directly.
- Reaction Turbines: Steam pressure changes as it passes through the blades.
- Gas Turbines: Utilized in natural gas power plants, they operate by burning natural gas to produce hot gases, which flow through the turbine to create rotation.
- Hydroelectric Turbines: In hydroelectric plants, water flows over turbine blades, converting the kinetic energy of flowing water into mechanical energy.
- Wind Turbines: Use the kinetic energy of the wind to rotate turbine blades, commonly seen in wind farms.
3. Components of a Turbine System
- Rotor and Shaft: The rotating component that drives the generator.
- Blades: These capture the steam, gas, or water flow, converting it into rotational energy.
- Nozzles: Direct the flow in impulse turbines.
- Casing: Encloses the turbine blades and directs the flow efficiently.
4. Efficiency and Optimization
- Heat Recovery: Modern turbines often include combined cycle systems, where waste heat from gas turbines is used to produce steam, driving an additional steam turbine and boosting overall efficiency.
- Cooling Systems: Turbines generate heat, so cooling mechanisms are essential to prevent overheating, which is often managed through cooling towers or condensers.
5. Environmental Impact and Innovations
- Fuel Efficiency: Advanced turbine designs and materials increase fuel efficiency, lowering emissions in fossil-fuel power plants.
- Renewable Adaptation: Innovations in wind and hydro turbines make renewable power generation more efficient and adaptable to various climates and locations.
6. Role in the Power Plant
- Turbines are the central machinery that converts thermal or kinetic energy into electricity. Their operation is fundamental in nearly every type of power plant, bridging the gap between fuel or resource energy and electrical output.
Turbines are critical for ensuring a reliable, efficient, and environmentally sound power generation process across various types of power plants.
1. Introduction to Turbines in Power Generation
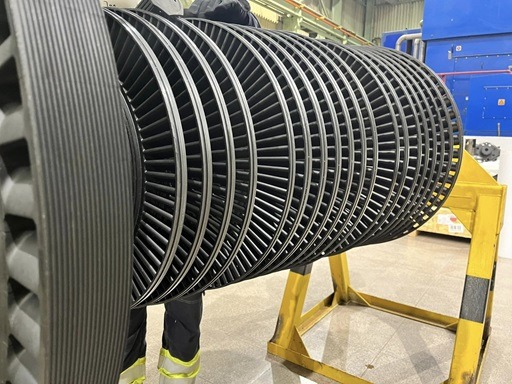
A turbine is a critical component in modern power plants, converting raw energy from fuels or natural resources into electricity. In both traditional and renewable energy systems, turbines play a central role by transforming thermal, kinetic, or hydraulic energy into mechanical energy that powers an electric generator. The technology surrounding turbine design has advanced significantly since its early applications in the 19th century, driven by the need for efficient and reliable electricity generation to meet growing demand.
Historically, the first widely used turbines appeared in steam engines, where steam pressure was harnessed to create movement. Over time, technological innovations allowed the development of various turbine types—such as steam, gas, hydroelectric, and wind turbines—each optimized for specific fuel sources and energy forms. Today, turbines are foundational in numerous types of power plants, from coal-fired and nuclear to wind farms and hydropower facilities.
The versatility of turbines is matched by their technical complexity. Each turbine type operates based on unique principles of thermodynamics and fluid dynamics, requiring intricate engineering to maximize efficiency. With rising environmental concerns, turbine technology is also adapting to minimize emissions and make better use of renewable resources. Advanced materials, high-precision manufacturing, and digital monitoring systems are now integral to turbine construction, helping power plants optimize performance and reduce environmental impact.
Understanding the mechanism and function of turbines offers insight into the intricacies of power generation and how turbines are engineered to maximize energy conversion. This in-depth exploration will detail the core principles, components, types, and operational dynamics of turbines within power plants.
2. Core Function of a Turbine
At its core, a turbine functions as an energy converter, transforming fluid-based energy—whether in the form of steam, gas, or water—into mechanical energy. This mechanical energy is then used to turn a generator, which produces electricity. The efficiency and reliability of turbines are paramount, as they directly affect the plant’s overall energy output, fuel consumption, and emissions.
Basic Principle of Energy Conversion
The core operating principle of turbines is based on energy transfer through fluid motion. In a typical power plant, energy from the combustion of fossil fuels, nuclear reactions, or natural sources like water flow or wind is transferred to a working fluid. For example, in a fossil fuel or nuclear power plant, water is heated to create high-pressure steam. This steam is directed toward the turbine blades, pushing them with enough force to create rotation. In hydroelectric plants, moving water flows directly over the turbine blades, and in wind power, wind energy propels the blades without an intermediary fluid.
The type of energy involved—thermal in steam turbines, kinetic in wind and hydroelectric turbines, and chemical in gas turbines—defines how the turbine must be engineered to maximize conversion. The mechanical rotation produced by this energy transfer is channeled through a rotating shaft to the generator, creating electricity.
Role in the Power Generation Cycle
Turbines are often seen as the “engine” of a power plant. They bridge the energy source, whether a fossil fuel, nuclear reactor, or renewable source, and the generator that produces electricity. The efficiency of turbines significantly impacts the amount of electricity generated per unit of fuel, making their design crucial for economic and environmental performance.
In steam and gas turbines, thermodynamic cycles (the Rankine cycle for steam and the Brayton cycle for gas) are used to maximize the conversion of fuel energy to rotational energy. These cycles establish a controlled sequence of heating, pressurizing, expanding, and exhausting the working fluid to maximize the energy transferred to the turbine blades. As the fluid flows through the turbine, it loses energy, creating movement that drives the generator.
3. Mechanism of Energy Conversion
The energy conversion mechanism in turbines depends on precise thermodynamic and fluid dynamic principles to capture, transfer, and utilize energy efficiently.
Key Phases of Energy Transfer
- Heat Absorption and Pressure Build-Up:
- In thermal power plants, fuel combustion or nuclear fission heats water, producing steam at high pressure. This steam expands as it flows into the turbine, pushing against the blades.
- For gas turbines, natural gas combustion produces high-pressure, high-temperature gases that expand through the turbine.
- In hydroelectric turbines, gravitational potential energy from falling or flowing water exerts force on turbine blades.
- Mechanical Rotation:
- As the working fluid (steam, gas, or water) moves through the turbine, it imparts energy to the blades, causing them to spin. In impulse turbines, the force directly strikes the blades, while in reaction turbines, the fluid’s pressure decreases as it flows, propelling the blades forward.
- This rotation is transmitted to the shaft connected to a generator. The shaft’s speed and the force applied depend on the energy input and turbine design, making each turbine type suited to specific fluid dynamics.
- Electrical Generation:
- The rotational motion of the turbine shaft is transferred to the generator, which uses an electromagnetic field to convert mechanical energy into electricity. The efficiency of this process largely depends on the consistency and strength of the rotational force supplied by the turbine.
Thermodynamic Principles in Turbines
Thermodynamics play a crucial role in turbine operation, guiding how energy is transferred from the fluid to the turbine blades. Two primary thermodynamic cycles govern turbine function:
- Rankine Cycle (Steam Turbines): In a steam turbine, water is boiled to produce high-pressure steam, which expands through the turbine, creating work. After passing through the turbine, the steam is condensed back to water and recycled, completing the cycle. This closed-loop system allows for repeated energy conversion with minimized waste.
- Brayton Cycle (Gas Turbines): In gas turbines, air is compressed, mixed with fuel, and ignited to produce high-pressure gas. This gas expands through the turbine, producing rotational force before being exhausted. This open-loop cycle is used mainly in natural gas power plants and jet engines.
The thermodynamic cycle choice impacts the turbine’s efficiency, fuel consumption, and environmental emissions, making it essential to select the right turbine type based on the fuel and plant requirements.
Fluid Dynamics in Turbine Operation
Fluid mechanics is fundamental to understanding turbine performance. Fluid velocity, pressure, and flow direction affect how efficiently a turbine converts energy. The fluid dynamics are controlled using components like nozzles, which guide the flow in impulse turbines, and blade designs, which maximize contact with the fluid for effective rotation. Efficient energy transfer is achieved through precision-engineered components that guide and optimize the fluid’s flow, balancing speed, and pressure as it moves through the turbine.
4. Components of a Turbine
Understanding a turbine’s function requires an in-depth look at its structural components. These parts work together to capture, transfer, and maintain the mechanical energy needed for electricity generation.
Key Components Explained
- Rotor: The rotor is the main rotating part of the turbine, comprising a shaft connected to the generator. It is subjected to high forces as it rotates at speeds often exceeding thousands of revolutions per minute (RPM). The rotor design is crucial for maintaining balance and efficient energy transfer.
- Blades:
- Blades are engineered to capture the fluid energy effectively. Their aerodynamic design varies by turbine type:
- Impulse Blades: These blades capture direct kinetic energy from high-speed fluid jets, ideal for turbines where high-velocity fluid strikes the blade surfaces directly.
- Reaction Blades: These blades rely on changes in fluid pressure and are often used in steam turbines where steam flows continuously over the blades.
- Blades must be crafted from materials that can withstand extreme temperatures, pressures, and corrosive environments, as in gas turbines where temperatures often exceed 1,000°C.
- Blades are engineered to capture the fluid energy effectively. Their aerodynamic design varies by turbine type:
- Shaft: The shaft connects the turbine rotor to the generator. It is usually a solid or hollow metal cylinder that transfers rotational energy, designed to resist bending, wear, and temperature-induced expansion.
- Nozzles: Nozzles play a crucial role in impulse turbines by directing the high-pressure steam or gas toward the blades at optimal angles. They help control the fluid’s velocity and distribution, ensuring consistent energy transfer.
- Casing and Housing:
- The casing or housing encloses the turbine’s internal components, maintaining pressure and directing fluid flow through the blades. It serves as a protective shell, managing pressure and preventing leaks or fluid losses.
Material Selection and Durability
Turbines operate under extreme conditions, and their materials must be chosen for strength, temperature resilience, and longevity. Advanced materials like superalloys—nickel-based alloys and titanium composites—are commonly used in high-temperature gas turbines. These materials resist thermal deformation, corrosion, and fatigue, extending the lifespan of turbine components and ensuring consistent operation.
Cooling and Lubrication
Due to high operating temperatures, turbines require effective cooling and lubrication systems:
- Cooling: Often achieved through air, water, or oil systems, cooling is essential in gas turbines, where high-temperature gases can damage components without proper temperature control.
- Lubrication: Bearings and shafts need lubrication to minimize friction, prevent wear, and reduce energy losses. Lubrication systems also help in heat dissipation, enhancing turbine longevity and operational stability.
5. Types of Turbines and Their Mechanisms
Turbines vary significantly in design and operational mechanism based on the type of energy they convert. Each type is tailored to specific fluid dynamics, fuel sources, and power plant designs. Here’s a detailed look at the main turbine types used in power generation:
Steam Turbines
Steam turbines are commonly used in coal-fired, natural gas, and nuclear power plants where steam generated by heating water is used to drive the turbine. Here’s how they operate:
- Mechanism of Operation:
- High-Pressure Steam Generation: Fuel combustion or nuclear reactions heat water, producing high-pressure steam. This steam flows into the turbine, where it rapidly expands, causing the turbine blades to rotate.
- Impulse vs. Reaction Designs: Steam turbines can be classified into two main designs based on how they convert steam energy:
- Impulse Turbines: These use a series of nozzles to direct high-velocity steam jets at the blades, transferring kinetic energy directly to the rotor. The pressure remains constant, but the steam speed decreases as it transfers energy.
- Reaction Turbines: In reaction turbines, steam pressure drops as it flows through the blades, creating a pressure gradient that pushes the blades forward. This type of turbine is commonly used in high-capacity power plants due to its higher efficiency.
- Role in Power Plants:
- Steam turbines are fundamental in traditional power plants because they can handle high temperatures and pressures. Their design allows for large-scale power generation, making them suitable for fossil fuel and nuclear plants.
Gas Turbines
Gas turbines are primarily used in natural gas power plants and can achieve high efficiency through direct combustion of fuel within the turbine. They operate on the Brayton cycle and differ from steam turbines in several key aspects:
- Mechanism of Operation:
- Combustion Chamber: Air is compressed, then mixed with natural gas and ignited in the combustion chamber. This combustion produces hot gases at high pressure and temperature.
- Expansion and Rotation: The hot gases expand rapidly as they pass through the turbine blades, creating rotational force. Unlike steam turbines, gas turbines operate with an open loop, exhausting the gas after it exits the turbine.
- Cooling Requirements:
- Due to the high temperatures generated by combustion, gas turbines require advanced cooling systems. Cooling can be achieved through air or oil systems to prevent damage to the turbine blades and rotor.
- Application:
- Gas turbines are valued for their ability to start up quickly, making them ideal for peaking power plants that respond to sudden increases in electricity demand. They’re also commonly used in combined cycle plants, where exhaust heat from a gas turbine generates steam for a secondary steam turbine, maximizing overall efficiency.
Hydroelectric Turbines
In hydroelectric power plants, water flow is used to drive the turbine. Hydroelectric turbines are optimized to harness kinetic and potential energy from water, making them suitable for renewable energy generation.
- Mechanism of Operation:
- Gravitational Energy: Water, stored at a height in reservoirs, flows down through the turbine when released. The flow speed and force depend on the water’s gravitational potential energy.
- Turbine Types: The three main types of hydroelectric turbines are:
- Francis Turbine: Suitable for medium to high heads (waterfall heights) and common in large hydroelectric dams. Francis turbines use a reaction-based mechanism with complex blade designs to optimize energy capture.
- Kaplan Turbine: Similar to a propeller, Kaplan turbines are used in low-head, high-flow situations. Their adjustable blades allow for high efficiency even with variable water flow.
- Pelton Wheel: Used in high-head, low-flow situations, the Pelton turbine is an impulse turbine where water jets strike individual buckets attached to the wheel, generating rotation.
- Role in Renewable Energy:
- Hydroelectric turbines are critical for sustainable power generation. They allow for large-scale, consistent power output and are highly efficient. Their use in pumped-storage systems also provides grid stability by storing excess electricity and releasing it when demand is high.
Wind Turbines
Wind turbines capture the kinetic energy of the wind and convert it into mechanical energy, which is then transformed into electricity. They are primarily used in wind farms for renewable power generation.
- Mechanism of Operation:
- Kinetic Energy of Wind: Wind turbines have large blades that act like airplane wings, creating lift as wind flows over them. This lift spins the rotor, transferring rotational energy to the generator.
- Yaw and Pitch Control: To maximize efficiency, wind turbines are equipped with a yaw mechanism that adjusts the rotor to face the wind direction. The blade angle, or pitch, is also adjustable to optimize energy capture based on wind speed.
- Types of Wind Turbines:
- Horizontal-Axis Wind Turbines (HAWT): These are the most common type and have a rotor that faces the wind horizontally.
- Vertical-Axis Wind Turbines (VAWT): Less common, VAWTs have a rotor positioned vertically, which can capture wind from any direction.
- Grid Connection and Efficiency:
- Wind turbines generate electricity intermittently, so they are typically connected to the grid alongside other power sources. To improve efficiency, modern wind turbines use advanced materials and control systems to adapt to varying wind speeds.
6. Operational Dynamics of Turbines
Turbines operate under various conditions, and understanding their start-up, shut-down, and load variability dynamics is essential for optimal performance.
- Start-Up and Shut-Down Processes:
- Starting a turbine requires gradually increasing speed and power output to reach optimal operating conditions without damaging the components. For example, steam turbines need a controlled heat-up period to avoid thermal stress, while gas turbines can ramp up faster.
- Shutting down a turbine involves carefully reducing speed, temperature, and pressure to protect components from sudden changes. A controlled cooling process helps extend component life and maintains plant safety.
- Load Variability and Performance Adjustments:
- Turbines adjust their output based on power demand. During peak load periods, they operate at full capacity, while at lower demand times, they may reduce output or shut down entirely in some cases. These changes impact the turbine’s efficiency, as full-load conditions are generally the most efficient.
- Efficiency Considerations During Partial Loads:
- Turbines are engineered to handle varying loads, but maintaining efficiency at partial loads remains challenging. Advanced control systems and materials help optimize efficiency across different load levels.
7. Efficiency and Optimization
The efficiency of turbines affects both economic and environmental outcomes, so optimization technologies and strategies are vital for modern power plants.
- Heat Recovery and Combined Cycles:
- Many power plants now use combined cycle technology, particularly with gas and steam turbines. Exhaust gases from a gas turbine are used to generate steam for a secondary steam turbine, capturing waste heat and converting it into additional electricity.
- Combined cycles can boost efficiency by up to 60%, compared to single-cycle systems.
- Advanced Technologies:
- Variable Geometry Turbines: Adjustable blade angles allow turbines to maintain efficiency at varying loads.
- Bladeless Turbines: Emerging technologies, like bladeless turbines, use oscillating motion rather than rotating blades, which may reduce maintenance and improve adaptability.
- High-Temperature Alloys and Coatings: Advanced materials, like ceramics and heat-resistant alloys, enable turbines to operate at higher temperatures, improving efficiency and reducing wear.
- Maintenance and Monitoring:
- Predictive maintenance is essential for turbines, reducing the risk of breakdowns and extending operational life. Advanced sensors and data analysis tools help monitor turbine health in real-time.
- AI and Machine Learning: These technologies analyze data trends to predict maintenance needs, optimize performance, and adjust turbine settings for maximum efficiency.
8. Environmental Impact and Innovations
With the growing focus on sustainable energy, turbines are evolving to reduce environmental impacts through innovative materials and renewable applications.
- Environmental Considerations:
- Advanced turbine designs and materials contribute to lower emissions and reduced fuel consumption, making traditional fossil-fuel-based turbines more efficient.
- Renewable Applications: Turbines in wind and hydro plants provide cleaner power options with minimal direct emissions, making renewable energy more viable on a large scale.
- Innovative Materials and Designs:
- Research into hydrogen-powered turbines, superconducting materials, and bladeless turbines promises even greater efficiency and environmental benefits.
- As renewable energy infrastructure expands, innovations in wind and hydroelectric turbines, such as floating offshore turbines and submersible turbines, are increasing their adaptability and efficiency.
9. Conclusion
Turbines remain central to electricity generation, embodying a fusion of mechanical, thermodynamic, and material sciences. By transforming fluid-based energy into mechanical power, turbines facilitate reliable and scalable electricity production across traditional and renewable energy systems. Continued advancements in turbine technology, materials, and energy recovery methods promise further improvements in efficiency and environmental sustainability. Turbines will continue to adapt, enhancing both the economic viability and ecological responsibility of global power generation.
Historical Development of Turbines
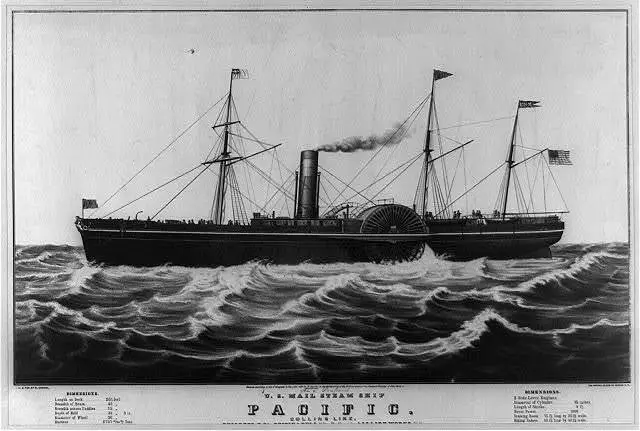
The use of turbines in power generation traces back to early concepts where simple mechanical turbines, like waterwheels, harnessed the energy of flowing rivers to drive mills and perform tasks such as grinding grain. This form of energy conversion represented one of the earliest utilizations of naturally occurring kinetic energy, laying the groundwork for more sophisticated turbine technologies.
The first significant advancement in turbine technology came during the Industrial Revolution, particularly with the advent of steam engines. James Watt, a pivotal figure in the evolution of steam technology in the late 18th century, introduced a more efficient engine that utilized steam expansion, paving the way for steam turbines. However, it wasn’t until the 1880s that Sir Charles Parsons developed the first successful steam turbine, which proved more efficient and could operate at a higher speed than reciprocating engines. Parsons’ turbine design transformed mechanical energy conversion, allowing it to be directly used for electricity generation, making it a cornerstone for modern power plants.
Over the 20th century, turbine technology advanced rapidly. Developments in gas turbines occurred with innovations in jet propulsion, which later adapted to power generation. Meanwhile, hydroelectric turbines became essential for harnessing renewable water energy, and wind turbines evolved from traditional windmills into high-tech systems for modern wind farms. These advancements in turbine technology allowed for increased efficiency, reliability, and the ability to harness a broader range of fuel types. Each type of turbine—whether steam, gas, hydroelectric, or wind—brought unique benefits and applications, establishing turbines as a universal solution for power generation.
The modern era has seen significant improvements in turbine materials, aerodynamics, and digital controls, enabling even higher efficiencies. Turbines today are central to both traditional and renewable energy plants, and they have been essential in the shift toward a cleaner and more efficient energy landscape.
2. Importance of Turbines in Energy Conversion
Turbines serve as the primary mechanism for transforming various forms of energy into electricity. They operate by converting high-pressure steam, combustion gases, or kinetic forces into mechanical energy, which is then used to turn an electric generator. This ability to directly link raw energy sources to electricity generation has made turbines indispensable in almost every type of power plant.
Turbines can utilize multiple energy types:
- Thermal Energy: In coal-fired and nuclear power plants, turbines convert thermal energy from high-pressure steam.
- Kinetic Energy: Wind and hydroelectric turbines use the natural kinetic energy of wind and water flow.
- Chemical Energy: In natural gas plants, turbines convert chemical energy from fuel combustion.
By enabling the conversion of these energy types into a usable electric form, turbines facilitate energy accessibility on a large scale. The efficiency of this energy conversion is crucial, as it directly impacts plant capacity, fuel consumption, and emissions. An efficient turbine design not only generates more electricity per unit of fuel but also minimizes waste and environmental impact. Thus, turbines are at the heart of both increasing power generation efficiency and meeting the growing demand for sustainable energy solutions.
3. Basic Working Principles of Turbines
The working principle of turbines revolves around energy transformation, where a fluid—steam, gas, water, or air—is forced over the turbine blades, creating mechanical rotation. This rotation is then transferred to a generator, producing electricity. The efficiency and effectiveness of turbines depend on the interplay of thermodynamic and fluid dynamics principles.
Thermodynamic Cycles: Turbines in power plants operate based on specific thermodynamic cycles designed to optimize energy transfer:
- Rankine Cycle: Used in steam turbines, the Rankine cycle heats water to create high-pressure steam, which flows through the turbine, expands, and does work. Afterward, the steam is condensed back into water, completing a closed loop that enables repeated use of the working fluid.
- Brayton Cycle: Gas turbines operate on the Brayton cycle, where air is compressed, mixed with fuel, and ignited. The resulting high-temperature gas expands through the turbine, generating rotational force before being exhausted in an open-loop system.
- Hydraulic Cycle: In hydroelectric turbines, gravitational potential energy from water is converted directly into mechanical energy, which drives the turbine. This cycle is simpler, as it does not require a combustion or heating process.
Fluid Dynamics and Force on Turbine Blades: The fluid flow is directed over the turbine blades in a controlled manner, either through a nozzle (as in impulse turbines) or by creating pressure differences across the blades (as in reaction turbines). The blade design—shape, angle, and material—ensures that maximum energy is transferred from the fluid to the turbine with minimal losses. The exact mechanism differs by turbine type:
- Impulse Turbines: The fluid strikes the blades at high speed, creating rotational force primarily from the impact.
- Reaction Turbines: Pressure changes across the blade surface drive the rotation, as is common in steam and hydroelectric turbines.
Together, these principles allow turbines to operate with precision, ensuring that the maximum amount of energy from the fuel or natural source is converted into mechanical work. Each turbine type employs unique designs and materials to achieve optimal efficiency, making turbines a versatile and adaptable technology in power generation.
4. Types of Turbines and Their Applications in Power Generation
Steam Turbines: Steam turbines are extensively used in coal, gas, and nuclear power plants, where they convert thermal energy from high-pressure steam into mechanical energy. This steam is generated by heating water using fuel combustion or nuclear reactions. Steam turbines often feature both impulse and reaction stages, allowing for efficient energy extraction at different steam pressures and speeds. They are particularly suitable for large-scale, high-capacity power plants, making them one of the most widely used turbine types.
Gas Turbines: Gas turbines, commonly used in natural gas power plants, generate electricity by burning fuel in a combustion chamber to produce high-temperature gases. These gases expand through the turbine, turning it to produce electricity. Gas turbines can start up quickly, making them ideal for peaking power plants that respond to fluctuations in demand. They are also central to combined cycle plants, where exhaust heat from the gas turbine is used to generate steam for an additional steam turbine, significantly improving efficiency.
Hydroelectric Turbines: Hydroelectric turbines harness the power of water flow to produce electricity, using the natural kinetic energy of rivers or reservoirs. There are several types of hydroelectric turbines, each suited to different conditions:
- Francis Turbines: Best for medium to high head applications.
- Kaplan Turbines: Similar to a propeller, these are suited for low head, high flow.
- Pelton Turbines: Used for high head, low flow applications.
These turbines play a major role in renewable energy generation, providing reliable and clean energy from natural water sources.
Wind Turbines: Wind turbines capture kinetic energy from wind and are predominantly used in wind farms. They consist of large blades attached to a rotor that spins with the wind’s force. The rotor is connected to a generator, converting rotational motion into electricity. Wind turbines are essential in the renewable energy sector, with both onshore and offshore installations contributing to sustainable power generation.
5. Technological Advancements in Turbine Efficiency
Turbine technology has evolved to enhance efficiency, reduce operational costs, and minimize environmental impact. Key advancements include:
- Blade Design and Aerodynamics: Innovations in blade shapes and materials, such as using composite materials and specialized alloys, improve turbine efficiency by optimizing energy capture.
- Combined Cycle Technology: In combined cycle plants, gas turbine exhaust heat is used to generate additional steam, allowing the use of both gas and steam turbines in one system. This increases plant efficiency significantly, achieving rates above 60% in some installations.
- Digital Monitoring and AI: Advanced sensors and digital control systems monitor turbine performance, predicting maintenance needs, and optimizing operation based on real-time data. AI and machine learning applications further enhance efficiency and reliability, making turbines more adaptable to operational demands.
These technologies have made turbines more resilient and adaptive to fluctuating loads, increased fuel efficiency, and enhanced overall power plant performance.
6. Environmental Impact and the Role of Turbines in Renewable Energy
Turbines are pivotal in the transition to cleaner energy sources, as they are essential in harnessing renewable resources like wind and water. They enable a substantial reduction in greenhouse gas emissions by improving fuel efficiency and supporting renewable energy generation. In natural gas plants, advanced turbines reduce carbon emissions through improved combustion efficiency. Renewable applications like wind and hydro turbines offer carbon-free energy, contributing to grid decarbonization.
Innovative designs are under development, such as hydrogen-powered turbines, which promise to further reduce emissions. Emerging technologies, such as bladeless turbines, aim to reduce noise and wildlife impacts, making renewable power generation more environmentally compatible.
7. Conclusion and Future Outlook
Turbines are indispensable in modern power generation, transforming natural and fuel-based energies into electricity with increasing efficiency and environmental responsibility. As technology advances, turbines are adapting to meet the needs of a sustainable energy future, incorporating renewable sources and maximizing efficiency in traditional fuel-based systems. Continued innovation in materials, design, and digital controls will likely expand their potential, positioning turbines as central to the transition toward cleaner and more reliable energy systems worldwide.
Overview of Turbine Function and Role in Power Generation
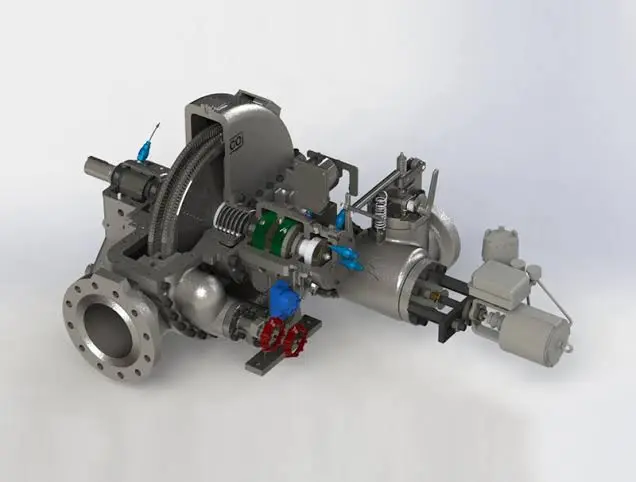
The function of a turbine is to convert energy from one form to another, predominantly transforming fluid energy—whether steam, gas, water, or wind—into mechanical energy that can then be converted into electrical power. This conversion process has positioned turbines at the heart of power generation in various types of plants, including fossil-fuel-based, nuclear, hydroelectric, and wind power plants.
Historically, turbines have been critical in the development of industrial-scale energy production, beginning with steam turbines in the late 19th century. Pioneers like Sir Charles Parsons advanced turbine technology by creating steam-driven designs that allowed for the large-scale production of electricity. As the power generation industry evolved, so did turbine technology, adapting to accommodate different fuels and energy forms. For instance, gas turbines emerged with the development of aviation engines and were later adapted to power generation. Similarly, the rise of renewable energy spurred advancements in hydroelectric and wind turbines, each tailored to optimize their unique energy sources.
In power plants, turbines serve as the primary mechanism of energy conversion. They are positioned between the energy source—whether fuel, nuclear reaction, water flow, or wind—and the generator, which converts mechanical rotation into electricity. The efficiency of this conversion process determines a plant’s fuel usage, output, and environmental impact. Today, turbines are indispensable across a wide range of power generation settings, offering versatile applications and ongoing advancements that improve efficiency, reduce emissions, and support the growing demand for renewable energy sources.
2. Principles of Energy Conversion in Turbines
Turbines operate on the fundamental principle of energy conversion, which is the transformation of thermal, kinetic, or potential energy into mechanical energy. This process involves several physical principles, particularly fluid dynamics and thermodynamics, which dictate how energy is transferred from a fluid to the turbine blades and ultimately to the generator.
- Energy Transformation Basics:
- The core function of a turbine involves receiving energy from a fluid—whether it be high-pressure steam, high-temperature gas, or fast-moving water or wind—and transferring it to the rotor and blades. As the fluid flows over the blades, it causes them to spin, creating mechanical rotation. This rotation is then transferred to a shaft connected to a generator, where it’s converted into electrical energy.
- The efficiency of energy transformation depends on the turbine’s design, fluid characteristics (such as pressure, velocity, and density), and thermodynamic principles that govern heat, pressure, and energy loss.
- Key Elements in Energy Transfer:
- Pressure: The pressure of the fluid plays a critical role in energy conversion. In steam turbines, for instance, high-pressure steam is directed over blades, causing them to spin as the steam expands. In hydroelectric turbines, the height of water (known as the “head”) creates pressure that drives turbine blades when released.
- Velocity: The velocity of the fluid impacts the amount of kinetic energy available for conversion. High-velocity fluids generate more rotational force on turbine blades. This principle is critical in wind and impulse turbines, where the kinetic energy of the fluid directly drives blade movement.
- Temperature: In gas and steam turbines, the temperature difference between the high-energy fluid and its surrounding environment affects the turbine’s efficiency. High temperatures lead to greater expansion and more energy transfer, but they also require turbines made from durable, heat-resistant materials.
- Laws of Thermodynamics in Turbine Operation:
- First Law of Thermodynamics: The first law, or the principle of energy conservation, dictates that the energy entering the turbine in the form of fluid energy will equal the energy leaving the turbine. This includes both the mechanical energy transferred to the generator and any losses through heat or friction.
- Second Law of Thermodynamics: This law governs the direction of energy transfer, stating that energy naturally flows from high to low energy states. For turbines, this means steam or gas flows from high-pressure areas to low-pressure ones, creating rotation in the process. The second law also introduces the concept of entropy, which affects efficiency by accounting for irreversibilities in the system.
These principles underscore the core function of turbines as energy transformers, carefully designed to maximize the energy transferred from fluid to mechanical form with minimal losses.
3. Mechanics of Turbines: Components and Design
A turbine’s mechanics rely on an array of components designed to optimize the capture and conversion of energy from a fluid. Each component, from rotors to blades, serves a distinct purpose in facilitating smooth, efficient energy transfer.
- Rotor, Shaft, and Blade Mechanics:
- Rotor: The rotor is the main rotating component in a turbine, housing the shaft and blades. It directly connects to the generator and serves as the central mechanism for energy transfer. Turbine rotors are built to withstand high rotational speeds and intense forces.
- Shaft: The shaft is the connecting component that transmits rotational force from the rotor to the generator. It’s designed to resist bending and withstand high mechanical stress, often using high-strength steel or alloy to ensure durability.
- Blades: Blades are essential to energy capture, as they intercept fluid flow and transfer energy to the rotor. They are often designed to have a specific curvature or angle that optimizes interaction with the fluid, such as maximizing pressure or capturing kinetic energy.
- Blade Design and Types:
- Impulse Blades: In impulse turbines, blades are designed to intercept high-speed fluid jets. The fluid’s pressure remains constant, but its velocity decreases as it strikes the blades, imparting kinetic energy to the rotor.
- Reaction Blades: Reaction blades use a change in pressure to generate rotation. As fluid flows over these blades, pressure drops, and a force is exerted across the blade surface, pushing it forward. Reaction blades are common in steam turbines, where pressure differentials are high.
- Blade Materials: Turbine blades must withstand high temperatures, pressure, and corrosion. In gas turbines, for example, blades are often made of superalloys or ceramic composites to resist thermal stress.
- Nozzles and Casings:
- Nozzles: In impulse turbines, nozzles direct the fluid into high-speed jets that strike the blades. By precisely controlling the flow, nozzles optimize the force applied to the rotor and improve efficiency.
- Casings: The turbine casing surrounds the rotor and blades, managing fluid flow and preventing leaks. Casings are typically made from materials that resist high temperatures and corrosion, as they must contain the fluid’s pressure and direct its flow within the turbine.
- Cooling and Lubrication Systems:
- Cooling: Turbines generate heat during operation, particularly gas turbines, which can reach temperatures exceeding 1,500°C. Cooling systems, such as air, water, or oil coolants, help manage this heat to protect components.
- Lubrication: Bearings and shafts within the turbine require lubrication to reduce friction, prevent wear, and extend lifespan. Lubrication systems circulate oil through high-friction parts, also assisting in heat dissipation.
4. Thermodynamic Cycles and Their Applications
Thermodynamic cycles are at the heart of turbine operation, dictating the sequence of processes that convert energy into work. Each type of turbine uses a specific cycle suited to its fuel or resource, and these cycles are carefully engineered to maximize efficiency. The three primary thermodynamic cycles are the Rankine cycle (used in steam turbines), Brayton cycle (used in gas turbines), and the Hydraulic cycle (used in hydroelectric turbines).
The Rankine Cycle (Steam Turbines)
The Rankine cycle is commonly used in power plants that operate steam turbines, such as coal, natural gas, and nuclear power plants. This cycle involves heating, expanding, and condensing water, allowing for repeated energy extraction.
- Heat Absorption: Water is heated in a boiler to create high-pressure steam. This heat source could be fossil fuels, nuclear reactions, or geothermal energy.
- Expansion: The high-pressure steam flows into the turbine, expanding as it passes through the blades. This expansion pushes the turbine blades, converting thermal energy into mechanical work.
- Condensation: After leaving the turbine, the low-pressure steam enters a condenser, where it cools and condenses back into water.
- Pressurization and Recycling: The condensed water is pressurized by a pump and fed back into the boiler, completing the cycle.
The Rankine cycle’s closed-loop system allows for efficient energy use and minimizes waste. Efficiency is further increased by using higher pressures and temperatures, as well as by employing superheating and reheat cycles.
The Brayton Cycle (Gas Turbines)
The Brayton cycle is the thermodynamic cycle used in gas turbines, which are common in natural gas power plants and jet engines. It involves compressing, combusting, and expanding air to generate power.
- Compression: Air is drawn into the compressor, where it is pressurized. Compressing the air increases its energy potential for the combustion stage.
- Combustion: The compressed air is mixed with fuel (usually natural gas) in a combustion chamber and ignited. This produces high-temperature, high-pressure gases.
- Expansion: These gases expand as they flow through the turbine, creating rotational force. This mechanical work drives both the generator and the compressor.
- Exhaust: The expanded gases are expelled from the turbine, completing the open cycle.
Gas turbines achieve higher efficiency in combined cycle plants, where exhaust heat is used to create steam for a secondary steam turbine, capturing additional energy from the fuel.
The Hydraulic Cycle (Hydroelectric Turbines)
In hydroelectric power plants, gravitational potential energy in stored water is converted into kinetic energy, which drives the turbine. Unlike the closed-loop Rankine cycle or the open Brayton cycle, the hydraulic cycle relies on a continuous flow of water.
- Potential Energy: Water stored at a height (in a dam or reservoir) possesses gravitational potential energy.
- Flow and Acceleration: When released, the water flows down through pipes or channels, accelerating due to gravity.
- Energy Transfer: The moving water flows over the turbine blades, imparting kinetic energy and creating rotation. This rotational energy is converted into electricity.
- Continuous Cycle: The water exits the turbine and flows downstream, maintaining a continuous cycle of energy generation.
The hydraulic cycle is highly efficient due to minimal energy loss and is renewable as long as water flow is sustained. Hydroelectric turbines, therefore, play a significant role in sustainable energy systems.
5. Fluid Dynamics in Turbine Operation
Fluid dynamics is critical to turbine functionality, as the movement of fluid—whether steam, gas, or water—drives the turbine blades. Fluid flow characteristics such as pressure, velocity, and turbulence directly influence the efficiency and output of a turbine.
- Fluid Flow and Pressure Gradients:
- Fluid flow is the motion of liquid or gas through a turbine, directed by pressure gradients. In a turbine, high-pressure fluid flows to lower-pressure areas, producing energy as it moves.
- Impulse Turbines: In impulse turbines, fluid flows at high speed through nozzles, creating jets that strike the blades, imparting kinetic energy and causing rotation.
- Reaction Turbines: Reaction turbines rely on pressure changes within the blades themselves. As fluid moves from high to low pressure, it accelerates and propels the blades forward.
- Velocity and Blade Interaction:
- The velocity of the fluid determines the kinetic energy available for conversion. High-velocity fluids produce more force on the turbine blades, maximizing mechanical output.
- The angle and curvature of turbine blades are carefully designed to capture fluid energy effectively. In impulse turbines, blades are curved to direct fluid flow efficiently, while in reaction turbines, the blade shape allows pressure changes to optimize energy transfer.
- Aerodynamics and Blade Design:
- Turbine blades are designed to minimize air resistance and turbulence, maximizing the smooth flow of fluid across their surfaces. Aerodynamic efficiency is especially critical in wind and gas turbines, where high-speed air or gas flows interact with the blades.
- Lift and Drag: In wind turbines, blade shapes are similar to airplane wings, creating lift when wind flows over them. This lift causes rotation with minimal drag, optimizing energy capture.
- Minimizing Turbulence:
- Turbulence disrupts fluid flow, causing inefficiencies in energy transfer. Turbine designs aim to maintain laminar (smooth) flow over the blades, reducing drag and preventing energy loss.
- Nozzles and vanes help control fluid flow direction and reduce turbulence, ensuring that fluid impacts the blades at optimal angles.
Fluid dynamics principles, when applied to turbine design, allow for precise control over energy transfer, enhancing the turbine’s ability to convert fluid energy efficiently into mechanical power.
6. Types of Turbines and Their Functionality
Each type of turbine—steam, gas, hydroelectric, and wind—functions differently based on the energy source and operational environment. Here’s a closer look at each type:
Steam Turbines
Steam turbines are widely used in thermal power plants, where steam generated from heated water drives the turbine.
- Impulse Mechanism: In impulse steam turbines, high-velocity steam jets strike the blades, transferring kinetic energy to the rotor.
- Reaction Mechanism: Reaction steam turbines rely on pressure changes across the blades, creating a pushing force that drives rotation. These are often used in large-scale power plants because they are highly efficient in handling high-pressure steam.
Steam turbines are robust, capable of high power outputs, and operate efficiently at high pressures and temperatures, making them ideal for coal, gas, and nuclear plants.
Gas Turbines
Gas turbines are primarily used in natural gas plants, where combustion of natural gas produces high-temperature gases that drive the turbine.
- Combustion Process: In gas turbines, compressed air is mixed with fuel and ignited, producing hot gases that expand and flow through the turbine.
- Combined Cycle Functionality: Gas turbines are often part of combined cycle plants, where waste heat is used to generate steam for a secondary turbine, increasing efficiency.
Gas turbines can quickly start up, making them suitable for peaking power plants, and their high-speed operation allows for efficient energy conversion in natural gas facilities.
Hydroelectric Turbines
Hydroelectric turbines convert water flow energy into mechanical power and are widely used in renewable power generation.
- Francis Turbines: Used for medium to high heads, these turbines use reaction blades and can handle large water flows.
- Kaplan Turbines: Kaplan turbines function like propellers, with adjustable blades suited to variable flow conditions.
- Pelton Turbines: In high-head, low-flow settings, Pelton turbines use impulse-driven buckets to capture energy from water jets.
Hydroelectric turbines are efficient, sustainable, and capable of providing base load power due to the constant availability of water flow.
Wind Turbines
Wind turbines harness the kinetic energy of wind to generate electricity, commonly used in wind farms.
- Horizontal-Axis Wind Turbines (HAWT): The most common type, with blades that rotate around a horizontal axis facing the wind.
- Vertical-Axis Wind Turbines (VAWT): Less common, these turbines capture wind from any direction, making them suitable for turbulent or variable wind conditions.
Wind turbines utilize aerodynamic blade design to create lift, converting wind energy into mechanical rotation. Control systems adjust blade pitch and yaw to maximize efficiency based on wind conditions.
7. Efficiency, Optimization, and Maintenance
Efficiency in turbine operation is crucial, as it impacts fuel usage, operational cost, and emissions. Several techniques optimize turbine efficiency and performance.
- Energy Efficiency Principles:
- Turbines are optimized to operate close to their maximum efficiency at full load. However, many power plants experience variable demand, necessitating partial load operation. Advanced control systems help maintain efficiency by adjusting flow, pressure, and blade angles according to load changes.
- Load Variability and Partial Load Efficiency:
- Turbines must adapt to varying power demands, adjusting their output based on load requirements. This variability can reduce efficiency, so turbines often include mechanisms like variable inlet vanes and adjustable blades to maintain efficiency across different loads.
- Maintenance Strategies:
- Predictive Maintenance: Sensors monitor component wear, heat, and pressure to predict when maintenance is required, reducing downtime and avoiding costly breakdowns.
- Condition-Based Maintenance: Regularly assessing turbine components helps ensure they remain within optimal operating conditions, preventing unexpected failures and improving efficiency.
- Role of AI, Sensors, and Machine Learning:
- Advanced monitoring systems collect data on turbine performance, which is analyzed using machine learning to predict maintenance needs, optimize operational settings, and identify potential efficiency improvements.
Efficient maintenance and optimization strategies ensure turbines operate reliably and economically, maximizing power output and reducing environmental impact.
8. Future of Turbine Technology
The future of turbines in power generation looks promising, with advancements in materials, design, and renewable energy applications leading the way.
- Innovative Materials and Blade Designs:
- Research into lightweight composites and high-temperature alloys aims to create blades that withstand higher pressures and temperatures, improving efficiency and reducing wear.
- Aerodynamic Innovations: Improved blade shapes and control mechanisms enhance energy capture, especially in wind and hydroelectric turbines, where efficiency is crucial for maximizing renewable energy output.
- Emerging Turbine Types:
- Hydrogen Turbines: Turbines designed to use hydrogen as fuel are being developed to reduce carbon emissions in power generation. These turbines offer a potential pathway for clean, high-efficiency energy.
- Bladeless Turbines: Experimental bladeless designs, such as oscillating turbines, reduce maintenance needs and environmental impact, especially for wind energy.
- Environmental Prospects:
- The use of turbines in renewable energy applications will likely expand as the world transitions toward cleaner energy sources. Turbines in hydroelectric, wind, and potentially hydrogen-fueled systems will play an increasingly vital role in sustainable power.
The continuous evolution of turbine technology promises to support both traditional and renewable power generation in a more sustainable, efficient, and cost-effective way.
Rotor and Shaft
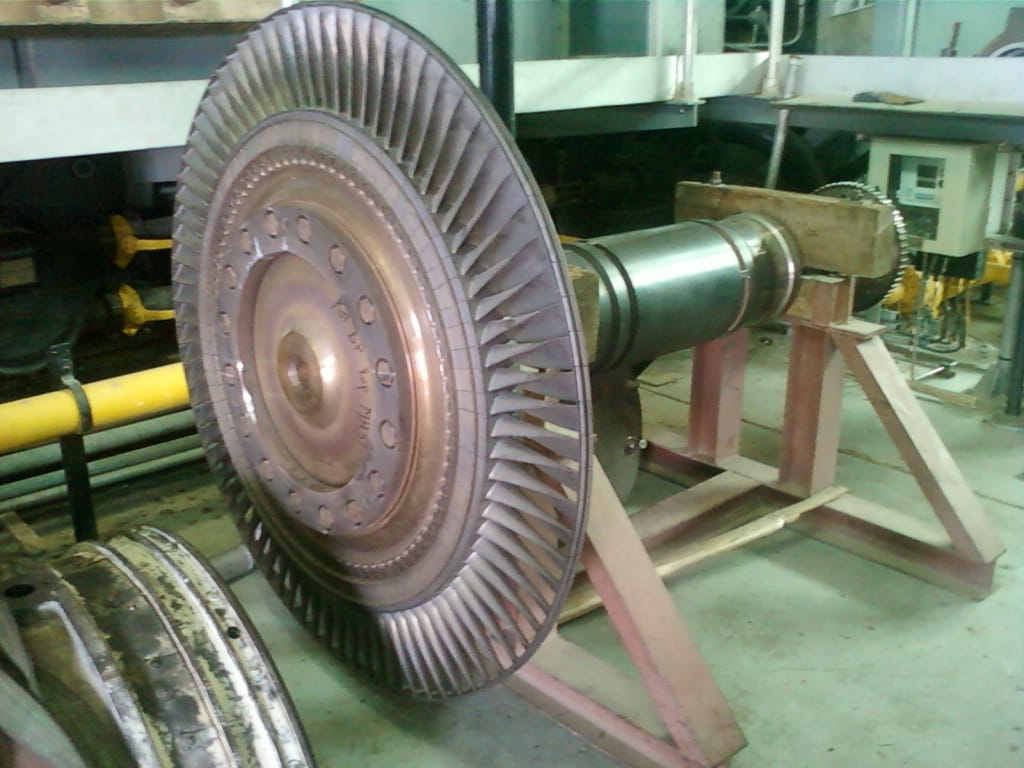
The rotor and shaft form the core of the turbine’s mechanical system, responsible for converting fluid-based energy into rotational energy that can be transferred to an electric generator. As the turbine’s primary rotating assembly, the rotor and shaft are engineered to handle the intense forces generated by high-speed rotation, fluid pressure, and mechanical loads.
Rotor:
- The rotor consists of the main body of the turbine’s rotating assembly, including the shaft and the blades attached to it. It’s designed to capture and convert the energy from fluid flow, turning this energy into the rotational force necessary to drive the generator.
- The rotor is designed with precision to ensure it can handle thousands of revolutions per minute (RPM) without incurring significant stress or deformation. Engineers often optimize the rotor’s weight and balance to minimize vibrations and maximize efficiency during operation.
Shaft:
- The shaft connects the rotor to the generator, transmitting the rotational energy produced by the rotor to the generator, where it is converted into electricity. Shafts are typically crafted from high-strength materials like stainless steel or alloyed metals, which provide the durability needed to handle high rotational speeds and resist bending or twisting under stress.
- Shafts in large turbines are often hollow, reducing weight and enhancing their resistance to stress. Advanced manufacturing techniques like forging and heat treatment are applied to strengthen the shaft, which ensures longevity and high performance.
Together, the rotor and shaft must endure the turbine’s high-speed operation and the resulting centrifugal forces. This makes material strength, precision engineering, and vibration control critical factors in rotor and shaft design, as they contribute to the turbine’s efficiency and operational stability.
2. Blades
Turbine blades are the components directly responsible for capturing the energy from the moving fluid—be it steam, gas, water, or wind—and converting it into rotational motion. Blades are perhaps the most complex and highly engineered parts of a turbine due to their critical role in energy conversion and the extreme conditions they face during operation.
Role of Blades in Energy Capture and Conversion:
- Turbine blades interact directly with the fluid, capturing its kinetic or thermal energy and converting it into mechanical force. As fluid flows over the blades, it transfers energy to the rotor, initiating rotation.
- Blades must be precisely shaped and positioned to capture as much energy as possible from the fluid. Each blade is strategically curved and angled to optimize interaction with the flow, minimizing energy loss and maximizing efficiency.
Types of Blades:
- Impulse Blades: These blades are primarily used in impulse turbines. In an impulse turbine, high-velocity fluid jets from nozzles strike the impulse blades, transferring kinetic energy directly to the rotor. The fluid’s pressure remains constant as it passes over impulse blades, but its speed decreases as it imparts energy.
- Reaction Blades: Reaction blades rely on pressure differences within the fluid. As the fluid flows over the reaction blades, its pressure drops, creating a force that pushes the blades and drives rotation. Reaction turbines, often used in steam and hydroelectric power, tend to have higher efficiency at variable loads due to this pressure-based energy transfer.
Materials and Coatings:
- Turbine blades are typically made from materials that can withstand high temperatures, pressures, and corrosion. Nickel-based superalloys, titanium, and ceramic composites are common materials for gas and steam turbine blades due to their heat resistance and durability.
- High-performance coatings, such as thermal barrier coatings (TBCs), are applied to blades, especially in gas turbines. These coatings protect blades from extreme temperatures, extending their life and improving thermal efficiency.
Aerodynamic Design and Blade Angle:
- Blade shape and angle are essential for maximizing energy capture. Engineers use computational fluid dynamics (CFD) to design blade profiles that enhance aerodynamic efficiency and reduce drag.
- Blades are set at specific angles, or pitch, to adjust their interaction with the fluid flow. In wind turbines, for instance, blade pitch control adjusts the angle depending on wind speed, maximizing energy capture and protecting the turbine during high winds.
Blades are thus central to the turbine’s function, as they directly influence its power output, efficiency, and operational lifespan. Their advanced materials and aerodynamic design enable turbines to operate at high efficiency under demanding conditions.
3. Casing and Housing
The casing or housing of a turbine serves as the protective shell that contains and directs the flow of the working fluid over the blades. The casing ensures that high-pressure fluids are efficiently routed through the turbine, maintaining control over flow direction and pressure while also protecting the rotor and blades from external elements.
Structure and Function:
- The turbine casing is designed to withstand the high pressures and temperatures associated with fluid flows, particularly in steam and gas turbines. It encloses the rotor and blades, directing fluid along the intended path and preventing leaks that could reduce efficiency.
- Casings are equipped with seals and insulation to contain the high-pressure fluid and maintain the internal environment. Seals are especially important in maintaining pressure and preventing leaks, which would result in energy losses.
Materials:
- Casings are typically made from materials that can endure the thermal and pressure stresses of turbine operation. Heat-resistant alloys or high-strength steels are often used in steam and gas turbines to withstand both high temperatures and pressure fluctuations.
- The interior surface of the casing may also be coated or lined with corrosion-resistant materials, which protect it from the erosive effects of high-velocity fluid and increase the turbine’s durability.
By maintaining a controlled environment within the turbine, the casing ensures that the working fluid flows over the blades as designed, optimizing energy capture and providing an essential layer of protection for the turbine’s internal components.
4. Nozzles and Guide Vanes
Nozzles and guide vanes are essential for directing fluid flow within a turbine, ensuring that it strikes the blades at the optimal angle and speed to maximize energy transfer. These components are particularly important in impulse and reaction turbines, where precise fluid flow control is critical.
Nozzles in Impulse Turbines:
- In impulse turbines, nozzles direct high-pressure fluid into jets that strike the impulse blades. The nozzles are precisely angled to optimize the impact, maximizing the kinetic energy transfer from the fluid to the blades.
- Nozzle design is crucial for impulse turbines, as they must control fluid speed and direction without causing excessive turbulence. Materials like ceramics and stainless steel are often used to resist wear from high-speed fluid.
Guide Vanes in Reaction Turbines:
- Guide vanes are commonly used in reaction turbines, where they direct fluid flow over the blades to ensure a smooth transition from high to low pressure. This controlled flow helps create a pressure gradient across the blade surface, driving rotation.
- Guide vanes are generally fixed, but some systems use adjustable vanes to fine-tune fluid flow and improve efficiency under varying loads.
Both nozzles and guide vanes contribute to fluid flow stability and minimize energy loss, making them integral to the turbine’s efficiency. Their design and materials are chosen to endure high-speed impacts and temperatures, extending their operational life.
5. Cooling and Lubrication Systems
Turbines generate substantial heat during operation, especially in high-temperature environments like gas turbines. Cooling and lubrication systems are therefore critical to prevent overheating and reduce wear on moving parts.
Cooling Systems:
- Cooling systems help regulate the temperature of turbine components, especially the blades and rotor, which experience intense thermal stress. Air, oil, and water are common cooling media, circulated through or around critical components to dissipate heat.
- In gas turbines, internal cooling channels within the blades circulate cooling air, protecting the blade material from extreme temperatures that can exceed 1,500°C. This allows the turbine to operate at higher temperatures, improving efficiency.
Lubrication Systems:
- Lubrication reduces friction between moving parts, such as bearings and the shaft. It prevents wear, lowers the risk of overheating, and improves mechanical efficiency.
- Turbine lubrication systems typically use high-grade synthetic oils that remain effective under high temperatures and pressures. The lubrication system often includes filters and cooling units to maintain oil quality, ensuring consistent performance.
Effective cooling and lubrication systems are vital to turbine longevity and efficiency, enabling components to withstand intense operating conditions while minimizing maintenance requirements.
6. Control and Monitoring Systems
Modern turbines are equipped with control and monitoring systems that optimize operation, adjust to load changes, and monitor component health.
Adaptive Control Mechanisms:
- Control systems automatically adjust parameters like fluid flow, blade angle, and rotational speed based on load demands, optimizing efficiency under varying operating conditions.
- Wind turbines, for instance, use control systems to adjust blade pitch and yaw to capture maximum wind energy.
Sensors and Monitoring for Predictive Maintenance:
- Sensors placed throughout the turbine monitor parameters like temperature, pressure, vibration, and rotational speed. This data is analyzed to detect potential issues before they lead to failure.
- Predictive maintenance uses data from sensors to anticipate maintenance needs, reducing downtime and prolonging component life.
These control and monitoring systems enhance turbine performance, reliability, and lifespan, ensuring efficient power generation and reducing operational costs.
Introduction to the Rotor and Shaft
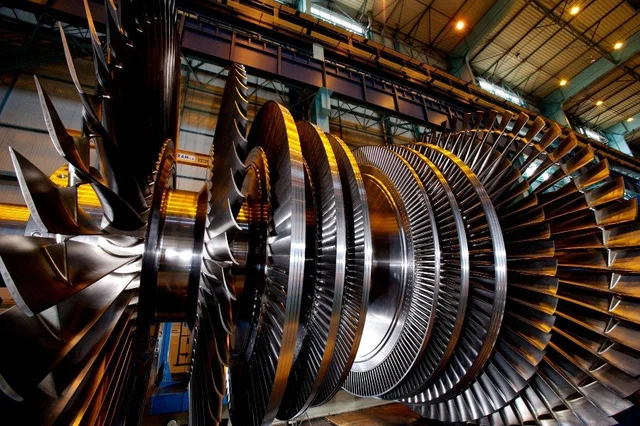
In turbine systems, the rotor and shaft are the central rotating components responsible for converting the energy of moving fluids—steam, gas, water, or wind—into mechanical energy that drives the electric generator. The rotor, housing the blades, captures fluid energy, while the shaft transmits this rotational energy to the generator. Together, these two components are essential for energy conversion efficiency, operational stability, and the longevity of the turbine.
The role of the rotor and shaft varies depending on the type of turbine:
- In steam turbines, the rotor is connected to high-speed blades, capturing energy from high-pressure steam. The shaft then transmits this rotation to the generator.
- Gas turbines operate similarly but require rotors and shafts to handle even higher temperatures due to combustion processes.
- Hydroelectric turbines have larger, slower-rotating rotors that handle the steady force of water flow.
- Wind turbines use rotors with large blades designed to capture wind energy efficiently, while the shaft transfers the lower-speed rotation to the generator.
The importance of the rotor and shaft in turbines extends beyond simply converting energy. Their structural integrity and precise design are crucial for minimizing vibrations, preventing material fatigue, and ensuring efficient energy transfer. Without robust and well-engineered rotors and shafts, turbines would suffer from inefficiencies, increased maintenance costs, and shorter operational lifespans.
2. Rotor Design and Function
The rotor is the main rotating assembly within the turbine, consisting of a central hub and blades attached to it. Its primary purpose is to convert the kinetic or thermal energy from fluid flow into mechanical energy, generating the rotational force that drives the shaft and subsequently the generator. The design of the rotor must account for efficiency, structural stability, and durability.
Function of the Rotor in Energy Capture and Conversion:
- The rotor’s blades are designed to interact optimally with the flow of fluid, whether it’s high-pressure steam, combustion gases, water, or wind. As the fluid moves through the turbine, it transfers energy to the blades, causing the rotor to spin.
- This spinning motion is the mechanical energy that powers the shaft. The speed, balance, and efficiency of this rotation directly affect the turbine’s power output and overall efficiency.
Types of Rotors Based on Turbine Types:
- Steam Turbine Rotors: These rotors are built to handle the high-speed, high-pressure environment of steam turbines. They are usually long and consist of a series of blades positioned to maximize the capture of thermal energy. Some steam turbines have multiple rotor stages to efficiently use steam at varying pressure levels.
- Gas Turbine Rotors: Gas turbine rotors must withstand extremely high temperatures due to the combustion of fuel within the turbine. These rotors are often shorter than those in steam turbines but must be made from materials resistant to thermal stress. Cooling channels may be integrated into the rotor to dissipate heat and protect components.
- Hydroelectric Turbine Rotors: In hydroelectric plants, the rotor (or “runner”) is larger and rotates more slowly than in steam or gas turbines. These rotors are typically designed to handle large water flows and can include multiple blades (such as in Kaplan and Francis turbines) to optimize energy capture from gravitationally driven water flows.
- Wind Turbine Rotors: Wind turbine rotors feature large, aerodynamically shaped blades attached to a central hub. They rotate at slower speeds compared to steam or gas turbines, but the rotor’s large diameter allows it to capture wind energy effectively. Yaw and pitch control mechanisms adjust the rotor’s position relative to the wind for optimal performance.
Aerodynamic and Structural Considerations in Rotor Design:
- Rotors are engineered to achieve the best balance between aerodynamics (to reduce drag) and structural integrity (to handle stress). In wind turbines, blades are shaped to maximize lift and minimize drag, improving energy capture from wind. In hydroelectric turbines, the rotor blades are optimized to handle steady, high-force water flow without causing cavitation.
- Engineers use computational fluid dynamics (CFD) and physical testing to refine rotor designs, ensuring that they achieve high efficiency and low energy loss. This attention to aerodynamics is especially important in wind and gas turbines, where minimizing drag and turbulence increases efficiency.
Balancing, Vibration Control, and Rotational Stability:
- A well-balanced rotor is crucial for the turbine’s stability, as imbalances can cause vibrations that reduce efficiency and cause mechanical wear. Rotors are balanced during manufacturing to ensure even weight distribution.
- Anti-vibration dampeners and shock absorbers are sometimes used to minimize vibration, especially in high-speed turbines. This reduces strain on the turbine and improves its operational life.
- Precision-engineered rotors maintain rotational stability even at high speeds, enabling efficient and smooth energy transfer from fluid to mechanical energy.
The rotor’s design, material choice, and engineering are all aimed at maximizing energy conversion efficiency, maintaining stability, and withstanding the intense forces generated by high-speed rotation and fluid flow.
3. Shaft Design and Function
The shaft is a critical component in the turbine system, acting as the conduit that transmits rotational energy from the rotor to the generator. Its design must ensure durability, flexibility, and the capacity to handle high rotational speeds without failure.
Function of the Shaft:
- The shaft is responsible for transferring the mechanical rotation from the rotor to the generator, where it is converted into electrical energy. This function is essential in ensuring consistent and efficient power generation.
- Shafts in turbines operate at high rotational speeds, particularly in steam and gas turbines, where RPMs can exceed thousands of revolutions per minute. This requires precision in both design and materials to avoid structural fatigue.
Types of Shafts: Solid vs. Hollow:
- Solid Shafts: These are typically used in smaller turbines or in applications where maximum durability is required. Solid shafts are simpler to manufacture and can handle high rotational forces, but they are also heavier, which can be a disadvantage in high-speed turbines.
- Hollow Shafts: Hollow shafts reduce weight and enhance flexibility, making them more suitable for larger, high-speed turbines where weight reduction improves efficiency and reduces stress. Hollow shafts are often reinforced with internal structures to maintain strength.
Materials and Structural Considerations:
- Shafts are commonly made from high-strength materials such as stainless steel or alloy steels, chosen for their ability to handle mechanical stress, resist bending, and endure prolonged operation without deformation.
- Special coatings or heat treatments are sometimes applied to improve wear resistance, reduce friction, and handle high temperatures, especially in gas turbines where the shaft may be exposed to heat from combustion gases.
Engineering Techniques for Stability and Durability:
- To prevent mechanical failure, engineers use techniques like finite element analysis (FEA) during the design phase, assessing how the shaft will respond to stresses at various points along its length.
- The shaft is often reinforced at points that experience high rotational force, such as near the rotor or where it connects to the generator. This reinforcement helps avoid stress fractures and bending, which could otherwise lead to turbine failure.
- Fatigue resistance is an essential quality, as turbine shafts operate continuously for long periods. High-quality alloys and manufacturing techniques like forging and precision machining increase the shaft’s resistance to fatigue.
A well-engineered shaft supports the efficient transfer of mechanical energy, ensuring that turbines operate smoothly, reliably, and with minimal downtime.
4. Materials and Manufacturing of Rotor and Shaft
The materials and manufacturing processes used for turbine rotors and shafts are chosen to optimize performance, durability, and efficiency under demanding conditions.
Materials:
- Nickel-Based Alloys: Often used in gas turbine rotors and shafts, these alloys offer high-temperature resistance, crucial in environments with combustion.
- Stainless and Alloy Steels: Commonly used for steam and hydroelectric turbine shafts, these materials provide the durability and wear resistance necessary for long-term operation.
- Titanium and Composites: In high-efficiency, lightweight turbines (such as some wind turbines), titanium and composite materials reduce weight while maintaining strength.
Manufacturing Techniques:
- Forging: Shafts and rotors are often forged to improve structural integrity and reduce weak points. Forging enhances the material’s resistance to stress, improving longevity.
- Heat Treatment: Heat treatment is applied to improve the material’s hardness, making it more resistant to wear and tear. This is especially useful in high-temperature applications like gas turbines.
- Precision Machining: After forging, precision machining creates the detailed shapes and fits required for efficient energy transfer. Rotors and shafts are finely machined to reduce surface roughness, enhancing durability and minimizing friction losses.
These materials and techniques ensure that the rotor and shaft can withstand the stresses of high-speed rotation, pressure, and temperature, enabling the turbine to operate reliably over extended periods.
5. Maintenance and Operational Challenges
The rotor and shaft face unique operational challenges that can lead to wear and require regular maintenance to sustain efficient turbine operation.
Common Challenges:
- Wear and Tear: Continuous rotation at high speeds can cause wear on the rotor and shaft, especially at points of contact with other components.
- Thermal Expansion: High temperatures, particularly in gas turbines, cause thermal expansion in rotors and shafts. Precision engineering and cooling systems mitigate this issue.
- Corrosion: Shafts and rotors in steam and hydroelectric turbines are exposed to moisture, making corrosion a risk. Protective coatings and corrosion-resistant materials help extend component life.
Maintenance Techniques:
- Predictive Maintenance: Sensors monitor vibrations, temperature, and pressure changes, identifying potential issues before they lead to failure.
- Balancing and Alignment: Regular balancing reduces vibration and wear, ensuring smooth operation. Realigning components also minimizes stress on the shaft.
- Lubrication: Regular lubrication minimizes friction between moving parts, extending component life and preventing overheating.
These maintenance practices help prolong the operational lifespan of turbine rotors and shafts, ensuring they continue to perform efficiently and reliably.
Introduction to Turbine Blades
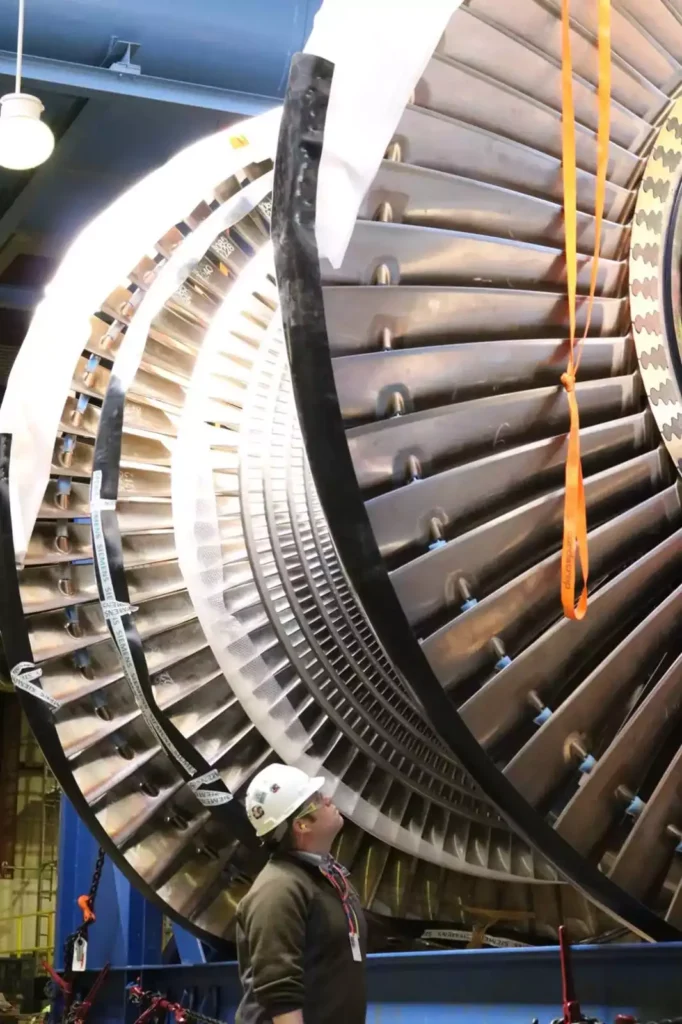
Turbine blades are essential components of any turbine system, as they directly interact with the moving fluid—whether it is steam, gas, water, or wind—to capture its energy and convert it into mechanical motion. This motion drives the turbine’s rotor and ultimately powers the generator to produce electricity. The blades’ efficiency and durability are central to the overall performance of the turbine, as they are responsible for the precise conversion of fluid energy into rotational energy.
The shape, size, and material of turbine blades vary significantly across different types of turbines to accommodate specific fluid dynamics:
- Steam and gas turbines typically have high-speed blades optimized for handling high-pressure and high-temperature steam or combustion gases.
- Hydroelectric turbines use larger, slower-rotating blades designed to capture energy from water flow, often within controlled channels.
- Wind turbines feature long, aerodynamically shaped blades that capture wind energy and convert it into rotational motion.
Given the crucial role that blades play in maximizing energy capture, they are engineered to withstand extreme operating conditions, including high pressure, temperature, and wear. Advances in materials and aerodynamic design have allowed turbine blades to achieve higher efficiency, longer operational lifespans, and greater reliability in diverse power generation applications.
Types of Turbine Blades
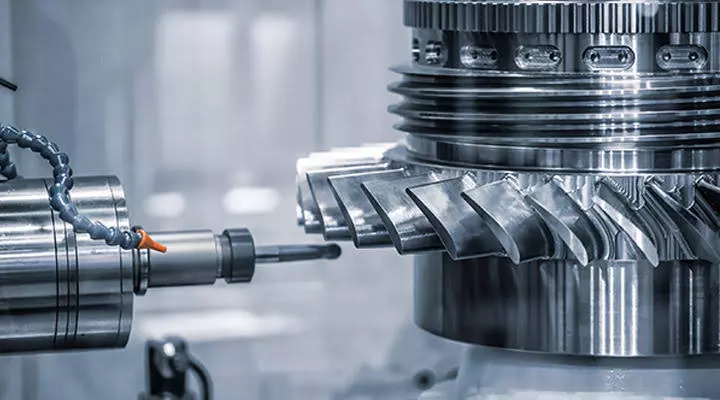
Turbine blades are typically classified into two main types based on their mode of energy capture: impulse blades and reaction blades. Each type serves a unique function, adapting to the specific energy characteristics of the fluid in various turbine applications.
Impulse Blades:
- In impulse turbines, impulse blades are struck by high-speed jets of fluid that transfer kinetic energy directly to the blade surfaces. The fluid pressure remains constant as it moves over the blades, while its velocity decreases as it transfers energy to the rotor.
- Impulse blades are typically used in applications where the fluid can be directed through nozzles to create high-speed jets, such as in steam turbines. Each nozzle accelerates the fluid before it hits the blade, producing a powerful force that spins the rotor.
- These blades are designed with curvature and angles that allow the fluid to strike at an optimal angle, maximizing energy transfer. After passing through one stage of impulse blades, the fluid may move to additional stages, creating a multi-stage process that increases efficiency.
Reaction Blades:
- Reaction blades use the fluid’s pressure to create a pushing force across the blade surface, producing rotation. As the fluid flows over the blade, it undergoes a drop in pressure, which generates a reaction force that pushes the blade forward.
- Reaction blades are often found in steam and hydroelectric turbines where pressure changes can be utilized to generate motion. These blades are typically longer and have a gradual curve to guide the fluid smoothly over their surfaces, allowing for continuous rotation.
- A distinguishing feature of reaction blades is that the fluid’s pressure drops as it moves through each stage, enabling efficient energy transfer at various pressure levels across multiple stages.
Blade Usage Across Different Turbine Types:
- Steam Turbines: Both impulse and reaction blades are used in steam turbines, often within multi-stage systems where pressure levels vary as the steam moves through each stage.
- Gas Turbines: Blades in gas turbines are similar to reaction blades, capturing energy from high-temperature combustion gases and relying on pressure gradients.
- Hydroelectric Turbines: Hydroelectric blades are typically reaction-type blades, as they leverage pressure differences created by flowing water. These blades are often large and adapted to capture energy efficiently in lower-speed, high-flow environments.
- Wind Turbines: Wind turbine blades are neither strictly impulse nor reaction but rather use lift created by aerodynamic forces. These blades are shaped to maximize wind energy capture by creating lift that rotates the rotor.
The specific blade type used in each turbine is determined by the fluid’s characteristics and the operational needs of the power plant, allowing for optimal energy capture and conversion.
3. Aerodynamic Design and Blade Angle
Aerodynamic design is fundamental to turbine blade efficiency, as the shape, angle, and surface of each blade impact how fluid flows over and interacts with it. These design factors help reduce drag, maximize lift, and enhance energy conversion.
Aerodynamics of Turbine Blades:
- Turbine blades are shaped to capture fluid flow efficiently, converting the energy into mechanical rotation. Blade shapes vary but are generally curved and angled to produce minimal drag and maximal lift, creating the conditions for efficient energy transfer.
- Lift and Drag: In wind turbines, blade shapes are designed to create lift, similar to airplane wings, by having a curved upper surface and a flatter lower surface. This shape causes a difference in air pressure on either side of the blade, generating lift and minimizing drag, which allows the rotor to spin with maximum efficiency.
- Minimizing Turbulence: Turbulence reduces efficiency by disrupting fluid flow. Blade designs incorporate smooth, aerodynamic surfaces to promote laminar flow, which is smoother and less turbulent. This is especially critical in gas turbines, where high-speed gases must move smoothly across blades to avoid energy loss.
Blade Angle and Pitch Control in Wind Turbines:
- In wind turbines, blade pitch control allows the angle of the blade to adjust based on wind speed. When wind speeds are low, the blades are angled to capture maximum wind energy. At high wind speeds, they pitch to a safer angle to avoid overspeeding and damage.
- Blade angle control is key to optimizing energy capture, as it allows wind turbines to adapt to variable wind conditions. By maintaining the optimal angle, pitch control ensures the turbine operates efficiently while protecting it from excessive stress.
Blade Staging and Efficiency Optimization in Steam and Gas Turbines:
- In steam and gas turbines, multiple rows or stages of blades capture energy as the fluid moves through the turbine. Each stage of blades (both impulse and reaction) is positioned at an angle that corresponds to the fluid’s velocity and pressure.
- Multi-stage turbines optimize efficiency by capturing energy at various fluid pressure levels. This arrangement ensures that energy from high-pressure fluids is fully utilized in the first stages, while lower-pressure fluid energy is captured in subsequent stages.
- Angle Optimization: Blade angles are adjusted for each stage to ensure that fluid flow strikes the blades at an optimal angle, minimizing energy loss. These angles are determined through aerodynamic modeling, which simulates fluid flow and optimizes blade positioning for maximum efficiency.
Computational Fluid Dynamics (CFD) in Blade Design:
- CFD simulations are integral to modern blade design, as they allow engineers to model how fluid will interact with different blade shapes, angles, and curvatures. CFD can simulate complex flow dynamics, providing insight into pressure distribution, drag, and potential areas of turbulence.
- CFD is used to design blade profiles that capture the maximum possible energy while minimizing energy losses. This process allows for custom blade designs tailored to specific turbine types and operational requirements.
- By using CFD, engineers can also predict performance under different operating conditions, such as variable wind speeds in wind turbines or fluctuating gas temperatures in gas turbines, allowing for adaptive blade designs that enhance overall efficiency.
The aerodynamic design of turbine blades is thus a complex and highly technical aspect of turbine engineering. Proper blade design maximizes energy capture, reduces turbulence, and ensures smooth fluid flow, all of which contribute to the turbine’s efficiency and durability.
4. Materials and Coatings
Turbine blades must be made from materials that can withstand high temperatures, pressure, and potential erosion. The choice of material impacts the blade’s efficiency, lifespan, and ability to perform under demanding conditions.
Common Materials Used in Turbine Blades:
- Nickel-Based Alloys: These alloys are common in gas turbine blades due to their ability to withstand high temperatures. Nickel alloys maintain structural integrity even at temperatures exceeding 1,500°C, making them ideal for high-stress environments.
- Ceramics and Ceramic Composites: Used in advanced gas turbines, ceramic materials provide excellent heat resistance and are often used in high-temperature regions to reduce the need for cooling systems.
- Steel and Stainless Steel: In steam and hydroelectric turbines, stainless steel offers corrosion resistance and durability at lower costs compared to alloys. This material is particularly suitable for environments exposed to moisture, such as hydroelectric plants.
- Composites: Composite materials, including carbon fiber composites, are commonly used in wind turbine blades to reduce weight and improve flexibility. These materials are strong yet lightweight, making them suitable for large-scale blades that need to be both durable and aerodynamic.
Protective Coatings:
- Blades are often coated to protect against erosion, corrosion, and thermal stress. Thermal Barrier Coatings (TBCs), for example, are used on gas turbine blades to prevent overheating by insulating the blade surface.
- Anti-Corrosion Coatings: In steam and hydroelectric turbines, coatings protect against corrosion caused by water or steam. These coatings enhance the durability of steel and stainless steel blades, preventing rust and material degradation.
- Erosion-Resistant Coatings: Blades, especially those in wind and hydroelectric turbines, face erosion from particles in the fluid (air or water). Erosion-resistant coatings are applied to extend the lifespan of the blades by minimizing wear.
By choosing materials and coatings suited to the operational environment, turbine blades can maintain high performance, resist wear, and reduce maintenance needs, which is critical for long-term turbine efficiency.
5. Durability and Structural Strength
Turbine blades operate under high-stress conditions, making durability and structural strength essential. Blades must withstand significant forces from high-speed fluid impacts, as well as thermal and mechanical stresses.
Engineering for Strength and Durability:
- Blades are engineered to handle cyclic loads—repeated stresses from continuous rotation and fluid impact. This requires materials with high fatigue resistance to prevent cracks or fractures over time.
- Structural strength is reinforced by selecting materials with excellent tensile strength and hardness, which prevent deformation under pressure. Advanced manufacturing processes like forging and heat treatment help strengthen the blade structure.
Fatigue Resistance and Cavitation Prevention:
- Fatigue resistance is crucial in high-speed turbines, where the repetitive nature of rotation can cause stress fractures. Blade materials and designs are selected to minimize stress concentration points, which are prone to crack initiation.
- In hydroelectric turbines, cavitation (the formation of vapor bubbles due to pressure differences) can damage blades. Blades are engineered to reduce cavitation by managing pressure distribution, and coatings help resist cavitation erosion.
The durability of turbine blades is essential for reliable performance and minimal downtime, ensuring turbines operate efficiently for extended periods.
6. Maintenance and Common Blade Issues
Regular maintenance is vital for turbine blades, as they are subject to wear, corrosion, and mechanical stress.
Common Blade Issues:
- Wear and Erosion: Wind and hydroelectric turbine blades face erosion from particles in the fluid, causing gradual wear. Regular inspection and recoating help extend blade life.
- Corrosion: Steam and hydroelectric turbine blades can corrode over time due to moisture exposure. Anti-corrosion coatings and periodic inspections help mitigate this issue.
- Thermal Fatigue: Gas turbine blades face thermal fatigue due to temperature fluctuations. Monitoring and replacing blades as they approach their thermal fatigue limit is essential for gas turbines.
Maintenance Practices:
- Predictive Maintenance: Sensors monitor temperature, vibration, and pressure on blades, identifying early signs of wear or failure. This enables predictive maintenance, reducing downtime and avoiding unexpected failures.
- Blade Repair and Replacement: Damaged blades are often repaired or replaced, especially in high-performance turbines. Regular servicing and part replacement prevent efficiency loss and improve turbine longevity.
Effective maintenance ensures that blades perform optimally, maximizing the turbine’s efficiency and extending its operational life.
Introduction to Casing and Housing
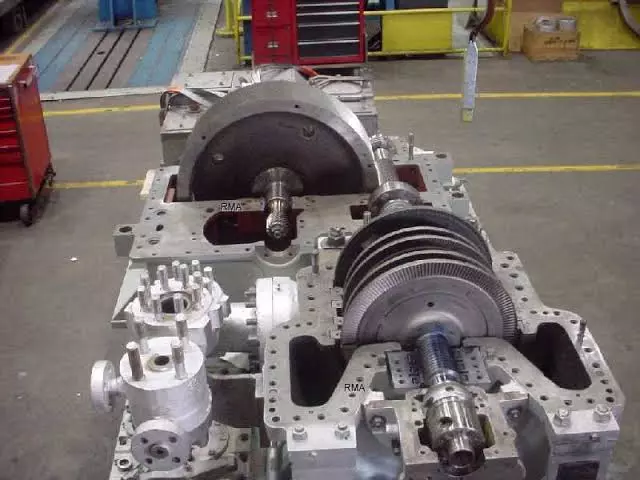
The casing and housing in turbines are essential structural components that enclose and protect the internal parts of the turbine. These outer shells serve multiple functions, from controlling the flow of the working fluid to maintaining optimal pressure levels, dissipating heat, and providing an insulated, safe environment for the rotor, blades, and shaft. The casing and housing effectively contain the intense forces generated by high-speed fluid flow, ensuring the turbine’s reliable and efficient operation.
In addition to providing structural support, turbine casings prevent fluid or gas leaks, control fluid flow, and withstand extreme temperatures and pressures. By maintaining a consistent environment, the casing and housing support the turbine’s performance and protect it from environmental damage, which is especially important in large-scale power generation applications like steam, gas, hydroelectric, and wind turbines.
Different turbine types require specific casing and housing designs due to varying fluid characteristics and operating conditions. For instance, gas turbines must withstand high temperatures from combustion gases, while hydroelectric turbines require corrosion-resistant casings to handle moisture exposure. In all cases, the casing and housing serve as vital elements that protect the turbine’s internal components, ensure safe operation, and contribute to overall efficiency.
2. Functions of Casing and Housing
The casing and housing perform several crucial functions that support the efficient, safe, and durable operation of turbines. These functions go beyond containment and play a role in directing fluid flow, maintaining system pressure, and protecting internal components.
Containment of Fluid Flow:
- One of the primary functions of the turbine casing is to contain the high-pressure, high-speed fluid within the turbine, ensuring it flows through the intended path over the blades. Proper containment of fluid prevents leaks, allowing maximum energy to be transferred to the turbine blades and enhancing overall efficiency.
- Casings have carefully designed channels and internal pathways that direct the fluid flow from entry to exit. This controlled flow is essential in steam and gas turbines, where the pressurized fluid must remain confined within specific regions to optimize energy transfer.
Maintenance of Pressure:
- Turbine casings are built to withstand high pressures, which is particularly critical in steam and gas turbines, where the fluid enters the turbine at extremely high pressures. The casing ensures that this pressure is contained without leaking or losing energy, allowing efficient energy transfer to the blades.
- In steam turbines, casings are designed to handle the high pressure of steam at multiple stages, maintaining pressure levels as the steam expands. Similarly, in gas turbines, the casing maintains pressure during combustion, enabling efficient energy generation.
Protection of Internal Components:
- Casing and housing provide a protective barrier that shields internal components from environmental hazards such as dust, moisture, and temperature fluctuations. For example, in hydroelectric turbines, the casing is made of corrosion-resistant materials to withstand prolonged exposure to water.
- In wind turbines, the casing protects the internal components from debris, wind gusts, and changing weather conditions. This protective function is crucial for the turbine’s longevity, ensuring that internal components such as blades, rotors, and shafts remain in optimal condition.
Impact on Turbine Efficiency and Safety:
- By maintaining consistent pressure and fluid flow, the casing contributes directly to the turbine’s energy conversion efficiency. Any leaks or variations in flow would reduce the energy captured by the blades, decreasing the turbine’s overall output.
- Additionally, the casing provides a structural framework that stabilizes the turbine, supporting safe operation even under extreme conditions. This stability helps prevent operational failures, protecting both the turbine and personnel in the vicinity.
The functions of the casing and housing ensure that the turbine operates safely, efficiently, and reliably, supporting the turbine’s central role in power generation.
3. Design and Structure of Casing and Housing
The design of the casing and housing varies significantly depending on the turbine type and the specific operating conditions it must endure. The structure must balance the need for strength, thermal and pressure resistance, and efficiency in fluid flow management.
Structural Design for Different Turbine Types:
- Steam Turbine Casings: Steam turbines require casings that can handle high pressures and elevated temperatures. The casing is typically multi-layered, with an insulated inner layer to manage heat and prevent thermal expansion. Steam turbine casings often contain multiple chambers for different pressure stages, enabling efficient energy extraction as steam expands and moves through the turbine.
- Gas Turbine Casings: Gas turbines operate at extremely high temperatures due to combustion. Casings are designed with cooling channels and heat-resistant materials to protect the structure from thermal stress. The gas turbine casing also contains the combustion chamber, where fuel is ignited and high-pressure gases are generated, making strength and thermal resistance paramount.
- Hydroelectric Turbine Casings: In hydroelectric turbines, casings are large and often cylindrical, built to contain the slower-moving but high-force water flow. These casings are made of corrosion-resistant materials to prevent rusting and erosion, as they are constantly exposed to water. Additionally, hydroelectric casings are designed to minimize cavitation, which can damage turbine blades.
- Wind Turbine Housings: Wind turbines are unique in that they do not deal with high pressure or temperature. Instead, their casings (known as nacelles) are designed to protect the internal components from weather, wind, and debris. Nacelles also provide access points for maintenance and contain yaw systems to adjust the turbine’s orientation relative to the wind.
Features for Handling High Pressure and Temperature:
- High-pressure fluid management is a central feature of steam and gas turbine casings, where even small leaks can lead to significant energy loss. Casings are designed with seals that prevent leakage and maintain pressure within the turbine.
- Thermal insulation is applied to inner surfaces to prevent excessive heat loss and manage thermal expansion. In gas turbines, cooling systems integrated into the casing help maintain a stable internal temperature, protecting internal components from thermal damage.
Flow Channels, Seals, and Insulation:
- Flow channels within the casing guide the fluid through the turbine, ensuring it impacts the blades at the optimal angle and pressure. These channels must be smooth to minimize turbulence, which could decrease efficiency and cause wear on the casing.
- High-quality seals are installed at casing joints to prevent fluid escape. In steam and gas turbines, these seals are typically made from materials that can withstand high pressure and temperature.
- Insulation inside the casing controls thermal expansion and prevents excessive heat from damaging the structure. Insulation is essential in steam and gas turbines, where high-temperature fluids can cause expansion and stress within the casing.
By combining these structural features, turbine casings and housings effectively support the turbine’s operational requirements, ensuring durability, efficiency, and safety.
4. Materials for Casing and Housing
The choice of materials for turbine casing and housing is crucial, as it must withstand various environmental and operational challenges, including high pressure, heat, corrosion, and mechanical stress. Selecting the appropriate material directly impacts the turbine’s efficiency and longevity.
Common Materials Used:
- High-Strength Alloys: High-strength alloys, such as stainless steel and nickel-based alloys, are commonly used in gas and steam turbine casings. These alloys offer high thermal stability, resist corrosion, and can handle high-pressure conditions without deformation.
- Composites: Some casings, especially those in wind turbines, use composite materials for lightweight durability. These materials are strong and resistant to weathering, which makes them ideal for turbines exposed to variable environmental conditions.
- Carbon Steel: For hydroelectric turbines, carbon steel is frequently used due to its corrosion resistance and ability to handle high water pressures. Carbon steel is also economical, making it a practical choice for the large casings required in hydroelectric plants.
Temperature and Corrosion Resistance:
- Casings must withstand high operating temperatures, particularly in gas turbines where combustion generates intense heat. Nickel-based alloys and ceramics are used in high-temperature regions of gas turbine casings to prevent warping or melting.
- Corrosion resistance is equally critical, especially in hydroelectric and steam turbines where moisture can lead to rusting. Stainless steel and anti-corrosive coatings are applied to prevent deterioration, ensuring the casing’s longevity.
Material Considerations for Different Environments:
- In gas turbines, materials must withstand thermal cycling caused by repeated heating and cooling, requiring alloys that resist thermal fatigue.
- In steam turbines, materials must handle high-pressure steam while avoiding corrosion, leading to the use of stainless steel and corrosion-resistant alloys.
- For hydroelectric turbines, materials must resist both corrosion and erosion, making materials like carbon steel and ceramic coatings ideal for protecting the casing from water damage.
Selecting the right materials for turbine casings ensures that they can withstand operational stress, maintain structural integrity, and support long-term turbine efficiency.
5. Thermal and Pressure Resistance
Casing and housing components in turbines are exposed to high pressures and temperatures, especially in gas and steam turbines. Effective thermal and pressure resistance is necessary to maintain stability, prevent leaks, and support turbine efficiency.
Managing Thermal Expansion and Contraction:
- High temperatures cause casing materials to expand, which can lead to structural stress if not managed properly. Thermal insulation is applied to inner surfaces to reduce heat transfer, while cooling channels in gas turbines help control temperature levels.
- Some casings are built with expansion joints to allow for controlled thermal movement, preventing cracks and other damage from thermal stress.
Pressure Resistance:
- The casing must withstand immense pressures, particularly in steam and gas turbines. High-strength seals and reinforced joints help the casing resist pressure-related stress. In steam turbines, where fluid pressure changes across stages, casings are designed to maintain pressure differentials without leaking.
- In hydroelectric turbines, casings manage the continuous pressure of water flow. The casing design incorporates smooth flow channels to prevent cavitation, a condition where bubbles form and collapse, potentially causing damage.
Heat Dissipation Techniques:
- Gas turbine casings incorporate cooling systems to dissipate heat from combustion, while insulation in steam turbines limits heat loss. In both cases, heat management prevents overheating and protects internal components.
- Effective heat dissipation reduces the risk of thermal fatigue, which can weaken materials over time and lead to casing failure.
Thermal and pressure resistance are crucial for casings to support long-term operation under extreme conditions, helping maintain the turbine’s performance and structural stability.
6. Maintenance and Operational Challenges
Regular maintenance of turbine casings and housings is necessary to prevent operational issues and extend the turbine’s lifespan. These components are subject to wear, corrosion, and thermal stress, which require ongoing monitoring and repair.
Common Issues:
- Thermal Fatigue: Repeated heating and cooling cycles can cause thermal fatigue, leading to small cracks or deformations in the casing. Monitoring thermal stress and performing regular inspections can help detect issues early.
- Corrosion: Exposure to moisture in steam and hydroelectric turbines can lead to corrosion, especially in casings without sufficient anti-corrosive coatings. Regular application of protective coatings helps combat corrosion.
- Wear and Tear: Continuous pressure and fluid flow cause wear on the casing’s inner surfaces. Inspecting for signs of erosion and implementing protective linings can prevent further damage.
Maintenance Practices:
- Predictive Maintenance: Using sensors to monitor temperature, pressure, and vibration can help identify potential issues before they lead to failure, reducing the need for unplanned downtime.
- Regular Inspection and Repair: Scheduled inspections allow maintenance teams to address small issues before they become major problems, extending the casing’s lifespan and ensuring operational efficiency.
By conducting regular maintenance, operators ensure that the casing and housing components remain structurally sound, supporting the turbine’s performance and reducing the risk of costly repairs.
Introduction to Nozzles and Guide Vanes
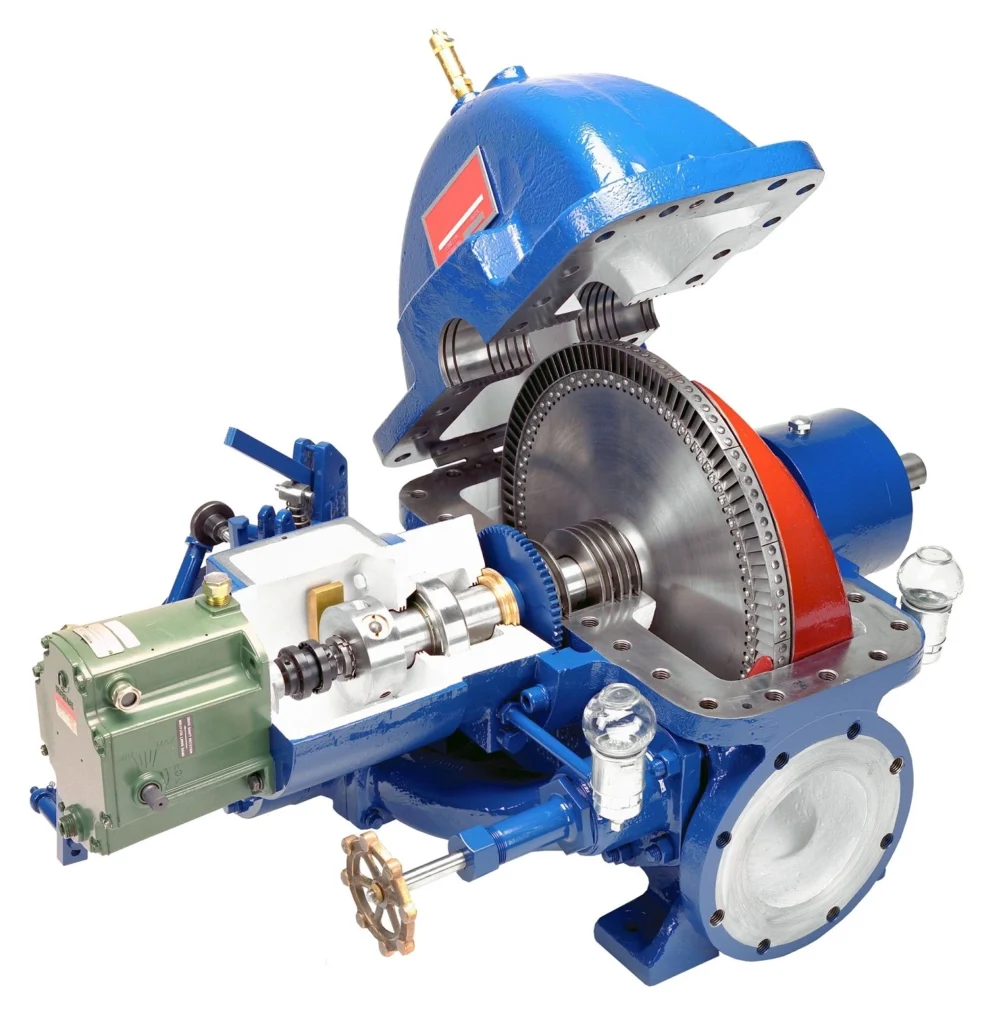
Nozzles and guide vanes are essential components in turbines that direct the flow of fluid—whether it is steam, gas, water, or air—through the turbine. Their primary function is to control the angle, speed, and pressure at which fluid interacts with the turbine blades. By managing the fluid’s characteristics as it enters and moves through the turbine, nozzles and guide vanes help maximize energy transfer to the rotor, improve efficiency, and maintain stable operating conditions.
The design, positioning, and material selection of nozzles and guide vanes vary based on the type of turbine and the working fluid’s properties. In impulse turbines, nozzles play a key role by converting high-pressure fluid into high-speed jets that strike the blades directly, transferring kinetic energy. In reaction turbines, guide vanes are used to direct the fluid smoothly over the blades, creating pressure changes that drive rotation. Similarly, in hydroelectric and wind turbines, guide vanes adjust the flow to optimize energy capture from water or wind.
Due to their critical role in directing fluid flow, nozzles and guide vanes are highly engineered for durability and precision, as they must withstand the high stresses and temperatures present within turbine systems. Proper design and maintenance of these components are essential for efficient and reliable turbine operation.
2. Function of Nozzles and Guide Vanes
Nozzles and guide vanes perform the critical function of shaping and directing the fluid flow within a turbine to maximize energy capture. Their primary purpose is to manage the fluid’s speed, pressure, and direction so that it strikes the turbine blades in an optimal manner.
Fluid Direction and Speed Control:
- Nozzles accelerate fluid by narrowing the flow path, converting pressure energy into kinetic energy. This is particularly important in impulse turbines, where the fluid (usually steam or gas) must reach high speeds to impart maximum kinetic energy to the blades. By controlling fluid speed, nozzles ensure that the fluid hits the blades with enough force to drive the rotor efficiently.
- Guide vanes, on the other hand, are used to direct the fluid smoothly onto the blades, especially in reaction turbines where fluid pressure changes across the blade surface drive rotation. Guide vanes help control the fluid’s angle and flow direction, ensuring consistent pressure and velocity as the fluid moves through the turbine.
Role in Impulse and Reaction Turbines:
- In impulse turbines, nozzles play the most important role. As the fluid exits the nozzle at high velocity, it strikes the impulse blades directly, transferring kinetic energy and causing the rotor to spin. The nozzles must be precisely angled to direct the fluid flow accurately, maintaining high efficiency.
- In reaction turbines, guide vanes are essential. They manage the fluid’s pressure and velocity as it moves across the blades, creating a pressure differential that drives rotation. This design allows reaction turbines to capture energy more gradually across multiple stages, enhancing efficiency at varying pressure levels.
Effect on Pressure, Velocity, and Energy Transfer:
- Nozzles convert high-pressure fluid into high-speed jets by narrowing the flow path, resulting in a dramatic increase in fluid velocity and a corresponding decrease in pressure. This process ensures that the maximum amount of kinetic energy is available to strike the blades.
- Guide vanes control the fluid’s direction, stabilizing pressure and velocity for efficient energy transfer. By managing fluid flow dynamics, guide vanes help maintain a stable environment within the turbine, reducing the potential for turbulence and inefficiencies.
The combined roles of nozzles and guide vanes ensure that the fluid flows through the turbine efficiently, maximizing energy capture and supporting smooth, consistent operation.
3. Types of Nozzles and Guide Vanes
There are various types of nozzles and guide vanes, each designed for specific applications and turbine types. Understanding these variations helps in appreciating how they enhance the functionality and efficiency of turbines.
Fixed and Variable Nozzles:
- Fixed Nozzles: These are stationary and provide a consistent flow rate and angle. Fixed nozzles are often used in impulse turbines where the fluid enters at a stable, high pressure, and consistency is key to maintaining optimal efficiency.
- Variable Nozzles: Variable nozzles can adjust the angle and flow rate of the fluid, making them ideal for turbines with fluctuating loads or variable fluid conditions. For example, in gas turbines, variable nozzles allow for adjustments in air and fuel flow to respond to changes in power demand, enhancing operational flexibility.
Guide Vanes for Different Turbines:
- Adjustable Guide Vanes: In hydroelectric turbines, guide vanes are often adjustable to optimize water flow based on water level and load conditions. These vanes change the flow direction and volume, helping hydroelectric turbines respond to fluctuating water availability and power demand.
- Fixed Guide Vanes: In wind turbines, fixed guide vanes (or stators) are used to stabilize airflow, ensuring that wind enters the blades at a controlled angle. Fixed guide vanes are also used in some reaction steam turbines, where consistent fluid direction is essential for smooth operation across multiple stages.
Comparison of Nozzle and Guide Vane Usage Across Turbine Types:
- Steam Turbines: In steam turbines, both fixed nozzles (for impulse stages) and adjustable guide vanes (for reaction stages) are used to manage steam flow and pressure. These components work together to maximize energy transfer across various pressure stages.
- Gas Turbines: Gas turbines often use variable nozzles to control airflow into the combustion chamber, optimizing fuel combustion and ensuring that exhaust gases hit the turbine blades at an efficient angle and speed.
- Hydroelectric Turbines: Adjustable guide vanes are crucial in hydroelectric turbines, as they allow operators to control water flow based on reservoir levels, turbine load, and environmental factors.
- Wind Turbines: Although wind turbines do not use nozzles in the traditional sense, they do incorporate fixed guide vanes (or stators) to help streamline airflow. This maintains consistent wind flow direction onto the blades, optimizing energy capture.
Different types of nozzles and guide vanes provide versatility in fluid flow control, helping turbines operate efficiently under various conditions.
4. Design and Aerodynamics of Nozzles and Guide Vanes
The design of nozzles and guide vanes is highly specific, as it directly affects the efficiency and performance of the turbine. Aerodynamics plays a critical role in minimizing drag, optimizing fluid flow, and reducing turbulence.
Optimal Shape and Angle for Fluid Flow Control:
- Nozzles are typically designed with a narrowing profile that accelerates the fluid as it exits. This nozzle shape is essential in impulse turbines, where converting pressure energy into kinetic energy is the primary goal. The shape ensures that fluid exits at high speed, maintaining the desired pressure and velocity for energy transfer.
- Guide vanes are often shaped with gentle curves to guide the fluid smoothly over the blades, minimizing turbulence. The exact angle of the vanes is crucial, as it determines how efficiently the fluid transfers its energy to the turbine blades. In adjustable guide vanes, this angle can be changed based on load and fluid flow requirements.
Aerodynamic Considerations in Design to Reduce Drag and Turbulence:
- Drag and turbulence reduce efficiency by causing energy loss, so minimizing these forces is essential. Nozzles and guide vanes are designed with aerodynamically optimized surfaces that promote laminar (smooth) flow, reducing drag and allowing the fluid to move with minimal resistance.
- In high-speed turbines, such as gas turbines, even slight surface roughness on nozzles or guide vanes can lead to significant energy loss. Therefore, these components are typically machined to have very smooth surfaces, minimizing drag and preventing boundary layer separation, which can cause turbulence.
Computational Fluid Dynamics (CFD) in Nozzle and Vane Design:
- CFD simulations are widely used in the design of nozzles and guide vanes to analyze fluid flow and optimize efficiency. CFD allows engineers to model various flow conditions, angles, and shapes, providing a precise understanding of how fluid will behave as it passes through the nozzle or vane.
- CFD modeling helps engineers identify areas of high drag, turbulence, or energy loss, allowing them to adjust designs accordingly. By testing different shapes, angles, and surface textures virtually, designers can create nozzles and vanes that maximize energy transfer while minimizing resistance.
- CFD is also valuable in optimizing the positioning of guide vanes in multi-stage turbines, ensuring that each stage receives fluid at the right angle and velocity to maintain efficiency across the entire turbine.
The aerodynamic design of nozzles and guide vanes is thus a highly technical process that combines precision engineering and advanced simulations to create components that facilitate smooth, efficient fluid flow.
5. Materials and Durability
Materials for nozzles and guide vanes must withstand high pressures, temperatures, and in some cases, corrosive environments. Selecting the right material is essential for ensuring durability and minimizing maintenance requirements.
Materials Used in High-Temperature and High-Pressure Environments:
- Nickel-Based Alloys: In gas turbines, where nozzles are exposed to extreme temperatures, nickel-based alloys are commonly used due to their high melting points and excellent thermal stability. These alloys maintain structural integrity even under intense heat.
- Stainless Steel: Stainless steel is often used in steam and hydroelectric turbines for guide vanes and nozzles, providing corrosion resistance and strength. Stainless steel is particularly useful in water-based environments where corrosion resistance is essential.
Corrosion-Resistant Coatings for Durability:
- Many nozzles and guide vanes are coated with corrosion-resistant materials to extend their operational lifespan. In hydroelectric turbines, where guide vanes are exposed to water, coatings protect against rust and water-related erosion.
- Thermal Barrier Coatings (TBCs) are applied to nozzles in gas turbines to protect against extreme heat and prevent material degradation.
Material Differences in Different Types of Turbines:
- Steam turbines require materials that can handle both high temperature and moisture. In such cases, heat-resistant stainless steel alloys are used to resist both thermal and moisture-related stress.
- In wind turbines, where temperatures and pressures are lower, lightweight and corrosion-resistant materials like composites may be used for guide vanes to minimize weight and wear.
By using materials tailored to each turbine’s operating conditions, nozzles and guide vanes achieve the durability required to operate efficiently over extended periods.
6. Maintenance and Operational Challenges
Maintenance of nozzles and guide vanes is critical, as these components are prone to wear, erosion, and other operational challenges.
Common Issues:
- Erosion: High-speed fluids can cause erosion on the surfaces of nozzles and guide vanes, particularly in steam and gas turbines where particle-laden fluids can abrade surfaces.
- Corrosion: Exposure to water in hydroelectric turbines or to humid steam in steam turbines can lead to corrosion. Protective coatings and regular inspection help prevent corrosion-related damage.
- Thermal Fatigue: In gas turbines, nozzles and guide vanes are subject to thermal cycling, which can lead to material fatigue over time. This makes inspection and timely replacement important to avoid performance issues.
Maintenance Practices:
- Predictive Maintenance: Sensors monitor temperature, vibration, and wear on nozzles and guide vanes, allowing operators to detect issues before they escalate. This proactive approach minimizes unplanned downtime.
- Inspection and Replacement: Regular inspections help identify erosion, cracks, or deformation. Replacement of worn nozzles and guide vanes is a key maintenance step in extending turbine lifespan.
Effective maintenance of nozzles and guide vanes ensures that they continue to function optimally, supporting turbine efficiency and reducing operational disruptions.
Introduction to Cooling and Lubrication Systems
In turbines, cooling and lubrication systems are essential for maintaining operational stability, preventing overheating, and minimizing friction among rotating components. The turbine’s high-speed operation generates substantial heat and mechanical stress, especially in steam and gas turbines, where temperatures can reach over 1,500°C in the combustion zones. Proper cooling and lubrication prevent thermal expansion, excessive wear, and material degradation, all of which can reduce turbine efficiency and lifespan.
Cooling systems protect critical turbine parts, including blades, rotors, and casing, from thermal stress by dissipating excess heat. Lubrication systems, on the other hand, reduce friction between moving parts such as bearings, shafts, and gearboxes, preventing mechanical wear and heat buildup. Together, these systems ensure that turbines operate smoothly, efficiently, and reliably over prolonged periods.
Given the diversity of turbine types—steam, gas, hydroelectric, and wind—cooling and lubrication systems are tailored to suit specific operational needs. While gas turbines may require advanced air and oil cooling due to extreme temperatures, hydroelectric and wind turbines may rely on simpler cooling methods. Effective cooling and lubrication systems are therefore integral to turbine performance, safeguarding against failures and supporting efficiency in power generation.
2. Functions and Importance of Cooling Systems
Cooling systems in turbines play a critical role in maintaining temperature stability and protecting components from overheating. Turbines generate considerable heat as they operate at high speeds, with fluid flowing over blades and through various chambers. Efficient cooling systems ensure that the turbine remains within safe operating temperatures, preventing damage and optimizing performance.
Temperature Management:
- Cooling systems manage the heat generated by high-speed rotation and fluid friction within the turbine. In gas turbines, where combustion produces extreme temperatures, cooling is especially important for preventing blade and rotor deformation.
- Cooling systems stabilize the temperature across the turbine’s components, preventing thermal gradients that could lead to cracking, warping, or expansion.
Preventing Thermal Expansion and Material Degradation:
- High temperatures can cause materials to expand and degrade, leading to a reduction in structural integrity. Cooling systems prevent such thermal expansion, maintaining material stability and ensuring the turbine’s components fit precisely together.
- Thermal fatigue occurs when turbine materials are exposed to repeated heating and cooling cycles, which can lead to cracks. By reducing temperature fluctuations, cooling systems extend the turbine’s lifespan and reduce maintenance needs.
Cooling Requirements for Different Turbine Types:
- Gas Turbines: Due to combustion processes, gas turbines have the most demanding cooling requirements. They often rely on advanced air and oil cooling systems to manage high temperatures. Cooling channels within blades, often combined with thermal barrier coatings, keep blades cool.
- Steam Turbines: Steam turbines operate at high temperatures and pressures, requiring efficient cooling to maintain stable operation. While they do not reach the temperatures of gas turbines, they still rely on water or air cooling to prevent material degradation.
- Hydroelectric and Wind Turbines: These turbines typically generate less heat and, as a result, have simpler cooling requirements. Natural airflow is sometimes sufficient for wind turbines, while water cooling is inherent in hydroelectric setups due to the presence of water as the working fluid.
Effective cooling systems are crucial for all turbine types, as they maintain temperature control, reduce wear, and enable longer operational periods without failure.
3. Types of Cooling Systems in Turbines
There are several types of cooling systems used in turbines, each chosen based on the turbine type, operating temperature, and the specific demands of the power plant. These systems include air, water, oil, and hybrid cooling methods, each suited to different operating conditions.
Air Cooling:
- Air cooling is a common choice for cooling turbine casings, rotors, and stators. In air-cooled systems, fans or natural airflow are used to dissipate heat generated by the turbine. This cooling method is simple, cost-effective, and is commonly used in wind turbines and smaller industrial gas turbines.
- Air cooling can be less effective in high-temperature zones, as air has a lower heat capacity than other cooling media. For gas turbines, air cooling is often used in combination with internal cooling channels within the blades.
Water Cooling:
- Water cooling is widely used in steam turbines, where high temperatures and pressures necessitate efficient heat dissipation. Water absorbs heat efficiently and has a high specific heat capacity, making it an ideal medium for cooling turbine components.
- In steam turbines, water cooling systems circulate water through the turbine casing or around critical components. Cooling towers are often used to cool the water after it has absorbed heat, ensuring that the system operates effectively and sustainably.
- Water cooling is especially effective in hydroelectric turbines, where the surrounding water can absorb excess heat naturally. This is both efficient and environmentally friendly, as the water is readily available and the process has minimal environmental impact.
Oil Cooling:
- Oil cooling is frequently used in gas turbines, particularly for lubricating and cooling high-temperature components such as bearings and gear systems. Oil has excellent heat dissipation properties and can circulate through the system to absorb heat from critical areas.
- Oil cooling is often integrated with lubrication systems, enabling a combined approach to reduce friction and manage temperature. In gas turbines, high-performance synthetic oils with high thermal stability are used, as they can withstand extreme temperatures without breaking down.
- In cases where additional cooling is needed, oil-to-water heat exchangers are sometimes used. These devices transfer heat from the oil to circulating water, providing a secondary level of cooling and maintaining a stable operating temperature.
Hybrid Cooling Systems:
- Hybrid cooling systems combine multiple cooling methods for enhanced temperature control, often used in large-scale gas turbines where single cooling methods are insufficient. For example, a hybrid system might use air cooling for casings, oil cooling for bearings, and water cooling for specific high-temperature zones.
- These systems are designed to maximize efficiency by addressing the unique cooling needs of different components. Hybrid systems are common in combined cycle gas plants, where gas and steam turbines work together, creating complex cooling requirements.
Each cooling method has its advantages and is selected based on the turbine’s operational demands. By effectively managing temperature, these systems support the longevity, stability, and efficiency of turbine components under varying conditions.
4. Functions and Importance of Lubrication Systems
Lubrication systems in turbines are essential for reducing friction between moving parts, preventing wear, and ensuring smooth operation. Lubrication also plays a role in dissipating heat generated from high-speed rotations, protecting critical components from overheating.
Reducing Friction and Preventing Wear:
- In a turbine, numerous components—including bearings, shafts, and gear systems—move at high speeds and are exposed to continuous mechanical stress. Without lubrication, these parts would suffer from rapid wear, leading to increased maintenance and shorter operational lifespans.
- Lubrication forms a protective layer between metal surfaces, reducing direct contact and minimizing friction. This helps prevent material degradation, reduces energy losses from friction, and enhances turbine efficiency.
Heat Dissipation:
- In addition to reducing friction, lubrication aids in heat dissipation. Friction generates heat, which, if not managed, can damage components or cause overheating. Circulating lubricants absorb this heat and transfer it away from critical areas.
- For example, in gas turbines, lubrication oil circulates through bearings and other high-friction parts, absorbing and dissipating heat to maintain stable operating temperatures.
Smooth Operation Under High-Load Conditions:
- Turbines often operate under varying load conditions, and lubrication systems ensure consistent performance across these conditions. By maintaining a constant lubrication film, the system supports smooth, uninterrupted rotation, which is especially important during peak load periods.
- Effective lubrication extends the life of moving parts and minimizes the risk of sudden mechanical failures, contributing to safe and reliable turbine operation.
Lubrication is therefore fundamental to turbine functionality, as it not only prevents wear but also assists in cooling, supporting both efficiency and durability.
5. Types of Lubrication Systems in Turbines
There are several types of lubrication systems used in turbines, each tailored to specific operational needs and component characteristics. These systems include forced lubrication, mist lubrication, grease lubrication, and advanced self-lubricating bearings.
Forced Lubrication Systems:
- Forced lubrication systems use oil pumps to circulate lubricant through the turbine. This system is essential in large turbines, where high-pressure oil flow is required to maintain consistent lubrication under heavy loads.
- The oil circulates through the bearings, shafts, and other high-friction components, providing a continuous lubrication film. Cooling units are often integrated into forced lubrication systems to regulate the oil temperature, enhancing both lubrication and heat dissipation.
- Forced lubrication is common in gas and steam turbines, where it is crucial for maintaining stable operation under intense conditions.
Mist Lubrication:
- Mist lubrication creates a fine mist of lubricant that is sprayed onto components, particularly useful for small or sensitive parts that require minimal lubrication. Mist systems are often used in wind turbines or other low-load areas, as they provide precise lubrication without excess oil application.
- Mist lubrication is also beneficial in minimizing the risk of contamination, as it applies only the necessary amount of lubricant to each part.
Grease Lubrication:
- Grease lubrication is used in areas that experience lower loads or slower speeds. Grease is thicker than oil and stays in place longer, making it suitable for components that do not require frequent lubrication.
- In hydroelectric turbines, grease lubrication is sometimes used in low-speed bearings and other areas exposed to moisture, as it provides a more stable lubrication layer and resists water washout.
Self-Lubricating Bearings:
- Self-lubricating bearings are advanced components designed to release lubricant gradually, minimizing the need for external lubrication systems. These bearings contain lubricant within their structure, which is released over time as the bearing rotates.
- This type of lubrication is particularly beneficial in remote or hard-to-access turbines, such as offshore wind turbines, where regular maintenance may be challenging.
Each lubrication system is designed to suit the specific demands of turbine components, ensuring that they operate smoothly and resist wear over long periods.
6. Materials and Durability in Cooling and Lubrication Systems
The materials used in cooling and lubrication systems must withstand high temperatures, pressures, and, in some cases, corrosive environments. High-quality materials are essential for durability and long-term performance.
Cooling System Materials:
- Stainless steel and nickel alloys are often used for cooling system components exposed to high temperatures, as they resist corrosion and thermal stress.
Lubrication System Materials:
- Synthetic lubricants with high thermal stability are commonly used in gas turbines, as they maintain performance at high temperatures without breaking down.
- Advanced coatings, such as anti-corrosion and wear-resistant films, are applied to protect system components from degradation.
These materials ensure that cooling and lubrication systems can operate reliably under extreme conditions.
7. Maintenance and Operational Challenges
Maintaining cooling and lubrication systems is essential for preventing failures and ensuring turbine longevity. These systems are prone to several operational challenges that require regular monitoring and maintenance.
Common Issues:
- Clogging: Dust, debris, or particles in the fluid can clog cooling channels and lubrication pathways, reducing system efficiency. Regular filtration and cleaning help prevent clogging.
- Overheating: Inefficient cooling or lubrication can lead to overheating, risking damage to critical components. Temperature monitoring systems are often used to detect overheating and trigger cooling adjustments.
- Oil Degradation: In lubrication systems, oil can degrade over time due to thermal stress and contamination. Routine oil analysis helps identify degradation early, allowing for timely oil replacement.
Maintenance Practices:
- Predictive Maintenance: Sensors monitor temperature, pressure, and flow rates in cooling and lubrication systems, providing real-time data on system health. This enables predictive maintenance, where issues can be addressed before they cause failures.
- Routine Inspection and Replacement: Regular inspections ensure that cooling and lubrication systems function effectively. Replacing filters, oils, and worn components prevents efficiency loss and extends turbine life.
By implementing regular maintenance practices, turbine operators can prevent failures, enhance efficiency, and ensure that cooling and lubrication systems continue to support optimal turbine operation.
Introduction to Control and Monitoring Systems
Control and monitoring systems are the core of a turbine’s operational intelligence, responsible for regulating, analyzing, and optimizing the turbine’s performance. These systems enable real-time data collection, providing operators with critical insights into parameters such as speed, temperature, pressure, and vibrations. Through advanced control and monitoring, turbines operate within optimal ranges, maximizing efficiency, minimizing wear, and enhancing safety.
Modern control systems use sensors and processors to automate adjustments, allowing the turbine to adapt to changing conditions such as variations in load demand or fluid flow. Monitoring systems, meanwhile, continuously analyze turbine health, detecting any deviations from normal performance that may indicate issues like mechanical wear or thermal degradation. Together, these systems not only prevent downtime by identifying potential problems early but also extend the turbine’s lifespan through proactive management.
With advancements in digital technology, control and monitoring systems now include Supervisory Control and Data Acquisition (SCADA), Distributed Control Systems (DCS), Programmable Logic Controllers (PLC), and even artificial intelligence (AI) and the Internet of Things (IoT) for enhanced analytics. These technologies allow for greater precision, predictive maintenance, and optimized efficiency in turbine operation.
2. Functions of Control Systems
Control systems in turbines play a crucial role in managing the various operational parameters that influence the turbine’s performance and efficiency. Their primary function is to regulate the turbine’s speed, temperature, pressure, and power output, ensuring that it operates within safe and efficient limits.
Regulating Speed and Output:
- Control systems regulate the turbine’s rotational speed to match the desired output. This is essential for power plants that need to adjust production based on demand. By controlling the speed, the system ensures that the turbine maintains the correct frequency and power output.
- For example, in wind turbines, control systems adjust the blade pitch angle to control the rotor speed in response to changes in wind speed, ensuring consistent power generation while protecting the turbine from overspeeding.
Temperature and Pressure Control:
- In gas and steam turbines, control systems manage temperature and pressure to prevent overheating and material degradation. By monitoring these parameters and adjusting cooling systems or fuel input, control systems ensure stable operation.
- Pressure control is particularly important in steam turbines, where high-pressure steam expands across multiple stages. By regulating the flow of steam, control systems help maintain consistent pressure across each stage, optimizing energy conversion efficiency.
Automated Adjustments for Load Changes:
- Turbine load conditions can vary significantly depending on power demand, especially in applications like natural gas turbines that respond to peak load demands. Control systems automatically adjust fuel input, blade angles, and coolant flow to match load changes, maintaining optimal efficiency across all operating conditions.
- These automated adjustments prevent stress on turbine components, allowing the turbine to adapt to fluctuating demand without compromising on performance or safety.
Importance of Safety in Control Systems:
- Control systems include safety protocols that prevent the turbine from operating outside safe limits. In case of anomalies such as excessive speed, high temperatures, or pressure spikes, the control system can automatically trigger shut-off mechanisms to prevent damage.
- Redundant safety features within control systems ensure that, even if one system fails, backup systems can maintain control, providing an added layer of protection for both the turbine and personnel.
Through real-time regulation and automated adjustments, control systems in turbines optimize performance, respond to changing load demands, and ensure the safe operation of the turbine.
3. Functions of Monitoring Systems
Monitoring systems in turbines are responsible for continuously tracking the operational health and performance of the turbine. By gathering real-time data on critical parameters, monitoring systems provide early warning signs of potential issues and support predictive maintenance strategies.
Real-Time Data Collection:
- Monitoring systems use a network of sensors to collect data on key performance indicators, such as temperature, pressure, speed, and vibration. This real-time data allows operators to make informed decisions and respond to any deviations that may indicate problems.
- For example, in gas turbines, temperature sensors monitor the combustion chamber and turbine blade temperatures. Any sudden increase in temperature may suggest a malfunction, allowing for immediate action.
Detection of Wear, Degradation, and Anomalies:
- Monitoring systems detect early signs of mechanical wear, degradation, and material fatigue, which are common in high-stress environments like turbines. Vibration sensors, for example, track changes in vibration levels that may indicate imbalance, bearing wear, or rotor misalignment.
- Anomalies, such as unexpected temperature or pressure fluctuations, are flagged by the system for further analysis. By identifying such deviations early, monitoring systems reduce the risk of sudden failures and extend the operational life of the turbine.
Predictive Maintenance:
- Predictive maintenance relies heavily on monitoring systems. By analyzing data trends, the system can forecast potential issues before they become critical. For example, if vibration levels show a gradual increase, this might indicate bearing wear, prompting maintenance before a breakdown occurs.
- Monitoring systems support cost-effective maintenance planning, as operators can schedule interventions based on actual data rather than fixed schedules. This minimizes downtime and reduces maintenance costs.
Performance Tracking and Optimization:
- In addition to identifying issues, monitoring systems help operators track performance over time. By comparing real-time data with baseline performance metrics, operators can identify areas for improvement and adjust operational strategies to optimize efficiency.
- Performance tracking is especially useful in renewable energy applications like wind and hydroelectric turbines, where fluctuating environmental conditions require close monitoring to maximize energy capture.
Overall, monitoring systems are indispensable for maintaining the reliability, efficiency, and safety of turbines by providing continuous insights into their operational health.
4. Types of Control and Monitoring Systems
Several types of control and monitoring systems are used in turbine operations, each offering specific features suited to different applications. The primary types include Supervisory Control and Data Acquisition (SCADA), Distributed Control Systems (DCS), and Programmable Logic Controllers (PLC), along with advancements in AI and IoT for enhanced data analysis.
Supervisory Control and Data Acquisition (SCADA):
- SCADA systems are widely used in power plants to provide centralized control and monitoring capabilities. They collect data from multiple sensors and present it in a user-friendly format, allowing operators to monitor turbine performance and control settings remotely.
- SCADA systems are particularly useful in large facilities, such as wind farms, where they can monitor and control numerous turbines from a single location. By integrating real-time data, SCADA enables quick responses to any operational changes or faults.
Distributed Control Systems (DCS):
- DCS provides a decentralized approach to turbine control, where each section of the turbine has its own control unit. This system improves reliability by localizing control functions and minimizing the risk of a single point of failure.
- DCS is commonly used in gas and steam turbines where high-speed processing is required to manage temperature and pressure control. By processing data closer to the source, DCS enhances response times and improves overall system stability.
Programmable Logic Controllers (PLC):
- PLCs are compact, flexible control systems that can be programmed for specific tasks, such as managing cooling systems or regulating speed in smaller turbines. They are reliable and can operate independently or as part of a larger control system.
- PLCs are popular in industrial applications where automation is needed for particular functions. In turbines, they are often used to control auxiliary systems or backup safety mechanisms.
Integration of AI and IoT for Advanced Analytics:
- AI and IoT technologies are transforming turbine control and monitoring systems by enabling predictive analytics and remote monitoring. IoT devices collect data from sensors, while AI algorithms analyze this data to predict maintenance needs and optimize performance.
- For example, machine learning algorithms can identify subtle patterns in vibration data, detecting early signs of wear that might go unnoticed by traditional monitoring. AI-driven insights help operators make proactive decisions, reducing downtime and extending the lifespan of the turbine.
Together, these control and monitoring systems provide a comprehensive suite of tools for managing turbine performance, supporting efficient and reliable operation.
5. Components and Sensors in Control and Monitoring Systems
The effectiveness of control and monitoring systems depends on the quality and accuracy of the sensors and components used. These systems rely on a network of sensors and processing units to gather data and automate responses.
Temperature Sensors:
- Temperature sensors monitor critical areas, such as the combustion chamber in gas turbines or the steam flow in steam turbines. Any deviations in temperature are immediately flagged for analysis, allowing for prompt adjustments to avoid overheating.
Pressure Sensors:
- Pressure sensors track the flow of fluid through the turbine, ensuring that the pressure remains within safe limits. In steam turbines, pressure sensors monitor each stage, ensuring that pressure changes align with operational requirements.
Vibration Sensors:
- Vibration sensors detect abnormal vibrations, which could indicate imbalance, misalignment, or bearing wear. Continuous vibration monitoring is essential for early fault detection, helping operators address potential issues before they lead to failures.
Speed Sensors:
- Speed sensors measure the rotational speed of the turbine rotor, ensuring it matches the desired output. Speed sensors are particularly important in applications like wind turbines, where varying wind conditions require constant speed adjustments.
Data Processing Units and Control Loops:
- Data processing units collect, analyze, and process data from sensors, facilitating real-time adjustments to the turbine’s operation. Control loops automate responses, maintaining stable performance by adjusting parameters like speed and temperature based on sensor input.
Connectivity and Communication Protocols:
- Modern control and monitoring systems use communication protocols like Ethernet, Modbus, and OPC to transmit data across the network, enabling centralized monitoring and remote access. This connectivity enhances system integration and enables seamless data flow across the turbine’s components.
These sensors and components form the foundation of control and monitoring systems, supporting accurate data collection and real-time response for optimal turbine performance.
6. Maintenance and Operational Challenges
Maintaining control and monitoring systems is essential to ensure accurate data collection, reliable performance, and operational safety. However, these systems are prone to challenges that require regular maintenance and monitoring.
Common Issues:
- Sensor Drift: Over time, sensors can experience drift, where measurements deviate from actual values. Regular calibration is essential to maintain sensor accuracy and ensure reliable data.
- Data Inaccuracy: Data inaccuracy can result from faulty sensors or signal interference. Accurate monitoring depends on reliable data, making regular checks and diagnostics critical.
- Connectivity Problems: Communication issues between sensors and processing units can disrupt data flow, affecting real-time monitoring. Maintaining strong connectivity and troubleshooting network issues are essential for seamless operation.
Maintenance Practices for System Reliability:
- Calibration: Sensors require regular calibration to ensure accuracy. This process involves comparing sensor readings to known standards and adjusting them to match, minimizing errors.
- Testing and Diagnostics: Regular testing helps identify potential issues before they affect turbine performance. Diagnostics software can detect faults in control loops or data processing units, allowing for prompt repairs.
- Predictive Maintenance: Many control and monitoring systems use predictive maintenance, where data trends indicate when a component may need replacement. Predictive maintenance minimizes unexpected failures and reduces repair costs.
By implementing regular maintenance practices, operators ensure that control and monitoring systems function effectively, supporting turbine reliability, efficiency, and safety.
Introduction to Turbines and Energy Conversion
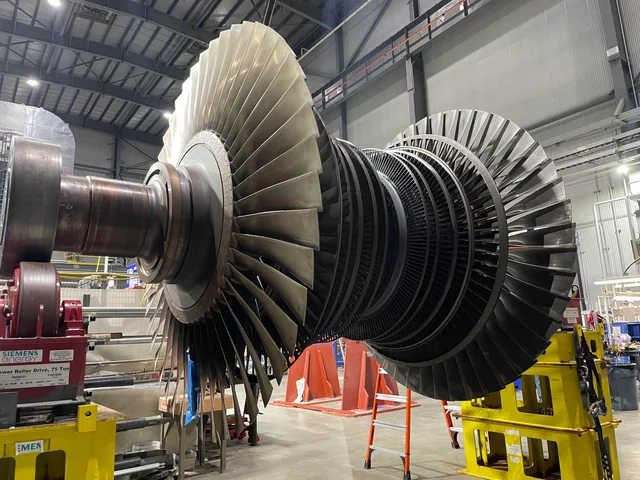
Turbines are essential devices in power generation, converting various forms of energy—thermal, kinetic, and potential—into mechanical energy, which is then converted into electrical power. Each type of turbine utilizes a unique mechanism to capture and transform energy from a specific source, such as steam, gas, water, or wind, making them critical components in a wide range of power plants.
Different turbine types are tailored to meet the requirements of their energy sources and operational environments. Steam turbines are often used in thermal and nuclear power plants, converting high-pressure steam into mechanical energy. Gas turbines rely on combustion processes, making them ideal for natural gas and peak power plants. Hydroelectric turbines harness the kinetic energy of flowing water in dams and rivers, while wind turbines capture the energy of wind to generate electricity. Each turbine type comes with its own set of advantages, efficiency factors, and challenges, reflecting the diversity and adaptability of turbine technology in modern power generation.
By understanding the unique mechanisms of each type, we gain insight into how turbines are optimized for specific applications and fuel sources, supporting both traditional and renewable energy production.
2. Steam Turbines
Steam turbines are a foundational technology in power generation, primarily used in fossil fuel and nuclear power plants. They operate based on the Rankine cycle, where water is heated, converted into high-pressure steam, and expanded through turbine blades, transforming thermal energy into mechanical energy.
Mechanism: Rankine Cycle and Pressure Stages:
- The Rankine cycle begins with water heated in a boiler, typically using coal, gas, or nuclear energy. The water is transformed into high-pressure steam, which flows into the turbine.
- As steam expands through the turbine, it passes through multiple stages, each with its own set of blades. The pressure decreases at each stage, transferring energy from the steam to the turbine rotor and creating rotational motion.
- Once the steam exits the turbine, it moves into a condenser, where it is cooled and converted back into water, completing the cycle. This closed-loop system maximizes the use of steam and reduces fuel consumption.
Types of Steam Turbines: Impulse and Reaction:
- Impulse Turbines: In impulse turbines, steam is directed through nozzles that accelerate it into high-speed jets. These jets hit the turbine blades, imparting kinetic energy and causing rotation. The steam’s pressure remains constant as it passes through the impulse blades.
- Reaction Turbines: Reaction turbines rely on pressure changes across the blades. As steam flows over the curved blades, its pressure drops, creating a reaction force that drives rotation. These turbines are more efficient at capturing energy from high-pressure steam over multiple stages.
Applications in Power Plants:
- Steam turbines are widely used in coal, natural gas, and nuclear power plants due to their ability to handle high-temperature and high-pressure steam. In nuclear plants, steam turbines convert energy from nuclear fission into electricity.
Advantages, Limitations, and Efficiency Factors:
- Steam turbines are known for their high efficiency and capacity to generate large amounts of power. By using multiple stages, they capture energy from steam at various pressure levels, increasing energy extraction.
- However, steam turbines require substantial cooling systems and regular maintenance to manage wear from high temperatures and pressures. Despite these challenges, they remain a reliable and scalable solution for baseload power generation.
Steam turbines have proven to be one of the most efficient and adaptable technologies in power generation, capable of converting various fuel sources into large-scale electricity.
3. Gas Turbines
Gas turbines are widely used in power generation and aviation, converting the chemical energy of natural gas or jet fuel into mechanical energy. They operate on the Brayton cycle, which includes compression, combustion, and expansion processes that generate high-speed rotation in the turbine.
Mechanism: Brayton Cycle:
- The Brayton cycle begins with the compression of air in a multi-stage compressor, raising its temperature and pressure. This high-pressure air then enters the combustion chamber.
- In the combustion chamber, fuel is injected and ignited, creating high-temperature, high-pressure combustion gases. These gases expand rapidly and are directed into the turbine.
- As the gases flow over the turbine blades, they transfer kinetic energy to the rotor, causing it to spin. This rotational energy powers both the compressor (in a closed-loop setup) and the generator, producing electricity.
Components: Compressor, Combustion Chamber, and Turbine Section:
- Compressor: The compressor draws in and pressurizes air, making it a critical component in setting up efficient combustion. Multi-stage compressors are commonly used to reach the desired pressure.
- Combustion Chamber: Here, fuel is mixed with compressed air and ignited, producing the high-energy gases that drive the turbine.
- Turbine Section: The turbine section is where the expansion of gases occurs, turning the blades and transferring energy to the rotor. Cooling systems are often integrated into the blades to manage the intense heat generated by combustion.
Applications in Natural Gas and Combined-Cycle Plants:
- Gas turbines are widely used in natural gas power plants, where they can quickly start up and respond to peak demand. They are also central to combined-cycle power plants, where exhaust heat from the gas turbine is used to generate steam for a steam turbine, maximizing efficiency.
Advantages and Challenges:
- Gas turbines are known for their fast startup times and high efficiency, especially in combined-cycle applications where they can achieve efficiencies above 60%.
- However, they operate at extremely high temperatures, which requires advanced materials and cooling techniques. Fuel cost fluctuations and emissions are also considerations, though modern gas turbines have reduced emissions through cleaner combustion processes.
Overall, gas turbines offer a flexible and efficient solution for meeting variable power demands, making them essential in both standalone and combined-cycle configurations.
4. Hydroelectric Turbines
Hydroelectric turbines harness the kinetic and potential energy of flowing water to produce mechanical energy. They are essential components in renewable hydroelectric power plants, converting the gravitational energy of water into electricity with minimal emissions.
Mechanism: Conversion of Water Flow Energy:
- Hydroelectric turbines use water’s kinetic and potential energy as it flows from higher to lower elevations. The pressure and flow rate of water generate force on the turbine blades, causing rotation.
- The turbine is typically connected to a generator, which converts the rotational energy into electricity. The mechanism is relatively simple, relying on the consistent movement of water to drive energy production.
Types of Hydroelectric Turbines:
- Pelton Turbines: Pelton turbines are impulse turbines, ideal for high-head, low-flow conditions. Water is directed through nozzles, creating high-speed jets that strike the turbine buckets, producing rotational energy. They are commonly used in mountainous regions where water drops from significant heights.
- Francis Turbines: Francis turbines are reaction turbines suited for medium to high-head applications. Water flows through the spiral casing and over the turbine blades, causing rotation. These turbines are versatile and widely used in hydroelectric power plants with moderate water flows.
- Kaplan Turbines: Kaplan turbines are axial-flow reaction turbines designed for low-head, high-flow conditions. They function similarly to a propeller, with adjustable blades that optimize performance based on flow conditions. Kaplan turbines are often used in river dams and tidal plants.
Applications in Renewable Hydroelectric Plants:
- Hydroelectric turbines are used in dam-based, run-of-river, and pumped-storage hydroelectric plants. They provide a renewable, consistent power source that can scale from small to large applications, depending on water availability and demand.
Advantages and Environmental Considerations:
- Hydroelectric turbines offer renewable energy production with minimal operational emissions. They can provide baseload power and peak load adjustments in pumped-storage systems.
- However, dam-based hydroelectric plants can impact local ecosystems, fish migration, and water quality. Sustainable hydroelectric designs focus on minimizing these effects through fish ladders, flow control, and habitat restoration.
Hydroelectric turbines play a central role in renewable energy, providing a dependable power source with low operational costs and environmental impact when sustainably managed.
5. Wind Turbines
Wind turbines capture the kinetic energy of wind to produce clean, renewable electricity. They operate by using aerodynamic forces, specifically lift and drag, to spin the rotor, converting wind energy into mechanical rotation.
Mechanism: Aerodynamic Lift and Drag:
- Wind turbines use large blades that are shaped like airplane wings. As wind flows over the curved blade surface, it creates a difference in pressure between the upper and lower surfaces, generating lift.
- This lift causes the rotor to spin. The rotational motion is transferred to a generator, where it is converted into electrical energy. The turbine’s orientation and blade pitch are adjusted based on wind direction and speed to maximize efficiency.
Types of Wind Turbines:
- Horizontal-Axis Wind Turbines (HAWT): The most common type, where blades rotate around a horizontal axis. HAWTs are highly efficient and are typically used in large-scale wind farms, both onshore and offshore.
- Vertical-Axis Wind Turbines (VAWT): These turbines have blades that rotate around a vertical axis, allowing them to capture wind from any direction. While less common, VAWTs are useful in turbulent wind environments and urban settings.
Applications in Onshore and Offshore Wind Farms:
- Wind turbines are installed in onshore and offshore wind farms to capture wind energy at different locations. Offshore wind farms have the advantage of stronger, more consistent winds, while onshore farms offer easier installation and maintenance.
Advantages in Renewable Energy:
- Wind turbines provide a clean, sustainable energy source that reduces carbon emissions. However, challenges include the variability of wind and the visual and noise impacts of onshore wind farms, which are addressed by advanced siting and design improvements.
Wind turbines are vital to global renewable energy goals, offering an effective way to harness wind power for sustainable electricity generation.
Introduction to Turbine Operational Dynamics
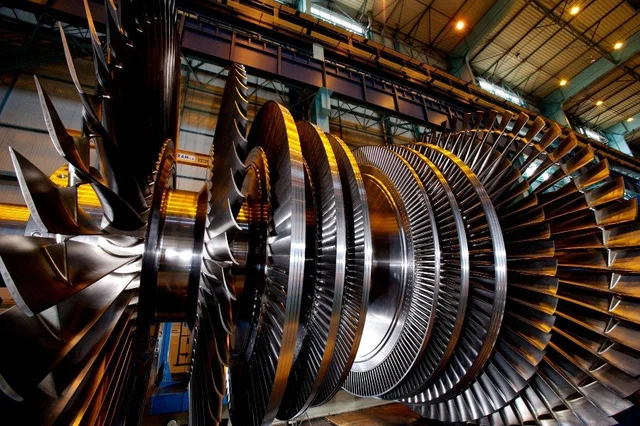
Turbines play a pivotal role in power generation, converting various forms of energy—whether from steam, gas, water, or wind—into mechanical energy. This energy is then transferred to a generator, where it is transformed into electricity. The operational dynamics of turbines encompass the physical and mechanical principles that govern how turbines convert fluid energy into rotational energy, maintain efficiency under varying conditions, and adapt to changes in load and environmental factors.
Understanding the dynamics of turbine operation is critical for optimizing performance, extending operational lifespan, and minimizing fuel and maintenance costs. As turbines operate at high speeds and under intense pressures and temperatures, a range of factors—including thermodynamics, fluid dynamics, load management, and control systems—must be carefully managed to maintain stability and efficiency. By exploring these dynamics, we gain insight into the complex interactions that define turbine performance and reliability.
2. Thermodynamic Principles in Turbine Operation
Thermodynamics is fundamental to turbine operation, governing how energy is converted from one form to another within the turbine. In power generation, turbines typically rely on thermodynamic cycles that describe how heat energy is converted into work.
Energy Conversion through Heat and Work:
- In a turbine, energy conversion occurs as high-pressure, high-temperature fluid (steam, combustion gases, or water) expands and flows over the blades. This expansion reduces the fluid’s pressure, transferring energy to the rotor as it spins.
- The efficiency of this energy conversion depends on maintaining an optimal temperature and pressure gradient. By managing these thermodynamic properties, turbines achieve high efficiency and effective energy capture.
Thermodynamic Cycles: Rankine, Brayton, and Hydraulic:
- Rankine Cycle: Used in steam turbines, the Rankine cycle involves heating water to create steam, which then expands through the turbine to generate mechanical energy. After expansion, the steam is condensed back into water, creating a closed loop that minimizes waste.
- Brayton Cycle: The Brayton cycle applies to gas turbines and involves compressing air, mixing it with fuel, and igniting the mixture. The resulting high-pressure gas expands through the turbine, converting thermal energy into mechanical work.
- Hydraulic Cycle: In hydroelectric turbines, gravitational potential energy of water is converted into kinetic energy as it flows through the turbine, creating rotation without significant heat generation.
Entropy, Enthalpy, and Efficiency:
- Entropy measures the disorder within a system and represents energy loss due to inefficiencies. Minimizing entropy in a turbine system helps maximize energy extraction from the working fluid.
- Enthalpy represents the total energy within the fluid. The difference in enthalpy as fluid enters and exits the turbine reflects the amount of energy captured. Efficiency improvements often focus on maximizing this enthalpy difference.
- By managing thermodynamic principles effectively, turbines operate more efficiently, with higher energy conversion rates and reduced fuel requirements, making them ideal for power generation across various applications.
Understanding these thermodynamic cycles and properties is crucial for designing turbines that capture maximum energy from their fluid sources, supporting efficient and reliable power generation.
3. Fluid Dynamics and Aerodynamics in Turbine Blades
Fluid dynamics is central to turbine operation, as it defines how fluid flows over the blades and transfers energy to the turbine’s rotor. Efficient fluid flow and optimal aerodynamic design are key to maximizing energy transfer and reducing operational inefficiencies.
Fluid Flow and Pressure Management:
- Turbine operation relies on directing fluid at high speeds and pressures over the blades to create rotational force. The flow and pressure must be precisely managed to ensure that fluid strikes the blades at an optimal angle, maximizing energy transfer.
- Impulse Turbines: In impulse turbines, fluid is directed through nozzles, creating high-speed jets that strike the blades. The fluid’s pressure remains constant, and only its velocity changes.
- Reaction Turbines: In reaction turbines, fluid pressure decreases across the blades, creating a pushing force that drives rotation. This requires careful pressure management to optimize energy capture.
Lift, Drag, and Turbulence:
- Lift is generated by the shape of turbine blades, allowing fluid to exert force that drives rotation. The blade’s angle and curvature are engineered to maximize lift while minimizing drag.
- Drag represents resistance as fluid flows over the blade surface. Reducing drag improves efficiency by allowing smoother fluid flow, which is particularly important in high-speed turbines like gas and steam turbines.
- Turbulence disrupts fluid flow and leads to energy loss. Turbine blades are designed with smooth surfaces and optimized shapes to minimize turbulence and ensure laminar flow, where fluid moves in orderly layers.
Aerodynamic Optimization:
- Aerodynamic principles guide blade design, with the goal of capturing as much energy as possible from the fluid. Computational Fluid Dynamics (CFD) models are often used to simulate fluid flow and refine blade shapes, angles, and surface textures.
- This aerodynamic optimization reduces energy loss, allowing turbines to operate at higher efficiencies and with reduced fuel consumption.
Effective fluid dynamics and aerodynamics are therefore essential for achieving maximum performance in turbine operation, ensuring that fluid energy is efficiently converted into mechanical power.
4. Load Management and Efficiency Optimization
Turbine efficiency is significantly influenced by how well it handles load variations. Load management enables turbines to adjust to fluctuations in demand, optimizing energy output and minimizing fuel consumption.
Role of Load Adjustments in Turbine Efficiency:
- In power plants, turbines are often required to operate under varying load conditions. Load refers to the amount of power the turbine is producing relative to its maximum capacity. Managing load efficiently is critical for achieving optimal performance and reducing fuel use.
- By adjusting load, operators can match power generation to demand. This is particularly important for natural gas turbines in peak-load power plants and hydroelectric turbines that adjust output based on water flow.
Partial Load vs. Full Load Dynamics:
- Full Load Operation: At full load, turbines operate at peak efficiency, utilizing maximum fluid flow and pressure. This is typically the preferred mode for baseload power plants, such as coal-fired or nuclear plants.
- Partial Load Operation: When operating below capacity, turbines are less efficient due to reduced fluid pressure and lower energy transfer rates. However, turbines designed with adaptive load management features can maintain efficiency even at partial loads.
Variable Inlet Guide Vanes and Other Load Management Technologies:
- Variable inlet guide vanes (VIGVs) help control the angle and volume of fluid entering the turbine, allowing it to adjust efficiently to different load levels. By managing fluid flow, VIGVs optimize performance across a wide range of operating conditions.
- Other adaptive technologies include pitch control in wind turbines, which adjusts blade angles based on wind speed, and bypass valves in gas turbines, which help regulate airflow and maintain stable operation during load changes.
Effective load management enables turbines to operate efficiently, adapt to demand fluctuations, and reduce fuel consumption, supporting reliable and flexible power generation.
5. Control Systems and Real-Time Monitoring
Control systems and real-time monitoring are essential for managing turbine stability, safety, and performance under dynamic conditions. These systems use sensors and data analytics to automate adjustments and ensure turbines operate within optimal parameters.
Role of Control Systems:
- Control systems regulate key parameters such as temperature, speed, and pressure to maintain stable turbine operation. They automate adjustments, such as changing fluid flow rates or blade angles, based on real-time data to match output with demand.
- For example, in gas turbines, control systems manage fuel flow and combustion temperatures, adjusting them to optimize power output and reduce emissions.
Sensors and Data Analytics:
- Sensors measure temperature, pressure, vibration, and speed across the turbine. This data is collected and processed to provide insights into the turbine’s operational health.
- Advanced data analytics and machine learning algorithms analyze these data trends, predicting maintenance needs and identifying performance improvement opportunities.
Predictive Maintenance and Safety:
- Real-time monitoring supports predictive maintenance by identifying signs of wear or component degradation before failures occur. This proactive approach minimizes downtime and maintenance costs.
- Control systems also enhance safety by automatically shutting down the turbine or reducing load in the event of abnormal conditions, such as excessive vibration or high temperatures.
Through real-time adjustments and continuous monitoring, control systems ensure turbine efficiency, reliability, and safety, optimizing performance across a range of operating conditions.
6. Challenges in Turbine Operation
Turbine operation involves a range of challenges, as these machines operate under intense conditions that can cause wear and operational issues. Addressing these challenges is critical for maintaining turbine efficiency and preventing costly downtime.
Thermal Expansion and Material Fatigue:
- High temperatures cause components to expand and contract, which can lead to material fatigue and cracks over time. Thermal cycling, common in gas and steam turbines, stresses components, leading to potential failures if not managed properly.
- High-quality materials and effective cooling systems help mitigate thermal expansion and reduce the risk of material degradation.
Vibration and Imbalance:
- Vibration is a common issue in turbines, especially when components become unbalanced due to wear or improper alignment. Excessive vibration can damage bearings, reduce efficiency, and even cause structural failures.
- Vibration sensors and balancing techniques help maintain stability, ensuring smooth operation and preventing wear.
Operational Challenges in High-Stress Environments:
- In high-temperature environments like gas turbines, cooling systems are essential to prevent overheating. However, cooling effectiveness can vary depending on load and environmental conditions, making it a constant challenge to maintain optimal temperatures.
- Hydraulic turbines face challenges with cavitation, where vapor bubbles form and collapse, causing erosion on blade surfaces. Managing pressure and flow rates helps reduce cavitation and extend blade life.
By addressing these challenges through advanced materials, regular maintenance, and monitoring, operators can enhance turbine longevity, maintain efficiency, and reduce operational risks.
Introduction to Turbine Efficiency and Technology
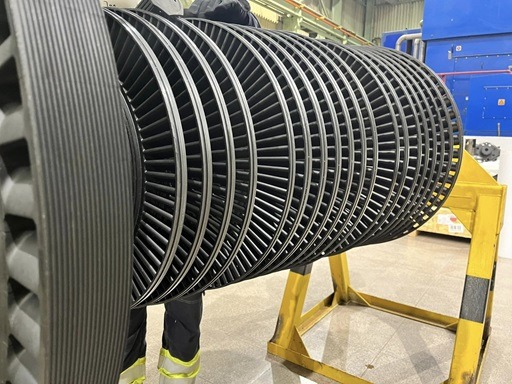
The efficiency of turbines directly impacts the effectiveness of power generation, fuel consumption, and emissions, making it a crucial focus area for both traditional and renewable energy sources. As turbines are responsible for converting various forms of energy—whether thermal, kinetic, or potential—into mechanical power, optimizing this conversion process is key to improving energy production while reducing environmental impact and operational costs.
Technological advancements in materials, aerodynamics, digitalization, hybrid systems, and control mechanisms have greatly enhanced turbine efficiency over the past few decades. Each innovation has contributed to higher energy capture rates, reduced maintenance needs, and increased reliability. From gas and steam turbines in thermal power plants to wind and hydro turbines in renewable energy systems, these advancements help turbines operate at their peak performance under a range of operating conditions.
By leveraging cutting-edge technology, modern turbines achieve better fuel efficiency, longer operational lifespans, and lower emissions, supporting sustainable energy generation. This ongoing technological evolution is essential for meeting global energy demands while transitioning to more environmentally friendly power sources.
2. Advanced Materials and Coatings
Material science has been a significant driver of turbine efficiency, as materials determine the durability, temperature tolerance, and overall performance of turbine components. High-quality materials and protective coatings allow turbines to operate at higher temperatures and pressures, reducing energy loss and increasing power output.
High-Temperature Alloys and Ceramic Composites:
- In gas turbines, where operating temperatures can exceed 1,500°C, high-temperature alloys like nickel-based superalloys are used to withstand extreme heat without deforming. These alloys improve the longevity of turbine components, minimizing the need for replacements and enhancing operational stability.
- Ceramic composites are also gaining traction for their heat-resistant properties. These materials allow turbine blades to withstand intense temperatures while remaining lightweight, contributing to greater efficiency. Ceramics are particularly valuable in high-temperature regions of gas turbines where traditional metals would degrade.
Thermal Barrier Coatings (TBCs):
- Thermal barrier coatings are essential for protecting turbine blades from heat damage. These coatings, often made from ceramic-based materials, create an insulating layer that shields the underlying metal from direct exposure to high-temperature gases.
- TBCs reduce the temperature at the blade surface, allowing turbines to operate at higher combustion temperatures, which directly correlates with higher efficiency in gas turbines. With this technology, turbines can achieve increased fuel efficiency and power output.
Erosion- and Corrosion-Resistant Materials:
- Wind and hydro turbines, which operate in variable environmental conditions, benefit from erosion- and corrosion-resistant materials. Stainless steel alloys and special coatings prevent material degradation due to moisture, particles, and high-speed impacts.
- In hydro turbines, erosion from cavitation (formation and collapse of vapor bubbles) is a common issue. Hard, erosion-resistant coatings like tungsten carbide protect against such wear, extending the lifespan of critical components and improving overall turbine efficiency.
Advanced materials and coatings not only increase durability but also enable turbines to operate at higher efficiencies and temperatures. This reduces maintenance needs, extends component lifespan, and supports reliable, long-term energy generation.
3. Aerodynamic Improvements and Blade Design
Aerodynamics is a core aspect of turbine efficiency, as blade design determines how effectively energy is captured from fluid flow. Recent advancements in computational modeling, materials, and blade mechanics have significantly improved blade performance across various turbine types.
Computational Fluid Dynamics (CFD) in Blade Design:
- CFD simulations allow engineers to analyze and refine blade shapes based on how fluid flows over them. By simulating real-world conditions, CFD enables precise adjustments to blade geometry, minimizing drag and turbulence.
- CFD also provides insights into pressure distribution and potential areas of energy loss, helping designers optimize blade angles and surfaces to maximize lift. This is especially useful in wind turbines, where aerodynamic efficiency is critical for capturing wind energy.
Adaptive Blade Pitch Control in Wind Turbines:
- Blade pitch control enables wind turbines to adjust the angle of their blades based on wind speed, optimizing efficiency. When wind speeds are high, the blades adjust to reduce drag and prevent overspeeding, while at lower speeds, they tilt to capture more wind energy.
- This adaptive pitch control technology allows wind turbines to maximize energy capture while operating safely under various wind conditions, enhancing their overall efficiency and reliability.
Advanced Blade Shapes and Surface Textures:
- Blade shapes have evolved to include advanced curvatures that enhance lift and reduce drag. For example, steam and gas turbine blades are designed with slight curves and optimized angles that guide fluid smoothly across the surface, increasing energy transfer efficiency.
- Surface textures and coatings also improve aerodynamics by reducing air or water resistance. In hydro turbines, smooth and hydrodynamic blade coatings minimize friction and cavitation, while in wind turbines, surface textures help prevent airflow separation, reducing energy loss.
Aerodynamic improvements in blade design allow turbines to capture more energy from fluid flow, operate efficiently at varying speeds, and reduce losses from drag and turbulence. These advancements contribute to enhanced power output and reduced wear on turbine components.
4. Digitalization and Data Analytics for Predictive Maintenance
Digitalization has revolutionized turbine efficiency through advanced monitoring and predictive maintenance capabilities. By integrating Internet of Things (IoT) sensors and data analytics, modern turbines are capable of real-time performance tracking, issue detection, and proactive maintenance.
IoT Sensors and Real-Time Monitoring:
- IoT sensors embedded in turbines track key performance indicators, including temperature, pressure, vibration, and rotational speed. These sensors continuously collect data, providing operators with insights into the turbine’s operational health and efficiency.
- Real-time monitoring allows for immediate detection of anomalies, enabling operators to address issues before they escalate. This reduces downtime and enhances the reliability of the turbine, supporting continuous and efficient power generation.
Predictive Maintenance Using AI and Machine Learning:
- Machine learning algorithms analyze data from sensors to predict when components might fail or need maintenance. This predictive maintenance approach minimizes unexpected breakdowns by identifying patterns that indicate wear or degradation.
- For example, vibration data may show gradual increases over time, signaling potential bearing wear. By addressing these early indicators, operators can replace parts before failure occurs, reducing repair costs and improving turbine uptime.
Digital Twins for Performance Optimization:
- A digital twin is a virtual model of the turbine that mirrors its real-time performance. By using real-world data, digital twins simulate operational conditions and predict outcomes, helping operators optimize settings for maximum efficiency.
- Digital twins also provide insights into potential adjustments, such as fluid flow rates or blade angles, that could improve performance. This technology allows operators to test scenarios virtually before applying changes to the actual turbine, reducing risk and enhancing efficiency.
The use of IoT, data analytics, and digital twins enables turbines to operate with higher reliability and efficiency, extending operational life, reducing maintenance costs, and supporting optimized performance.
5. Hybrid Systems and Combined Cycle Innovations
Hybrid systems and combined-cycle technologies have significantly increased turbine efficiency by integrating multiple energy conversion processes. These innovations improve energy utilization, reduce fuel consumption, and lower emissions, making them valuable in both conventional and renewable energy contexts.
Integration of Gas and Steam Turbines in Combined-Cycle Plants:
- Combined-cycle power plants use both gas and steam turbines to maximize energy extraction from fuel. In a combined-cycle system, a gas turbine generates electricity through combustion, and the hot exhaust gases are used to produce steam that powers a steam turbine.
- This dual-stage process increases overall efficiency, as the waste heat from the gas turbine is repurposed rather than lost. Combined-cycle plants can achieve efficiencies exceeding 60%, making them one of the most efficient power generation options available.
Waste Heat Recovery Technologies:
- Waste heat recovery systems capture and reuse thermal energy that would otherwise be lost in the exhaust. Heat recovery steam generators (HRSGs) are commonly used in combined-cycle plants to convert exhaust heat into steam, improving energy efficiency and reducing fuel costs.
- These technologies are also valuable in renewable energy applications, where waste heat from geothermal or biomass processes can be captured to boost efficiency.
Advantages of Hybrid Systems in Emission Reduction and Cost Savings:
- Hybrid systems reduce emissions by maximizing energy extraction from fuel, minimizing the need for additional fuel input. This is particularly beneficial in natural gas plants, where lower emissions are a priority.
- By increasing efficiency and reducing fuel costs, hybrid systems provide a cost-effective and environmentally friendly approach to power generation.
Hybrid and combined-cycle technologies demonstrate how integrating multiple energy sources and processes can achieve higher efficiencies, lower emissions, and improve overall sustainability.
6. Enhanced Control Systems and Automation
Control systems and automation play a crucial role in maintaining turbine efficiency, especially under fluctuating demand and environmental conditions. Advances in automation and adaptive control mechanisms have made turbines more responsive, efficient, and reliable.
Role of Advanced Control Systems in Load and Speed Management:
- Advanced control systems adjust turbine parameters like speed, load, and temperature to maintain optimal performance. In response to changes in demand, these systems regulate fuel flow, fluid pressure, and rotational speed to match power output with consumption.
- By automating load management, control systems ensure that turbines operate within efficient ranges, minimizing energy waste and improving fuel economy.
Smart Grids and Turbine Automation for Demand Response:
- Smart grid technology enables turbines to respond automatically to shifts in demand across the power grid. In renewable energy applications, such as wind and hydroelectric plants, automation allows turbines to adapt to changes in wind speed or water flow, ensuring efficient energy capture.
- Demand response capabilities help stabilize the grid, allowing power plants to adjust output in real time, reducing energy losses and maintaining balance between supply and demand.
Enhanced Efficiency Through Adaptive Control Mechanisms:
- Adaptive control mechanisms continuously monitor turbine performance and make real-time adjustments to maintain efficiency. For example, gas turbines may adjust combustion temperature based on current load requirements, optimizing fuel usage.
- These adaptive controls improve operational stability, reduce emissions, and enhance fuel efficiency, allowing turbines to operate more sustainably.
By automating control and incorporating adaptive mechanisms, turbines achieve higher efficiency, improved reliability, and greater flexibility in response to changing operational demands.
Role of Turbines in Energy Conversion
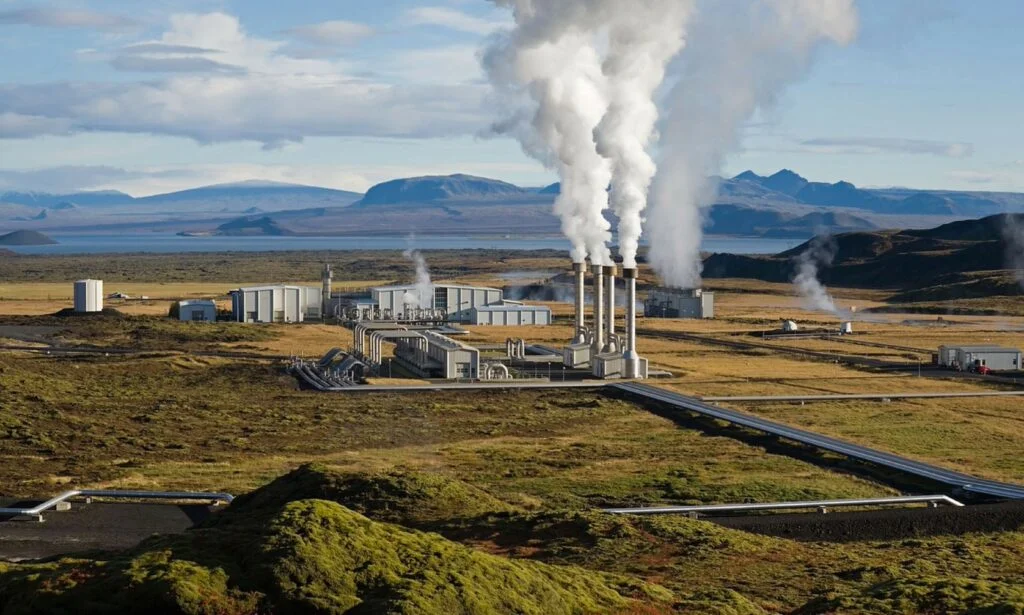
Turbines are a central technology in power generation, responsible for converting various forms of energy—whether thermal, kinetic, or potential—into mechanical energy, which is then transformed into electricity. These systems are essential across a wide range of power plants, from conventional fossil-fuel-based plants to renewable sources like hydroelectric, wind, and geothermal energy facilities. The universal goal for turbines is to maximize energy capture and conversion with minimal losses, making them crucial for efficient, large-scale electricity generation.
Each type of turbine operates differently depending on the energy source:
- Steam turbines rely on thermal energy, typically from steam produced by burning fossil fuels or nuclear reactions. This steam drives the turbine blades, creating rotational energy.
- Gas turbines use combustion gases from fuel and compressed air mixtures to generate high-pressure exhaust that spins the turbine rotor.
- Hydroelectric turbines harness the kinetic energy of flowing water, while wind turbines capture the kinetic energy of wind.
- Geothermal turbines extract thermal energy from naturally heated groundwater or steam beneath the Earth’s surface.
Through these different mechanisms, turbines facilitate the conversion of available energy into mechanical power, which powers an electric generator. The efficiency of this process directly influences power output, fuel consumption, emissions, and the overall effectiveness of energy systems. Because of this, technological advances have consistently focused on enhancing turbine efficiency to improve the performance, reliability, and sustainability of power generation.
2. Importance of Efficiency in Turbines
Efficiency is one of the most critical metrics for evaluating turbine performance, as it reflects how effectively a turbine can convert its energy source into usable mechanical and electrical power. Higher turbine efficiency has a wide range of benefits, impacting not only energy production but also environmental sustainability and operational costs.
Fuel Consumption and Cost Reduction:
- For traditional fossil-fuel-based turbines, efficiency improvements mean that more power is produced per unit of fuel burned. This reduces fuel costs and lowers dependency on nonrenewable resources. Higher efficiency allows power plants to meet energy demands with fewer resources, optimizing operational expenses and reducing fuel imports in energy-dependent regions.
Emissions Reduction and Environmental Impact:
- Efficiency also has a direct effect on emissions. When turbines operate more efficiently, they consume less fuel to produce the same amount of power, which in turn reduces greenhouse gas and pollutant emissions. This is particularly significant in gas and coal power plants, where efficiency gains contribute to lowering carbon footprints.
- For renewable energy sources, efficiency improvements help increase the feasibility of clean energy by making renewable power generation more competitive with fossil fuels. Wind and hydro turbines, for example, capture more energy with each improvement in blade design or material quality, contributing to greener, more sustainable energy production.
Role of Efficiency in Energy Transition and Sustainability Goals:
- Improving turbine efficiency is a vital strategy in the global transition to more sustainable energy systems. With worldwide efforts focused on reducing carbon emissions and moving toward net-zero targets, efficient turbines help lower the environmental impact of energy generation.
- Additionally, efficiency improvements contribute to meeting growing energy demands sustainably. As global energy consumption rises, enhancing the efficiency of power generation technologies like turbines is essential to ensure a balance between supply, cost, and environmental responsibility.
In sum, turbine efficiency is not just about maximizing power generation but also about creating a positive impact on the environment and supporting sustainable energy transitions.
3. Key Technological Advances in Turbine Efficiency
Over the past few decades, numerous technological advancements have significantly boosted turbine efficiency, enabling turbines to achieve higher power outputs with less energy loss. These advances have come from various fields, including materials science, aerodynamics, and digital technology.
High-Temperature Materials and Coatings:
- Materials science has brought substantial improvements in turbine durability and heat tolerance. In gas turbines, where temperatures can exceed 1,500°C, high-temperature alloys like nickel-based superalloys and ceramic composites provide exceptional heat resistance, allowing turbines to operate at higher temperatures, which directly correlates with efficiency gains.
- Thermal barrier coatings (TBCs) are also essential for gas turbines, protecting blades from direct exposure to extreme heat. This allows the turbine to increase combustion temperatures without damaging components, maximizing thermal efficiency.
Aerodynamic Advances in Blade Design and Shape:
- Advanced blade shapes and designs have reduced aerodynamic drag, increased lift, and minimized turbulence, all of which contribute to energy capture and conversion. Computational Fluid Dynamics (CFD) models allow engineers to test and refine blade shapes for optimal performance under real-world conditions, improving fluid flow and reducing energy loss.
- In wind turbines, adaptive pitch control mechanisms adjust the blade angle based on wind speed, maximizing energy capture in variable conditions. For hydro turbines, optimized blade and casing designs reduce friction losses and improve overall efficiency.
Digital Technologies for Monitoring and Predictive Maintenance:
- The integration of IoT sensors and real-time monitoring systems has revolutionized turbine maintenance and operational efficiency. Sensors continuously monitor parameters like temperature, vibration, and pressure, providing data that informs predictive maintenance.
- By analyzing operational data, machine learning algorithms can detect patterns indicative of potential issues, allowing operators to address wear and tear before it results in costly downtime. This approach not only reduces maintenance costs but also extends the lifespan of turbines, supporting long-term efficiency.
Through these advancements, modern turbines are more efficient, reliable, and cost-effective than ever, supporting energy systems in meeting performance and environmental goals.
4. The Future of Turbine Efficiency and Sustainability
As the energy industry increasingly focuses on sustainability, the future of turbine technology will be shaped by innovations that further enhance efficiency and reduce environmental impact. Hybrid and combined-cycle systems are one promising area of development, where gas and steam turbines work together to capture more energy from fuel. In these systems, exhaust heat from a gas turbine is repurposed to generate steam, which powers a steam turbine, thereby increasing total energy output and fuel efficiency. These systems can achieve efficiencies above 60%, making them ideal for reducing emissions and fuel costs.
Digitalization and Smart Technology Integration:
- Digitalization will continue to play a pivotal role, with AI and machine learning enabling real-time performance optimization. Advanced control systems that adjust turbine parameters automatically in response to changing loads or environmental conditions are paving the way for more flexible, efficient power generation.
- The use of digital twins—virtual replicas of turbines that simulate performance under various conditions—will also enhance operational efficiency. By testing scenarios virtually, operators can optimize real-world performance, detect issues early, and improve reliability.
Renewable Energy and Sustainability Goals:
- Future advancements will also focus on renewable energy applications, making wind and hydro turbines more competitive with fossil fuels. As these technologies continue to improve, turbines will play an essential role in supporting clean energy transitions, meeting global energy demands sustainably, and reducing reliance on carbon-intensive power sources.
With ongoing technological progress, turbines are set to become even more efficient, versatile, and sustainable, supporting the future of energy with minimal environmental impact and optimal performance.
1. Introduction to Materials and Coatings in Turbines
Turbines operate under extreme conditions, where high temperatures, pressures, and fluid forces can cause significant wear and degradation. The choice of materials and protective coatings is therefore crucial in ensuring that turbine components withstand these harsh conditions, maintain durability, and operate efficiently over extended periods. Advanced materials like high-temperature alloys and ceramic composites have been developed specifically to endure these demands, while coatings such as thermal barriers and corrosion protectants provide an added layer of defense. Together, these innovations allow turbines to achieve greater efficiency, improved longevity, and more reliable performance across both traditional and renewable energy systems.
2. High-Temperature Alloys
High-temperature alloys are essential in turbine construction, particularly in gas turbines, where combustion processes expose components to extreme heat. Nickel-based superalloys are among the most widely used materials in high-temperature turbine components, as they offer exceptional resistance to heat, oxidation, and mechanical stress.
Properties of Nickel-Based Superalloys:
- Nickel-based superalloys, such as Inconel and Hastelloy, have unique metallurgical properties that allow them to retain strength at high temperatures. These alloys are designed with elements like chromium, cobalt, and aluminum, which improve their ability to withstand temperatures exceeding 1,500°C without deforming.
- These superalloys are resistant to oxidation and corrosion, which protects the turbine components from gradual degradation due to heat and exposure to corrosive gases.
Applications in Gas Turbine Blades and Vanes:
- Gas turbines rely on nickel-based superalloys for blades and vanes in the combustion and turbine sections. These components are subjected to direct contact with combustion gases, and their high durability ensures that they can handle rapid temperature fluctuations and high rotational speeds without failure.
- Superalloys maintain their structural integrity even when exposed to the intense pressures and stresses generated by fast-moving, high-temperature exhaust gases, ensuring continuous power generation and safe operation.
Benefits of Temperature Tolerance and Durability:
- Using high-temperature alloys enhances turbine efficiency by allowing gas turbines to operate at higher combustion temperatures, which directly increases thermal efficiency. Higher operating temperatures enable turbines to convert more of the fuel’s thermal energy into mechanical energy, thereby improving fuel economy and reducing emissions.
- The durability of these materials also reduces maintenance needs and extends the lifespan of critical components, supporting reliable turbine operation in high-demand settings like power plants and industrial facilities.
3. Ceramic Composites
Ceramic composites are another advanced material used in turbine construction, particularly in areas exposed to extreme heat. Ceramics are inherently resistant to high temperatures, providing an advantage in high-temperature applications where metals might degrade or deform.
Thermal Properties and Heat Resistance:
- Ceramics have excellent heat resistance, with the ability to withstand temperatures well above those tolerated by metal alloys. Ceramic composites, which combine ceramic materials with reinforcing fibers, exhibit enhanced strength and thermal stability, making them ideal for use in the hottest sections of gas turbines.
- Because ceramics are poor conductors of heat, they help insulate turbine components from intense thermal exposure, preserving the integrity of the surrounding structure and minimizing thermal expansion.
Applications in High-Temperature Zones:
- In gas turbines, ceramic composites are often used in components such as rotor blades and nozzle guides, where they experience direct exposure to high-temperature gases. Their lightweight nature also reduces the overall weight of these components, contributing to improved rotor speed and efficiency.
- Some advanced gas turbines employ ceramic matrix composites (CMCs) for hot gas path parts, as CMCs can tolerate the mechanical stresses of high-speed rotation while resisting heat-induced deformation.
Advantages in Weight Reduction and Thermal Stability:
- Ceramic composites are lighter than traditional metal alloys, which allows for faster turbine rotation and enhances overall efficiency. This weight reduction can also contribute to lower fuel consumption and operational costs in gas turbines.
- Their exceptional thermal stability means that ceramic components maintain their structural integrity under prolonged high-temperature operation, supporting consistent performance and reducing the need for cooling systems.
4. Thermal Barrier Coatings
Thermal barrier coatings (TBCs) are essential for protecting turbine components from high-temperature damage. Made primarily from ceramic materials, TBCs insulate turbine parts from direct exposure to hot gases, enabling turbines to operate at higher temperatures and achieve greater efficiency.
Purpose and Composition of TBCs:
- TBCs are typically composed of yttria-stabilized zirconia (YSZ), a ceramic material with low thermal conductivity and high resistance to thermal cycling. This ceramic coating is applied to the surface of turbine blades, vanes, and other components that face direct exposure to hot gases.
- The coating creates a thin insulating layer that reduces the temperature experienced by the underlying metal, allowing turbines to increase combustion temperatures without damaging critical parts.
How TBCs Enable Higher Operating Temperatures:
- By insulating turbine components, TBCs allow gas turbines to operate at higher combustion temperatures, which directly boosts thermal efficiency. In a typical gas turbine, the higher the temperature of the combustion gases, the more mechanical energy can be extracted, enhancing power output.
- TBCs also reduce thermal fatigue and oxidation, common issues in high-temperature turbine sections. By protecting the underlying metal from these stresses, TBCs extend component lifespan and support more reliable operation.
Role in Protecting Components and Improving Efficiency:
- TBCs play a critical role in preserving the structural integrity of turbine blades and other high-temperature components. Their resistance to cracking and chipping, even under rapid temperature changes, ensures that they provide consistent insulation.
- The ability of TBCs to allow turbines to operate at higher temperatures translates into improved fuel efficiency, as more of the fuel’s thermal energy is converted into mechanical power. This efficiency improvement reduces both fuel consumption and emissions, supporting environmentally friendly energy production.
5. Erosion- and Corrosion-Resistant Coatings
Erosion- and corrosion-resistant coatings are vital for protecting turbine components, especially in hydroelectric and wind turbines exposed to moisture, particles, and other environmental elements. These coatings extend component life by providing a protective layer that resists wear, rust, and degradation.
Importance in Wind and Hydroelectric Turbines:
- Wind and hydro turbines are exposed to various elements that can erode or corrode surfaces. Water droplets, dust, and particles can erode the leading edges of blades, while moisture and chemicals in the environment can corrode metal surfaces.
- Erosion- and corrosion-resistant coatings safeguard turbine blades, casings, and other components from these harsh conditions, preserving their structural integrity and efficiency over time.
Materials Like Tungsten Carbide for Erosion Resistance:
- Tungsten carbide and other hard coatings are often applied to surfaces prone to erosion, providing a durable protective layer that can withstand the impact of particles and droplets in high-speed fluid environments.
- In hydro turbines, these coatings prevent cavitation erosion, where vapor bubbles form and collapse against blade surfaces, causing pitting and material loss. By mitigating erosion, these coatings enhance the lifespan and performance of hydro turbine components.
Corrosion-Resistant Coatings for Moisture and Chemical Protection:
- Corrosion-resistant coatings, often based on stainless steel alloys or polymer-based films, protect turbine parts from rust and chemical exposure, which is especially beneficial in hydroelectric applications where constant water exposure can lead to corrosion.
- These coatings enable turbines to operate in a broader range of environments, making them more versatile and reliable for long-term energy production.
1. Introduction to Aerodynamics in Turbine Blades

Aerodynamics is a central factor in turbine blade design, as it determines how effectively a turbine can convert fluid energy into rotational force. Through aerodynamic improvements, turbines capture more energy, operate with reduced drag, and generate higher power outputs. Whether harnessing the kinetic energy of wind or the thermal energy of steam, aerodynamically optimized blades play a crucial role in turbine performance and efficiency. Key aerodynamic forces such as lift and drag are carefully managed to maximize the turbine’s energy capture, improve fuel economy, and minimize wear. Advances in blade shape, surface textures, and control technologies are pushing turbine efficiency to new heights.
2. Aerodynamic Principles: Lift, Drag, and Angle of Attack
In turbine operation, lift and drag are the primary aerodynamic forces that influence blade performance. These forces are managed through careful blade design and angle adjustments to optimize energy capture and minimize losses.
Lift and Drag in Blade Function:
- Lift is the force that enables the turbine blade to move by creating a difference in air pressure across the blade’s surfaces. When fluid flows over a blade with a curved shape, the pressure above the blade is lower than the pressure below, resulting in lift. This force drives the rotor, converting fluid energy into rotational motion.
- Drag is a resistance force that acts against the blade’s movement, typically caused by air or fluid friction as it moves over the blade’s surface. High drag reduces efficiency by slowing down the rotor and increasing energy loss, making drag reduction a priority in blade design.
Role of Angle of Attack in Maximizing Efficiency:
- The angle of attack is the angle at which the fluid meets the blade. Adjusting this angle helps maximize lift and minimize drag. When the angle of attack is too steep, drag increases, potentially causing stalling in wind turbines or reducing the efficiency of energy transfer in steam and gas turbines.
- In wind turbines, adaptive blade pitch control adjusts the angle of attack in real time to optimize lift and reduce drag in response to changing wind conditions. For steam and gas turbines, the blade angles are fixed, but optimized based on expected fluid velocities and pressures.
Application in Different Turbine Types:
- Wind Turbines: Lift and drag are critical in capturing wind energy and require precise adjustments in blade pitch for optimal performance.
- Steam and Gas Turbines: Lift aids in energy extraction, but drag reduction is equally crucial, as these turbines operate at high speeds where fluid resistance can lead to inefficiencies.
- Hydro Turbines: For water turbines, blade curvature and angle are adjusted to maximize energy capture from flowing water while reducing resistance, similar to wind and steam turbines.
By managing lift, drag, and the angle of attack, turbine blades capture more energy with greater efficiency, supporting more sustainable and cost-effective power generation.
3. Blade Shape Optimization and Surface Textures
Blade shape optimization is central to enhancing turbine efficiency, as the shape directly impacts lift, drag, and overall fluid dynamics. Innovations in blade curvature, angle, and surface textures enable turbines to capture more energy with minimal resistance, improving both performance and longevity.
Importance of Blade Shape in Reducing Drag and Improving Efficiency:
- The shape of a turbine blade is designed to maximize lift while minimizing drag. In wind turbines, blades are typically longer and more slender with an aerodynamic profile similar to an airplane wing. This shape helps streamline airflow, creating high lift with low drag, enabling the turbine to capture more wind energy.
- In steam and gas turbines, blade shape is more compact and optimized for handling high-pressure, high-velocity fluid. Curved blades allow for smoother fluid flow across surfaces, minimizing turbulence and drag. This efficient flow maximizes energy transfer from high-speed steam or gas to the turbine rotor, increasing power output.
Innovations in Curved Blade Design and Optimized Angles:
- Curved blades are carefully engineered with optimal angles to manage fluid flow across each stage of the turbine. In multi-stage steam and gas turbines, each blade row is angled to direct fluid smoothly onto the following row, reducing energy losses.
- In wind turbines, blades are often twisted, with the angle of the blade surface varying along the length of the blade. This twist ensures that each section of the blade captures wind efficiently, as wind speeds and directions can vary from root to tip.
Use of Surface Textures to Enhance Laminar Flow and Minimize Turbulence:
- Surface textures and coatings also play an important role in reducing drag by promoting laminar flow, where fluid flows smoothly over the blade surface. Textured coatings, like sharkskin-inspired riblets, reduce drag by channeling airflow more efficiently, reducing turbulence.
- In hydro turbines, smooth hydrodynamic surfaces reduce friction from water flow, increasing energy capture. Additionally, advanced coatings protect blades from erosion and cavitation, ensuring that the smooth surfaces remain intact and maintain efficiency over time.
Through optimized blade shapes, angles, and surface textures, turbines capture fluid energy more effectively and reduce energy loss due to resistance, supporting higher efficiency and better performance in diverse operating environments.
4. Computational Fluid Dynamics (CFD) in Blade Design
Computational Fluid Dynamics (CFD) has transformed turbine blade design by enabling detailed simulations of fluid flow across blade surfaces. CFD allows engineers to test and optimize blade shapes, angles, and surface textures in a virtual environment before actual production, reducing design time and improving overall efficiency.
Role of CFD in Simulating Fluid Flow and Refining Blade Shapes:
- CFD software simulates real-world fluid dynamics, allowing designers to visualize how fluid will interact with different blade shapes under varying conditions. Engineers can adjust the curvature, thickness, and angle of blades to achieve optimal lift, minimal drag, and efficient fluid flow.
- CFD analysis helps identify areas of high drag, turbulence, or pressure drops, allowing designers to make precise modifications that improve energy capture and minimize resistance.
Benefits of CFD in Reducing Design Time and Testing Conditions:
- By conducting virtual simulations, CFD reduces the need for extensive physical testing, which is both time-consuming and costly. CFD enables rapid prototyping, where multiple blade designs can be tested and refined in less time.
- CFD has contributed to aerodynamic improvements across all turbine types, from large wind turbine blades designed to capture maximum wind energy to compact gas turbine blades optimized for high-speed fluid flow.
Using CFD, designers have been able to achieve breakthroughs in blade efficiency, enabling turbines to operate with higher energy capture rates, lower drag, and improved overall performance.
5. Adaptive Blade Control and Real-Time Adjustments
Adaptive blade control technologies enable turbines to adjust blade angles in real-time, optimizing energy capture and reducing mechanical stress. These systems are particularly beneficial in environments where fluid flow varies, such as wind and hydro turbines.
Adaptive Blade Pitch Control in Wind Turbines:
- In wind turbines, adaptive pitch control allows blades to adjust their angle based on wind speed and direction. When wind speeds are high, blades pitch to reduce drag and prevent overspeeding. During lower wind speeds, blades adjust to maximize energy capture.
- This control enhances the turbine’s efficiency and prevents mechanical wear from fluctuating wind conditions.
Variable Geometry Blades in Gas and Steam Turbines:
- In some gas and steam turbines, variable geometry blades adjust to optimize performance under different load conditions. Variable inlet guide vanes, for example, control the angle at which fluid enters the turbine, ensuring efficient energy transfer.
Through adaptive control, turbines respond dynamically to changing conditions, enhancing efficiency, extending component life, and supporting reliable power generation.
1. Introduction to Hybrid and Combined-Cycle Systems
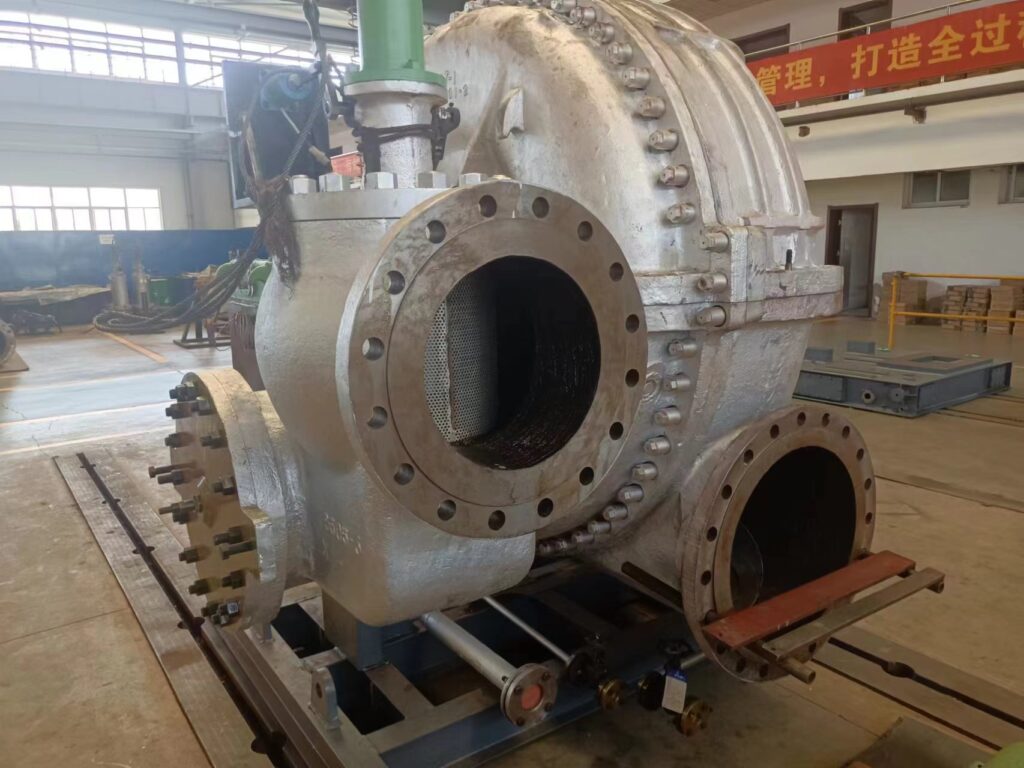
Hybrid and combined-cycle systems have transformed power generation by improving energy efficiency, reducing fuel consumption, and cutting emissions. By integrating multiple energy sources or processes, these systems capture more energy from each fuel unit, making power generation more sustainable and economical.
Combined-cycle plants use both gas and steam turbines to maximize energy extraction from natural gas. They operate by recovering the waste heat from a gas turbine to produce steam, which then powers a steam turbine. This dual process allows for higher overall efficiency and is widely used in natural gas power plants.
Hybrid systems combine different energy sources or storage solutions, such as solar, wind, geothermal, and battery storage, with conventional power sources like natural gas. These configurations help stabilize the grid, improve reliability, and make renewable energy more viable. Both hybrid and combined-cycle technologies are essential in meeting global energy demands sustainably, as they enhance fuel flexibility, optimize resource use, and support environmental goals.
2. Working Principles of Combined-Cycle Systems
Combined-cycle power plants are designed to utilize waste heat from gas turbines to drive steam turbines, creating a highly efficient dual-turbine system. This integration is based on the Brayton and Rankine cycles, which describe how gas and steam turbines convert thermal energy into mechanical energy.
Gas Turbine and Brayton Cycle:
- In a combined-cycle plant, the primary process begins with a gas turbine. Air is compressed and mixed with fuel, then ignited in the combustion chamber. The resulting high-pressure exhaust gases drive the gas turbine blades, generating mechanical power that is converted into electricity. This process follows the Brayton cycle, which is highly efficient but results in exhaust gases at high temperatures.
Heat Recovery Steam Generator (HRSG):
- Rather than releasing exhaust gases into the atmosphere, a combined-cycle system channels this waste heat into a Heat Recovery Steam Generator (HRSG). The HRSG captures the thermal energy from the exhaust gases to produce steam without additional fuel, maximizing energy utilization and efficiency.
Steam Turbine and Rankine Cycle:
- The generated steam then drives a steam turbine, producing additional power in what is known as the Rankine cycle. By converting exhaust heat into mechanical energy, the steam turbine supplements the gas turbine’s output, raising the plant’s overall efficiency.
Together, the gas and steam turbines create a two-stage process that boosts energy output with minimal additional fuel. Combined-cycle systems can reach efficiencies above 60%, significantly higher than single-cycle gas turbines, making them one of the most efficient power generation technologies available.
3. Advantages of Combined-Cycle Technology
Combined-cycle technology offers several significant advantages over conventional single-cycle power plants, including improved efficiency, reduced emissions, and operational flexibility.
Higher Efficiency and Fuel Savings:
- The two-stage process of combined-cycle plants allows for more complete energy extraction from fuel. By utilizing waste heat to drive a second turbine, combined-cycle plants achieve efficiencies exceeding 60%, compared to around 35-40% in conventional gas turbine plants. This high efficiency reduces fuel consumption, resulting in substantial cost savings over the plant’s operational life.
- Fuel flexibility is another benefit, as combined-cycle plants can operate on a variety of fuels, including natural gas, syngas, and even biofuels. This flexibility supports stable power generation under different fuel availability conditions.
Emissions Reduction and Environmental Impact:
- The fuel-efficient operation of combined-cycle plants results in fewer greenhouse gas emissions per unit of electricity generated, making them a cleaner alternative to coal-fired plants. With lower carbon dioxide, nitrogen oxide, and sulfur dioxide emissions, combined-cycle plants support environmental goals and align with global emissions reduction targets.
- Combined-cycle technology also reduces thermal pollution, as HRSG systems capture waste heat that would otherwise contribute to environmental warming.
Flexibility in Meeting Demand:
- Combined-cycle plants offer flexible operation, capable of ramping up quickly to meet peak demand or scaling back for base load. This flexibility is especially valuable in energy grids that integrate intermittent renewable sources like wind and solar, as combined-cycle plants can adjust output as needed to stabilize the grid.
These advantages make combined-cycle technology a key component of modern power generation, supporting both efficiency and sustainability goals.
4. Hybrid Systems in Renewable and Conventional Energy
Hybrid systems integrate renewable energy sources with conventional power plants, offering a flexible, efficient solution that reduces reliance on fossil fuels and supports grid stability. Hybrid configurations vary widely, incorporating sources such as solar thermal, wind, geothermal, and battery storage in ways that optimize energy production.
Integration with Solar and Geothermal Energy:
- Solar-thermal hybrid systems combine solar energy with natural gas or coal-fired plants. Solar energy preheats the water used in the steam generation process, reducing the fuel needed to produce steam. This configuration is particularly effective in sunny regions, reducing fuel consumption and emissions while increasing energy output.
- Geothermal hybrids combine geothermal energy with other power sources, such as natural gas. In this setup, geothermal energy provides a stable base load, while gas turbines ramp up during high-demand periods. This approach reduces the reliance on fossil fuels and offers a renewable base power source.
Battery Storage and Grid Support:
- Battery storage is increasingly integrated into hybrid systems to store excess power generated during low-demand periods. Stored energy can then be used to support the grid during peak times or when renewable sources are unavailable.
- Hybrid systems with battery storage provide consistent, reliable power output, helping mitigate issues with intermittency in renewable sources and supporting smoother grid operation.
By combining renewable and conventional energy sources, hybrid systems contribute to a more sustainable power generation model, balancing efficiency with environmental impact.
5. Future of Hybrid and Combined-Cycle Innovations
The future of hybrid and combined-cycle systems is promising, with emerging technologies and digital tools paving the way for even greater efficiency and environmental benefits.
Carbon Capture Integration in Combined Cycles:
- Innovations in carbon capture and storage (CCS) technologies are now being integrated into combined-cycle plants, further reducing greenhouse gas emissions. By capturing CO₂ emissions from the exhaust, combined-cycle plants can achieve near-zero emissions, making natural gas plants far more environmentally friendly.
Digital Integration and Real-Time Optimization:
- Digital tools, including AI and IoT, are revolutionizing hybrid and combined-cycle systems. Real-time monitoring and predictive analytics allow operators to optimize performance, adjusting fuel use and power output to match demand and maximize efficiency. Digital twins—virtual models of physical systems—allow for virtual testing and scenario planning, improving plant reliability and efficiency.
Sustainable Power Generation Prospects:
- As energy demands grow and environmental regulations tighten, hybrid and combined-cycle systems are expected to play a central role in sustainable power generation. These technologies offer an adaptable, efficient approach that meets both immediate energy needs and long-term environmental goals.
With ongoing advancements, hybrid and combined-cycle technologies continue to evolve, supporting a transition toward greener, more efficient energy production.
1. Introduction to Turbines in Power Generation
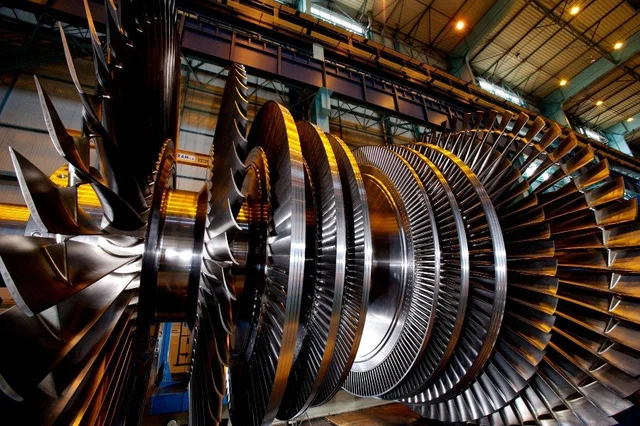
Turbines are the backbone of power generation, serving as the primary devices for converting thermal, kinetic, or potential energy into mechanical power. This mechanical energy is then transformed into electricity via generators, making turbines essential in various power plant settings, from traditional fossil fuel plants to renewable energy sources like wind and hydroelectric facilities. Each type of power plant utilizes turbines in a unique way, tailored to the energy source and specific operational requirements.
In fossil fuel and nuclear plants, turbines operate through the expansion of high-pressure steam or combustion gases, which drive the turbine blades. Hydroelectric turbines use the potential energy of water, while wind and geothermal turbines capture energy from natural forces to generate power. Turbine efficiency directly impacts a plant’s overall performance, influencing fuel consumption, emissions, and operational costs. As technological advancements enhance turbine efficiency, power plants are able to produce electricity more sustainably, supporting global efforts to transition toward cleaner energy. Understanding the role and operational dynamics of turbines in different power plants offers insight into how these devices support a reliable and resilient energy infrastructure.
2. Turbines in Fossil Fuel Power Plants
In fossil fuel power plants, turbines are central to the energy conversion process, transforming thermal energy from coal, oil, or natural gas combustion into electricity. These plants use steam turbines in coal and oil-fired power plants and gas turbines in natural gas-fired plants, each with mechanisms tailored to their fuel source.
Mechanisms of Energy Conversion in Coal, Oil, and Gas Power Plants:
- In coal and oil power plants, fuel is burned in a boiler to produce high-pressure steam. This steam is directed through the blades of a steam turbine, causing them to rotate. The rotation drives a generator, which converts the mechanical energy into electricity.
- In gas-fired power plants, the fuel combustion process produces high-temperature, high-pressure gases that are directed through a gas turbine. The turbine’s blades are designed to harness the energy from the expanding gases, spinning the turbine and generating electricity.
Role of Steam and Gas Turbines in Energy Production:
- Steam Turbines: Steam turbines play a major role in coal and oil plants, where high-pressure steam created in boilers is used to produce electricity. Modern steam turbines often include multiple stages to capture energy at different pressures, improving efficiency.
- Gas Turbines: Gas turbines are used in natural gas power plants, which are often chosen for their quick start-up times and ability to adjust to demand changes. In combined-cycle plants, the exhaust from gas turbines is used to generate additional steam, which drives a secondary steam turbine, maximizing energy capture.
Efficiency Improvements and Emissions Reduction:
- Turbine efficiency improvements in fossil fuel plants are crucial for reducing fuel consumption and minimizing greenhouse gas emissions. By incorporating advanced materials, optimized blade design, and heat recovery systems, modern turbines can convert more thermal energy into electricity with minimal losses.
- Combined-cycle plants are particularly efficient, achieving efficiencies above 60% by utilizing waste heat from the gas turbine. This approach reduces emissions per unit of electricity generated, supporting cleaner energy production from fossil fuels.
The role of turbines in fossil fuel power plants highlights the importance of efficiency in reducing environmental impact while meeting energy demand. Advances in turbine technology continue to make fossil fuel plants more sustainable by enhancing performance and lowering emissions.
3. Turbines in Nuclear Power Plants
In nuclear power plants, turbines are essential for converting the thermal energy produced by nuclear fission into electricity. Nuclear plants use steam turbines in a process similar to that of coal and oil plants, but the heat source is nuclear fuel rather than combustion.
Steam Turbines and the Rankine Cycle:
- Nuclear power plants typically operate using the Rankine cycle, where water is heated to create steam that drives a steam turbine. In this cycle, nuclear reactors replace conventional boilers as the heat source, using controlled nuclear fission reactions to heat water and produce steam.
- The high-pressure steam generated in the reactor flows through multiple stages in the steam turbine, gradually losing pressure as it transfers energy to the turbine blades. This energy is then converted into electricity via a generator.
Heat Generation Through Nuclear Fission:
- In a nuclear reactor, fission reactions produce intense heat, which is transferred to a coolant (usually water) that circulates through the reactor core. This heated coolant transfers thermal energy to a separate water source in a steam generator, creating the steam required to drive the turbine.
- The controlled environment of nuclear fission provides a consistent heat source, making nuclear power plants ideal for base-load electricity generation, as they can operate continuously with minimal interruptions.
Safety Considerations and Efficiency Challenges:
- Nuclear plants have rigorous safety requirements due to the nature of radioactive materials. Turbines and other components must be designed to handle potential emergency shutdowns, rapid temperature changes, and high-pressure steam without failure.
- While steam turbines in nuclear plants are similar to those in coal plants, efficiency improvements are limited by the need for stable, lower-temperature operation to ensure safety. However, advancements in turbine materials and design continue to improve efficiency, reducing operational costs and enhancing nuclear energy’s competitiveness.
The role of turbines in nuclear power plants demonstrates the critical function of these devices in ensuring the safe and continuous production of electricity, supporting reliable, low-carbon energy.
4. Turbines in Hydroelectric Power Plants
Hydroelectric power plants utilize turbines to convert the potential and kinetic energy of water into electricity, making turbines essential for clean, renewable energy production. Unlike thermal plants, hydroelectric plants rely on gravity and water flow, which powers turbines as water is released from reservoirs or flows naturally in rivers.
Role of Hydroelectric Turbines in Energy Conversion:
- In a typical hydroelectric plant, water flows from a high elevation to a lower elevation through a penstock, gaining speed and pressure. This high-speed water strikes the turbine blades, causing them to rotate and drive a generator, producing electricity.
- The energy conversion process is highly efficient, as water can be controlled and directed with minimal energy loss. This is why hydroelectric turbines can reach efficiencies of up to 90%.
Types of Turbines Used in Hydroelectric Power Plants:
- Pelton Turbines: Pelton turbines are impulse turbines that work well in high-head, low-flow conditions. Water is directed through nozzles that convert pressure into high-speed jets, striking the turbine buckets and driving rotation.
- Francis Turbines: Francis turbines are reaction turbines designed for medium to high-head applications. Water flows radially into the turbine, turning the blades as it moves through, ideal for large-scale hydroelectric dams.
- Kaplan Turbines: Kaplan turbines are axial-flow reaction turbines suited for low-head, high-flow conditions. The blades can adjust their pitch to optimize performance, which is advantageous in fluctuating water flow conditions.
Environmental Considerations and Efficiency Factors:
- Hydroelectric turbines offer a sustainable source of energy but can impact local ecosystems, fish migration, and water quality. Turbine designs now include fish-friendly modifications and control systems to minimize environmental disruption.
- Efficiency in hydroelectric turbines is largely dependent on water flow rates and pressure, with maintenance practices focusing on preventing erosion and cavitation damage to blades.
Turbines in hydroelectric plants are a prime example of how renewable energy can be harnessed sustainably, supporting clean energy production with minimal environmental impact when managed effectively.
5. Turbines in Renewable Energy Plants (Wind and Geothermal)
In renewable energy plants, turbines are essential for harnessing natural forces like wind and geothermal heat, allowing for sustainable electricity production without fossil fuels. These plants rely on turbine efficiency and advanced technology to maximize energy capture from renewable sources.
Wind Turbines and Aerodynamic Principles:
- Wind turbines convert the kinetic energy of moving air into mechanical energy. Their blades are designed with an aerodynamic shape to maximize lift and minimize drag, capturing energy as wind flows over the blades and causing rotation.
- Wind turbines typically feature adjustable blade angles (pitch control) to optimize performance based on wind speed. Onshore and offshore wind farms utilize these turbines to provide large-scale renewable energy.
Geothermal Turbines and Steam Extraction:
- Geothermal power plants use steam turbines to harness heat from beneath the Earth’s surface. Geothermal reservoirs provide a constant steam source, which drives the turbine similarly to a traditional steam turbine.
- By extracting naturally occurring steam, geothermal turbines provide consistent, renewable base-load power. Efficiency in geothermal turbines depends on temperature and steam pressure, with advanced materials used to withstand the corrosive nature of geothermal fluids.
Turbines in wind and geothermal plants highlight the versatility of turbine technology in renewable energy production, supporting a clean energy transition with sustainable, low-emission power generation.
6. Technological Advancements and Future Trends
The role of turbines in power plants continues to evolve, with innovations aimed at improving efficiency, flexibility, and environmental impact. Hybrid systems and combined-cycle technology are making power generation more efficient by integrating gas and steam turbines or combining renewable sources with conventional energy.
Hybrid and Combined-Cycle Innovations:
- Combined-cycle plants use both gas and steam turbines, capturing waste heat to generate additional power. These plants achieve efficiencies above 60%, providing a cleaner alternative to traditional fossil fuel plants.
- Hybrid systems combine renewable energy sources, such as solar or wind, with battery storage and gas turbines. This combination offers flexible, reliable power generation with lower emissions.
Digital Integration and Predictive Maintenance:
- Digital tools like IoT sensors and machine learning improve turbine efficiency by enabling real-time monitoring and predictive maintenance. Digital twins and AI allow operators to simulate and optimize turbine performance under different conditions, reducing downtime and operational costs.
As technology advances, turbines will play an even more central role in the global transition to sustainable energy, supporting cleaner, more efficient power generation across various energy sectors.
1. Overview of Turbine Function and Energy Conversion
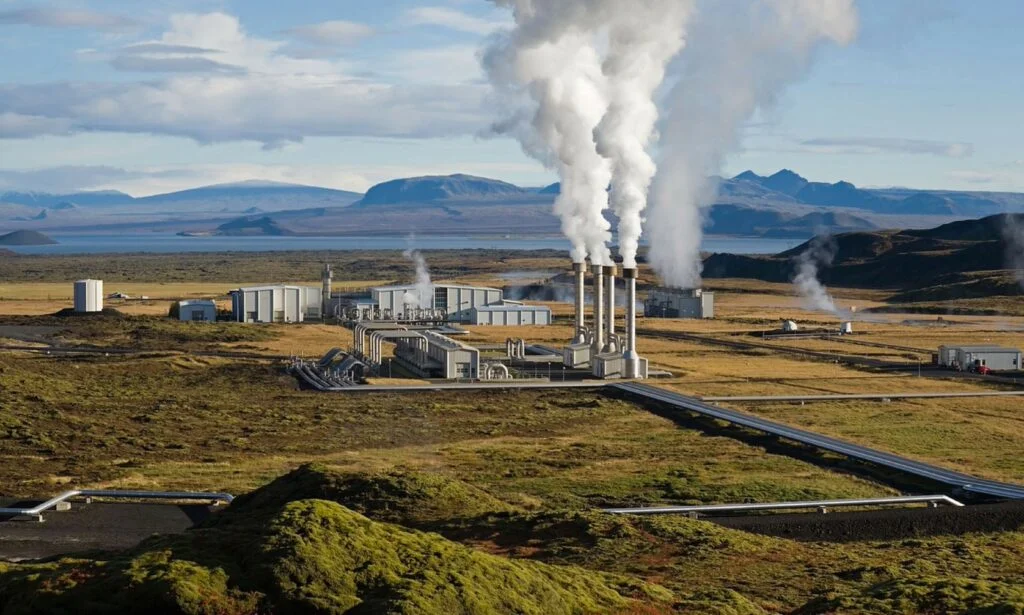
Turbines are crucial to power generation, functioning as the primary devices that convert various forms of energy—thermal, kinetic, and potential—into mechanical energy. This mechanical energy is subsequently transformed into electrical energy by a generator, making turbines the backbone of nearly all modern power plants. By harnessing energy from fuels like coal, oil, natural gas, and renewable sources such as wind, water, and geothermal heat, turbines enable large-scale electricity production that powers homes, industries, and infrastructure.
The basic operating principle of a turbine is simple: as fluid or gas flows over the turbine blades, it imparts kinetic energy that causes the blades to spin. This spinning motion drives a rotor connected to a generator, producing electricity. The design and operation of a turbine vary widely depending on the energy source it harnesses, which has led to the development of different types of turbines tailored to specific power generation needs.
The primary turbine types include steam turbines, which operate in coal, oil, and nuclear plants; gas turbines, used in natural gas and combined-cycle plants; hydroelectric turbines, which rely on water flow; and wind turbines, which capture energy from wind currents. Each type operates under unique conditions and with distinct mechanisms, but all share the common purpose of converting energy into electricity as efficiently as possible. As global energy demand continues to rise and sustainability becomes a top priority, optimizing turbine performance and efficiency is essential in advancing cleaner and more efficient power generation technologies.
2. Types of Turbines and Their Operating Principles
Steam Turbines:
- Steam turbines are commonly used in fossil fuel and nuclear power plants, where they convert thermal energy into mechanical energy by harnessing high-pressure steam. In a typical steam turbine system, water is heated in a boiler, creating steam that reaches high pressures. This pressurized steam flows over the turbine blades, causing them to rotate and generate power. The Rankine cycle describes this process, in which water is repeatedly heated, vaporized, and condensed in a closed-loop system, optimizing energy use.
- Steam turbines are highly efficient and often use multiple stages to capture energy from steam at varying pressures. In nuclear power plants, steam turbines play a critical role by converting the heat generated from nuclear fission into electricity, providing a stable, low-carbon source of base-load power.
Gas Turbines:
- Gas turbines are widely used in natural gas-fired power plants and are integral to peak-load power generation. These turbines operate on the Brayton cycle, where compressed air is mixed with fuel and ignited in a combustion chamber. The resulting high-temperature gases rapidly expand and flow over the turbine blades, producing rotational energy. Gas turbines are particularly valued for their quick start-up times, making them ideal for applications that require rapid response to changes in demand.
- In combined-cycle power plants, gas turbines work alongside steam turbines, where waste heat from the gas turbine is used to produce steam, which drives a secondary steam turbine. This integration maximizes energy extraction from the fuel, significantly improving plant efficiency and reducing emissions.
Hydroelectric Turbines:
- Hydroelectric turbines convert the potential energy of water into electricity, making them central to renewable power generation. These turbines operate in dams or run-of-river installations, where flowing water is channeled through penstocks to drive the turbine blades. As the water flows over the blades, it causes them to spin, generating mechanical energy that powers an electric generator.
- There are several types of hydroelectric turbines designed to optimize energy capture under different flow conditions. Pelton turbines are impulse turbines suited for high-head, low-flow environments, while Francis and Kaplan turbines are reaction turbines that work efficiently in medium- to low-head, high-flow settings. Hydroelectric turbines offer one of the most efficient means of power generation, with energy conversion efficiencies reaching up to 90%.
Wind Turbines:
- Wind turbines capture the kinetic energy of moving air and convert it into electrical power, making them a vital component of modern renewable energy. Wind turbine blades are designed with aerodynamic profiles to maximize lift and minimize drag, capturing wind energy as they spin. The rotor’s motion drives a generator, producing electricity.
- Wind turbines are available in two main designs: horizontal-axis and vertical-axis turbines. Horizontal-axis turbines, the most common type, use long, slender blades and are typically deployed in large onshore and offshore wind farms. Vertical-axis turbines are less common but are sometimes used in urban settings due to their compact design and ability to capture wind from any direction.
- Wind turbines rely heavily on location, wind speed, and wind consistency, which influence their efficiency and power output. Advances in materials, blade design, and control technologies have made wind turbines more efficient, supporting the growth of wind energy as a major source of clean power.
Each type of turbine is designed to meet specific energy demands and operating conditions, making turbines versatile devices that power a wide array of energy systems across the globe.
3. Importance of Turbine Efficiency and Technological Advancements
Turbine efficiency plays a crucial role in power generation, impacting fuel consumption, operational costs, and emissions. Higher efficiency means that more energy is converted into electricity for every unit of fuel, reducing waste and enhancing sustainability. Technological advancements in turbine design, materials, and operational control have dramatically improved turbine efficiency, supporting more sustainable power generation.
Fuel Use and Operational Costs:
- In traditional power plants, higher turbine efficiency reduces the amount of fuel required to produce a given amount of electricity, directly impacting fuel costs. By optimizing turbine performance, power plants can meet energy demands with less fuel, minimizing costs and reducing dependence on fossil fuels.
Emissions Reduction:
- Efficiency improvements have a direct effect on emissions. With less fuel consumption, plants generate fewer greenhouse gases and pollutants, supporting environmental goals and compliance with emissions regulations. For example, combined-cycle gas turbines achieve efficiencies above 60%, reducing carbon emissions significantly compared to single-cycle systems.
Technological Innovations:
- Advances in high-temperature materials, such as nickel-based superalloys and ceramic composites, allow gas turbines to operate at higher temperatures, improving efficiency. Thermal barrier coatings protect turbine blades from heat damage, enabling safe operation at increased combustion temperatures.
- Aerodynamic blade designs and computational fluid dynamics (CFD) simulations help optimize blade shape, angle, and surface textures, reducing drag and increasing lift. These improvements ensure more efficient fluid flow across the turbine blades, enhancing energy capture.
- Digital integration, including IoT sensors and predictive maintenance algorithms, enables real-time monitoring of turbine performance. Predictive maintenance reduces unexpected downtime, lowers maintenance costs, and ensures that turbines operate at peak efficiency.
Through these advancements, modern turbines operate more efficiently, cost-effectively, and sustainably, making them essential for meeting today’s energy and environmental challenges.
4. Future Trends and Sustainability in Turbine Technology
The future of turbine technology will be shaped by advancements in hybrid and combined-cycle systems, digital integration, and a strong focus on sustainability. As the energy industry transitions toward cleaner energy sources, turbines are expected to play an even more significant role in supporting renewable and low-carbon energy production.
Hybrid and Combined-Cycle Systems:
- Hybrid systems that integrate renewable sources with conventional turbines are gaining traction. For instance, combining solar-thermal energy with natural gas turbines allows power plants to reduce fossil fuel use while providing reliable energy. Combined-cycle plants, which use both gas and steam turbines, achieve higher efficiencies and lower emissions, making them ideal for reducing the environmental impact of natural gas power generation.
- These systems maximize energy capture and reduce waste by utilizing multiple sources or stages of energy extraction, aligning with sustainability goals and supporting a more resilient energy grid.
Digital Integration and Predictive Maintenance:
Digital technologies, such as IoT and machine learning, are transforming turbine operation. Real-time data collection and analysis
EMS Power Machines
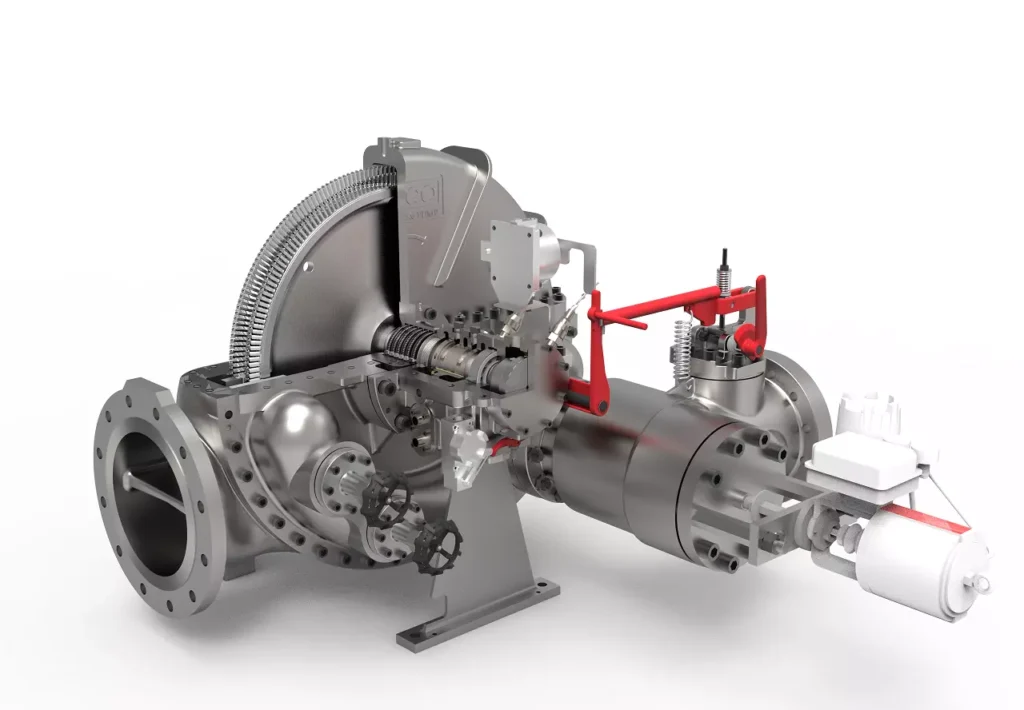
We design, manufacture and assembly Power Machines such as – diesel generators, electric motors, vibration motors, pumps, steam engines and steam turbines
EMS Power Machines is a global power engineering company, one of the five world leaders in the industry in terms of installed equipment. The companies included in the company have been operating in the energy market for more than 60 years.
EMS Power Machines manufactures steam turbines, gas turbines, hydroelectric turbines, generators, and other power equipment for thermal, nuclear, and hydroelectric power plants, as well as for various industries, transport, and marine energy.
EMS Power Machines is a major player in the global power industry, and its equipment is used in power plants all over the world. The company has a strong track record of innovation, and it is constantly developing new and improved technologies.
Here are some examples of Power Machines’ products and services:
- Steam turbines for thermal and nuclear power plants
- Gas turbines for combined cycle power plants and industrial applications
- Hydroelectric turbines for hydroelectric power plants
- Generators for all types of power plants
- Boilers for thermal power plants
- Condensers for thermal power plants
- Reheaters for thermal power plants
- Air preheaters for thermal power plants
- Feedwater pumps for thermal power plants
- Control systems for power plants
- Maintenance and repair services for power plants
EMS Power Machines is committed to providing its customers with high-quality products and services. The company has a strong reputation for reliability and innovation. Power Machines is a leading provider of power equipment and services, and it plays a vital role in the global power industry.
EMS Power Machines, which began in 1961 as a small factory of electric motors, has become a leading global supplier of electronic products for different segments. The search for excellence has resulted in the diversification of the business, adding to the electric motors products which provide from power generation to more efficient means of use.